Geroscience in heart failure: the search for therapeutic targets in the shared pathobiology of human aging and heart failure
Abstract
Age is a major risk factor for heart failure, but one that has been historically viewed as non-modifiable. Emerging evidence suggests that the biology of aging is malleable, and can potentially be intervened upon to treat age-associated chronic diseases, such as heart failure. While aging biology represents a new frontier for therapeutic target discovery in heart failure, the challenges of translating Geroscience research to the clinic are multifold. In this review, we propose a strategy that prioritizes initial target discovery in human biology. We review the rationale for starting with human omics, which has generated important insights into the shared (patho)biology of human aging and heart failure. We then discuss how this knowledge can be leveraged to identify the mechanisms of aging biology most relevant to heart failure. Lastly, we provide examples of how this human-first Geroscience approach, when paired with rigorous functional assessments in preclinical models, is leading to early-stage clinical development of gerotherapeutic approaches for heart failure.
Keywords
INTRODUCTION
Aging in heart failure epidemiology
Heart failure (HF) is a clinical syndrome defined by symptoms or signs of pulmonary or systemic congestion caused by a structural and/or functional cardiac abnormality[1]. Approximately 64 million people worldwide are currently living with HF, which has more than doubled in the past two decades[2,3]. While multiple factors, including rising rates of cardiometabolic disease (i.e., obesity, diabetes), are contributing to the increasing prevalence of HF, a major impetus for the rising number of HF cases is the progressive aging of the world’s population. Currently, ~14% of the world’s population is > 60 years old; by 2050, this figure is anticipated to reach 22%, representing more than 2 billion older adults worldwide (https://who.int/news-room/fact-sheets/detail/ageing-and-health).
Similar to its role in most chronic diseases, age is a major risk factor for HF. The incidence of HF is over
Aging biology: a new target for therapeutic development in heart failure?
The search for therapeutic targets for HF has evolved with our understanding of its pathophysiology. The recognition that chronic activation of compensatory neurohormonal pathways (i.e., adrenergic nervous system, renin-angiotensin-aldosterone [RAAS] system) contributes to maladaptive myocardial remodeling and HF progression revolutionized drug development for HFrEF from the 1990-2000s[6]. In the past two decades, insights into the mechanistic underpinnings of various subtypes of HF, including cardiac amyloidosis and hypertrophic cardiomyopathy, have led to the recent approval of targeted therapeutics (e.g., tafamidis, mavacamten) for these specific forms of HF[7,8]. More recently, sodium-glucose cotransporter 2 (SGLT2) inhibitors and glucagon-like peptide 1 (GLP1) agonists have emerged as effective therapies for both HFrEF and HFpEF[9-11]. While the mechanisms mediating their benefits in HF are multifactorial and not yet fully understood, their effects on metabolic remodeling have invigorated efforts to target this pathobiology in HF.
Conversely, age has long been a thorn in HF management. Conventional thinking construes age as an important risk factor to recognize and consider in the management of older adults with HF, but one that cannot be modified. This concept, however, is changing with the rapidly evolving field of Geroscience[12-14]. Geroscience is the study of the biology of aging that prioritizes human translation. It is based on a central hypothesis that aging is a primary cause of most chronic diseases in older adults, and thus, attenuating or reversing biological processes that govern aging could simultaneously delay or treat many of these diseases, including HF[12,15]. This hypothesis is, of course, dependent not only on aging being truly malleable, but also on the establishment of shared causal biology between aging and disease. If these two criteria are met, then simply put, age becomes more than just a risk factor, but one that can be targeted for therapeutic development.
While the field of Geroscience is relatively new, the hypothesis that cardiovascular aging is part of the causal pathophysiology of HF has been around since at least the 1940s[16]. Traditional risk factors (e.g., hypertension, diabetes, coronary artery disease, etc.) account for only ~53% of the population attributable risk for HF in the elderly[17], suggesting that intrinsic elements of aging may be unrecognized drivers of HF in this population. Indeed, the aging cardiovascular system exhibits numerous parallels to HF, from the physiological to the molecular level [Figure 1]. Elegant physiology studies in healthy humans, across the spectrum of age, have shown that normal aging is associated with progressive impairments in subclinical systolic and diastolic function, cardiac reserves, peripheral oxygen extraction, and overall exercise capacity, along with changes in macro- and microvascular function[18-21]. At a cellular and molecular level, aging is associated with cardiomyocyte hypertrophy, impaired Ca2+ cycling, mitochondrial dysfunction, altered proteostasis, dysregulated metabolism, loss of regenerative potential, in addition to endothelial dysfunction and increased fibrosis. A detailed discussion of these changes is beyond the scope of this paper, but they have been extensively reviewed in recent publications[13,14,21-23]. Notably, while cardiovascular aging, in itself, does not equate to clinical HF, it results in a substrate in older adults that can easily transition into the disease manifestation of HF. The question is no longer whether the aged cardiovascular system is more susceptible to HF, but more so, what biological mechanisms of aging drive this increased HF vulnerability in older adults.
Figure 1. Schematic of the physiological, structural, and molecular changes that occur with cardiovascular aging. This illustration was partly generated using images adapted from BioRender and Servier Medical Art (https://smart.servier.com). Servier Medical Art is provided by Servier, licensed under a Creative Commons Attribution 4.0 license (https://creativecommons.org/licenses/by/4.0/).
At a fundamental level, the parallels between aging and HF likely stem from similar core biology [Figure 2]. Evolutionary theories of aging, including the theory of antagonistic pleiotropy[24] and the disposable soma theory[25], propose that while evolution favors traits that maximize early survival, growth, and reproduction, these same traits can have deleterious effects in late life. Additionally, as mutations, DNA damage, and oxidative stress accumulate in the soma over time, resource utilization shifts toward pathways to maintain its survival, often at the expense of function. The “healthy” aged heart is no exception. Processes critical for optimal cardiac function, such as fatty acid metabolism, Ca2+ cycling (e.g., SERCA2a), and β-adrenergic sensitivity, are all downregulated in the aged heart[21,23], while survival pathways, such as senescence and Akt signaling, are activated. As these cell survival pathways become chronically activated, they become maladaptive and contribute to adverse remodeling and functional impairments in the aged heart[26-28], similar to the core pathophysiology underlying HF[6,29]. Ultimately, the unintended consequences of these early life selection forces and the compensatory adaptations that occur later in life contribute not only to the progressive functional decline associated with organismal aging, but also to the development of chronic diseases, such as HF.
Figure 2. Similar fundamental biology underlying aging and heart failure. The antagonistic pleiotropy theory of aging proposes that evolution strongly selects for traits that maximize early-life survival, growth, and reproduction. However, these same traits can be deleterious in late life and lead to aging and chronic diseases, such as heart failure. Classic examples of this are senescence and IGF1 signaling, which enhance survival and growth in early life, but contribute to adverse cardiac remodeling and dysfunction in late life. The modified disposable soma theory of aging proposes that in early life, organisms will prioritize resources for biological processes that maximize early mid-life survival/growth/function/reproduction (at the expense of maintaining the soma), ultimately leading to accumulating DNA damage and aging. In later life, organisms shift priorities and resource utilization to maintain the soma, even at the expense of function. However, chronic activation of these survival pathways (e.g., senescence, Akt signaling) can become maladaptive, contributing to further adverse remodeling and functional decline in the aging heart. This illustration was partly generated using images adapted from BioRender and Servier Medical Art (https://smart.servier.com). Servier Medical Art is provided by Servier, licensed under a Creative Commons Attribution 4.0 license (https://creativecommons.org/licenses/by/4.0/).
Evidence supporting this conceptual framework in both aging and HF is seen in the hallmarks of aging[12]. A quintessential example is senescence, which is a process of irreversible cell cycle arrest that promotes cell survival in response to injury and stress. While senescence protects from cancer and enhances survival/reproductive potential in early-mid life, its persistence in late life contributes to the inflammation and multi-organ system failure, including HF, that occurs with aging[28,30]. Anabolic insulin-like growth factor 1 (IGF1) signaling, the first longevity pathway discovered[31,32], provides additional evidence of how aging and HF pathophysiology follow the principles of antagonistic pleiotropy. In humans, higher circulating levels of IGF1 are associated with lower disease risk in youth, but correlate with increased risk for incident disease and death in older adults[33]. Indeed, across species, reduced IGF1 receptor (IGF1R) signaling enhances longevity[34]. In HF, although early studies found that circulating IGF1 was inversely associated with HF risk in older adults[35], more recent work has elegantly shown that when IGF1R signaling is directly modulated in the heart, it displays a more similar pattern to aging, in which IGF1R activation improves cardiac function in young animals, but promotes heart failure in older ones[36,37]. Ultimately, in accordance with the Geroscience hypothesis, understanding this shared (patho)biology between aging and HF could identify novel therapeutic targets for both.
A human-first approach to translating aging research in heart failure
The integration of Geroscience into cardiovascular research[14,38], combined with the rapidly growing number of interventions that can potentially “reverse” aging, has led to an explosion of biological aging mechanisms with therapeutic implications in HF[12,22]. However, it is important to recognize that the success rate of a drug target identified in a preclinical study reaching phase II/III clinic trials is extremely low, especially for cardiovascular diseases[39,40]. The reasons for this are multifold[40], involving the high costs of funding large-scale clinical trials and prohibitive safety issues with many experimental therapeutics, as well as intrinsic differences in the biology governing aging and HF between humans and experimental animal models. For example, evolutionary selection pressures likely differ between short- versus long-lived species; thus, genetic drivers of lifespan and healthspan in a worm that lives for 2-3 weeks may be fundamentally different than those for humans. Similarly, most preclinical models of HF use acute cardiac injury or stress in young animals, which may not fully reflect the chronic pathophysiology of human HF or the relevance to aging in older adults with HF[41].
While numerous strategies have been proposed to overcome the “valley of death” between preclinical research and clinical drug development, a common theme has been an increasing emphasis on human biology in initial target discovery[40,42,43]. Over the past few decades, remarkable advancements in -omics technology (e.g., genomics, transcriptomics, proteomics, metabolomics) have enabled this human-first approach, providing insights into the shared biology of human aging and HF. Notably, while this approach has revolutionized drug development in cancer, the same has not been true in aging or HF. This is not only due to their complex polygenic nature, but also the heterogeneous systemic (patho)physiology of aging and HF that often requires rigorous assessment in animal models before making the leap to patients[41,44].
Here, we start by reviewing recent -omics studies in human aging and HF to demonstrate how this human-first approach can be used to (1) gain insights into the shared biology between aging and HF; and (2) prioritize those mechanisms of aging biology with greater potential for therapeutic development in HF. The breadth of omics data in aging and HF is beyond the scope of any single review. Here, we focus on genomics and proteomics as a starting point. We discuss how genomics can identify causal genetic determinants underlying the strong association between aging and HF, while proteomics conveys a broader picture of their common (mal)adaptative pathophysiology. We conclude each section by providing a detailed discussion of some of the top candidates from human-based omics discovery, which highlights how this Geroscience approach can lead to effective translation of therapeutics targeting aging biology in HF.
GENOMICS
Genome-wide association studies in human aging and heart failure
Genomics is the comprehensive study of the entire genome, including the interaction between genes, their regulation, and their downstream effects in the organism. Monogenic causes of premature aging (e.g., progeria) or cardiomyopathy have provided important, albeit relatively specific, insights into the biology of aging and HF. Given the heterogeneity of these complex traits, more recent efforts have utilized genome-wide association studies (GWAS) to better understand their polygenic underpinnings. GWAS involve the genotyping of large populations, usually on the scale of 104-106 individuals, in which statistical associations between millions of germline variants and pre-defined traits of interest (e.g., longevity) are tested. Methods, such as quantitative trait locus (QTL) analysis, colocalization, and Mendelian randomization (MR), are then utilized to assess putative functional effects or infer causality of candidate variants.
Extreme age cutoffs, parental lifespan, or healthspan have been used to define the longevity trait in genomics. Heritability of human longevity is estimated at ~16% or less[45], and to date, GWAS have identified > 100 loci associated with human longevity [Table 1][46-51]. Interestingly, offspring of longer-lived parents have ~14% lower risk of developing HF and lower genetic risk scores for HF risk factors[52], suggesting there may be common genetic drivers in aging and HF. Similar to longevity, the heterogeneity of HF has made GWAS challenging. However, collaborative efforts across multiple consortiums are leading to the identification of multiple loci associated with HF or its endophenotypes (e.g., HF risk factors, quantitative imaging traits, etc.) [Table 1][53-57].
Genome-wide association studies in human aging and heart failure. This table summarizes the variants that are associated with aging traits (longevity, parental lifespan, healthspan) or incident HF (and HF endophenotypes). Bold indicates gene loci associated with both aging and HF traits
Study | Population | Size | Associated genes |
Longevity | |||
Deelen et al., 2014[46] | Meta-analysis of 14 European ancestry studies | 44,368 | MTR, RYR2, CSRNP3, PDE5A, MAD2L1, EBF1, EYA1, CYP2C19, TANC2, APOE, TSH22, ZNF217, NTN1, MARCH10, TANC2 |
Broer et al., 2015[47] | Cohorts for heart and aging research in genomic epidemiology (CHARGE) | 9,793 | GRIK2, CADM2, RGS7, SOX6, MBOAT1, PFKM, LIMCH1, FOXO3 |
Yashin et al., 2015[48] | Framingham heart study | 1,529 | TLK2, C1QTNF5, ECHS1, RIMBP2, CYP51A1, WRN, NRDE2 |
Zeng et al., 2016[49] | Chinese longitudinal healthy longevity study (CLHLS) | 4,477 | IL6, ANKRD20A9P, MIR3156-3, AKR1C2, FAM13A, BEND4, EPHA6, ZFYVE28, ASIC2, OLFM4, APOE |
Gurinovich et al., 2021[50] | New England centenarian study; LonGenity | 5,169 | APOE, SHB, ALDH1B1, FBX015, LRRC3B, APBA2, CCNC, PRDM13, MYYO5B |
Deelen et al., 2019[51] | Meta analyses of 23 studies (18 European, 1 East Asian, 1 African American) | 142,203 | GRP78, RP1, RDH5, APOE, KALRN, K1F13B, CDKN2A/B |
Parental lifespan | |||
Pilling et al., 2017[227] | UK biobank | 389,166 | CELSR2, HLA-DRB1, LPA, CDNK2B-AS1, ATXN2, CHRNA3/4, FURIN, APOE, BEND3, EPHX2, PROX2, MC2R, TNNI3K, USP2-AS1, PSORS1C3, B3GALT3L, MICA/B, TOX, ZW10, SEMA6D, EGLN2, CYP2A6, EXOC3L2, MARK4, C20orf187 |
Joshi et al., 2017[228] | CHARGE | 606,059 | HLA-DQA1/DRB1, LPA, CHRNA3/5, APOE |
Wright et al., 2019[229] | AncestryDNA | > 300,000 | CDKN2B-AS1, WAPL, LPA, SRRM3, CHRNA3/5, APOE |
Timmers et al., 2019[230] | UK biobank, LifeGen | 1,012,240 | MAGI3, KCNK3, HTT, HLA-DQA1, LPA, CDKN2B-AS1, ATXN2/BRAP, CHRNA3/5, FURIN, HP, LDL3, APOE |
Healthspan + parental lifespan + longevity | |||
Timmers et al., 2020[231] | UKBiobank | 1,349,462 | CELSR2, MAGI3, KCNK3, CASP8, SLC4A7, HTT, LINC02513, DUSP22, HLA-DRB1, FOXO3, LPA, CDKN2B-AS1, TCF7L2, TYR, ZW10, FGD6, ATXN2, CHRNA3, FURIN, TOX3, DEF8, LDLR, APOE, NOL4L |
Heart failure | |||
Smith et al., 2010[53] | CHARGE | 23,821 | USP3, LRIG3, BCHE, EVX1, SNX16, MOBKL2B, CH25H, PRICKLE1, TBC1D4, GNA15, SH3GL2, RPUSD4, TMTC1, BTG1, HLX-AS1, TEX51 |
Shah et al., 2020[54] | Heart failure molecular epidemiology for therapeutic targets (HERMES) consortium | 977,323 | CELSR2, PITX2, FAM241A, KLHL3, CDKN1A, LPA, CDKN2B-AS1, ABO, SURF1, SYNPO2L, AGAP5, BAG3, ATXN2, FTO |
Levin et al., 2022[55] | HERMES, eMERGE, Penn Med Biobank, Mt Sinai BioME, geisinger DiscovEHR, FinnGen, global biobank | 1,665,481 | RP11, NPC1, ZFHX3, CDKN2B, PITX2, HDGFL1, FTO, CDKN1A, SURF6, SYNPO2L, CLCNKA, IRAK1BP1, FAM133B, STRN, BAZ1A, SRR, GNPDA2, PMAIP1, TMEM18, GTF2I, HSD17B12, BAG3, HECTD4, LPA, SH2B3, DMRTA2, USP36, OR2A2, POM121C, CACNB2, ASXL3, CHMP3, TTC39A, NFIA, KCNIP4, TUBA3C, ORC5, ZNF280A, COX7C |
Joseph et al., 2022[56] | Million veteran program, UK biobank | 529,386 | SPARP, HSPB7, ZBTB17, CELSR2, E2F6, ABHD5, PITX2, CDKN1A, LPA, GTF2I, CDKN2B-AS, ABO, CAMK2G, BAG3, FTO, NFAT5, SMG6, PNMT, PGAP3, YPEL2, BPTF, MAP3K7CL |
Rasooly et al., 2023[57] | HERMES, million veteran program | 1,279,610 | HSD17B12, HDGFL1, SPATS2L, TMEM18, SCARB1, CAMK2D, FAF1, GNPDA2, POM121C, ZEB2, RIC8B, FANCL, PRKD1, SLC39A8, HEATR5B, PHIP, SYMPK, SGIP1, CDKN2B, PITX2, FTO, LPA, CDKN1A, PSRC1, C1orf64, BAG3, ABO, SYYNPO2L, BPTF, KLHL3, BACH1, GTF21, NFAT5, SMG6, ALDH2, MIA3, PNMT, PHACTR1 |
The overlap in genetic variants associated with both longevity and HF is not extensive. However, two shared between these complex traits are notably loci related to senescence, including CDKN2A/B (p14/p15/p16/ARF cluster) and the regulatory non-coding RNA CDKN2B-AS1 (also known as ANRIL). While variants near the CDKN1A gene (which encodes the senescence protein p21) have not reached genome-wide significance in human longevity studies, they have repeatedly emerged in HF GWAS with MR analysis inferring causality in HF and LVEF endophenotypes[54,56,57]. As previously alluded to, senescence represents a quintessential example of how evolutionary theories explain aging [Figure 2]. The association of these loci in both human longevity and HF points to senescence as a potential causal process of aging biology in HF, which will be further discussed in the upcoming sections.
Somatic mutations in human aging and heart failure
While GWAS identify associations between germline variants (i.e., what we are born with) and traits of interest, most of the body’s cells (collectively referred to as the soma) will also accumulate DNA mutations throughout their lifetime. Most somatic mutations have little or no effect on the cell, but their aggregate accumulation increases the risk of deleterious functional mutations, which is the premise of the classical DNA damage theory of aging[58]. Recent progress in next-generation sequencing has led to exciting new insights into the genomic mosaicism that occurs with aging, supporting a functional role of somatic mutations in chronic disease pathogenesis[59].
Our knowledge of how age-associated somatic mutations contribute to HF pathobiology is rapidly evolving. Elegant work by Choudhury et al. recently showed that human cardiomyocytes accumulate ~124 somatic mutations per year, a rate 3 times faster than other nondividing cells, such as neurons[60]. While this work provides the most rigorous proof that somatic mutation accumulates in the aging human heart, the functional relevance of these findings remains to be determined. Although not specific to the heart, recent work in clonal hematopoiesis of indeterminate potential (CHIP) provides insights into some of this shared pathobiology between aging and HF. CHIP refers to the clonal expansion of hematopoietic-lineage cells, which occurs due to somatic mutations in key epigenetic regulators (e.g., DNMT3A, TET2, ASXL1, JAK2). While CHIP is exceedingly rare in young adults, it increases progressively with chronological age, and is present in nearly 20% of adults greater than 90 years old[61]. CHIP is also associated with other hallmarks of biological aging, including epigenetic modifications, telomere shortening, and senescence[62-65]; however, whether there is a causal link between these hallmarks and CHIP is unclear. Notably, a series of landmark papers in 2014 and 2017 showed that CHIP is not only strongly associated with, but likely causal, in age-related atherosclerotic cardiovascular diseases[61,66]. Subsequent work has now shown similar relationships with HF[66-69]. The mechanisms by which CHIP contributes to HF pathophysiology are not fully elucidated, but likely stem from cell-intrinsic proinflammatory processes of these clonal lines, which could explain the inflammatory pathophysiology in aging and HF[70]. Emerging evidence from animal models strongly supports this hypothesis, which will be discussed in more detail in the following sections.
In summary, genomics points to common genetic drivers of human aging and HF that are rooted in senescence biology and somatic mutation-driven inflammation [Figure 3]. While epigenomics and transcriptomics, which are not reviewed in this article, provide even greater depth into how these genetic underpinnings of aging contribute to HF pathophysiology, we believe this provides a starting point for prioritizing these hallmarks of aging biology for HF therapeutic development. Next, we review how functional assessments of these candidates in preclinical models have strengthened the rationale for pursuing them in HF.
Figure 3. Shared (patho)biology of human aging and heart failure identified from genomics and proteomics. Genomics has identified senescence and age-associated accumulation of somatic mutations/inflammation as central mechanisms of aging biology that contribute to HF pathogenesis. Proteomics provides insights not only into the downstream effects of these two hallmarks of aging in HF pathogenesis, but also into the maladaptive processes in later life that link aging and HF.
Preclinical evidence supporting translation of genomic candidates in heart failure
Senescence biology
Cellular senescence is a programmed response to cell stress that occurs with aging and disease[30]. The activation of the p53/p21Cip1 and p16Ink4a/Rb pathways are central to this process, leading to a permanent state of cell cycle arrest that promotes resistance to apoptosis. Senescence is characterized by DNA damage responses, telomere shortening, senescence-associated β-galactosidase (SA-βGal) activity, mitochondrial dysfunction, and metabolic reprogramming. Notably, senescent cells remain metabolically active and develop a senescence-associated secretory phenotype (SASP) characterized by pro-fibrotic and proinflammatory factors that can lead to tissue remodeling and dysfunction[71-73].
In animal models, senescence has been linked to the physiological decline that occurs in organ systems with aging[71,74,75]. This includes the aging cardiovascular system, in which senescent cells contribute to adverse cardiac and vascular remodeling, endothelial dysfunction, and atherosclerosis[28,72,76,77]. In the aging heart, senescent cardiomyocytes produce a SASP profile that contributes to age-associated cardiac hypertrophy, fibrosis, systolic and diastolic dysfunction, and loss of regenerative potential[28,75,78]. The drivers of senescence in aged cardiomyocytes are multifold, including DNA damage, telomere damage, oxidative stress, NFkB signaling, and TGFβ signaling[28,79,80]. Senescence not only increases in the aged heart, but has also been implicated in multiple cell lineages and HF syndromes. Endothelial senescence contributes to HFpEF phenotypes, including left ventricular hypertrophy, diastolic dysfunction, and exercise intolerance, in the obese senescence-accelerated mouse[81]. Cardiomyocyte senescence contributes to maladaptive remodeling in the ischemia-reperfusion mouse model[82]. Cardiac fibroblast senescence increases in the transverse aortic constriction (TAC) model and is associated with perivascular fibrosis[83]. Recently, our group also found that placental-derived SASP contributes to cardiac dysfunction and adverse myocardial remodeling in a mouse model of peripartum cardiomyopathy (PPCM), an idiopathic form of acute HFrEF that occurs with pregnancy[84].
Conversely, decreasing senescence has been shown to improve cardiac remodeling and function in both animal models of aging and HF. In aged mice, removing senescent cells, through either pharmacologic senolytics or genetic clearance (e.g., p16-INK-ATTAC), reduces age-associated cardiac hypertrophy and myocardial fibrosis, and improves diastolic function and regenerative potential of the heart[28,75,78]. Senolytic approaches have also been shown to improve LV remodeling and systolic function in MI[82,85,86], afterload[87], chemotherapy cardiotoxicity[88], and PPCM[84] models of HF.
The pharmacologic senolytics used in the aforementioned preclinical studies include navitoclax (ABT-263), dasatinib and quercetin (D&Q), and fisetin. Navitoclax is an inhibitor of several Bcl‐2 family proteins through which it selectively induces apoptosis of senescent cells. Dasatinib is a Src/tyrosine kinase inhibitor, and quercetin a natural flavonoid that binds to Bcl-2 and modulates transcription factors, cell cycle proteins, pro- and anti-apoptotic proteins, growth factors, and protein kinases. Fisetin is a plant-based flavonoid with senolytic properties that are mediated through sirtuin activation, NF-kB deactivation, glutathione upregulation, and Bcl-xl inhibition[89]. While senolytics are entering early phase clinical trials for various indications, including pulmonary fibrosis[90], Alzheimer’s disease[91], and aging/frailty[14,92], to date, there has been no clinical trial of senolytics in HF. However, senomorphs, or drugs which do not directly kill senescent cells, but target senescence-related pathways, including SASP, are being explored in HF. One example is metformin, which is a synthetic biguanide used to treat diabetes. Although metformin likely has pleiotropic effects in HF, evidence in animal models suggests that some of its benefits are derived from inhibiting SASP[93-98]. Post-hoc analyses of clinical trials suggest that metformin potentially lowers mortality and HF hospitalizations in both HFrEF and HFpEF[99-101], and two clinical trials (NCT03629340, NCT05093959) are now testing metformin in HFpEF.
Clonal hematopoiesis and inflammation
Animal models have also been used to gain deeper mechanistic insights into how CHIP contributes to cardiac aging and HF. Although spontaneous mutations in human CHIP-associated genes (TET2, JAK2, DNMT3A) are not common in healthy aged mice, likely due to their shorter lifespan[102], various strategies to mimic clonal hematopoiesis in mice have been established. Bone marrow transfer approaches, based on competitive transplantation of mutant bone marrow cells or myeloid ablation of driver genes, have been utilized to model CHIP in rodents.
Using these techniques, a series of studies by the Walsh group has provided some of the most compelling preclinical evidence supporting the causal role of CHIP in HF pathobiology. In established murine HF models, including TAC, LAD ligation, and Ang II infusion, Tet2, Jak2, and Dnmt3a deficiency all worsened cardiac remodeling, inflammation, and dysfunction, which were rescued by targeted NLRP3 inflammasome inhibition with the small molecule MCC950[103-105]. Notably, a similar mechanistic link between CHIP mutations and the NLRP3 inflammasome occurs in the low-density lipoprotein receptor-deficient (Ldlr-/-) mouse model of atherosclerotic cardiovascular disease[106]. Similar effects of NLRP3 inhibition have also been reported in HFpEF models, in which MCC950 improves adverse cardiac and pulmonary artery remodeling, diastolic function, and overall exercise capacity, suggesting that the NLRP3- IL1β axis could be a potential therapeutic target for a broad range of HF[107].
While the aforementioned studies prove that clonal hematopoiesis is sufficient to exacerbate cardiac dysfunction in models of cardiac injury and stress, less is known about whether CHIP contributes to physiological cardiac aging. As mentioned, aged mice do not exhibit a high frequency of mutations in human CHIP-associated genes[102]. However, mice receiving Tet2-deficient bone marrow transplantation display accelerated cardiac aging with increased cardiac hypertrophy, fibrosis, inflammation, and dysfunction compared to age-matched controls[108]. Interestingly, in these mice, Tet2 deficiency also exacerbated age-related cardiometabolic phenotypes[109], which are common in older adults with HFpEF[110].
Whether CHIP pathobiology can be directly targeted as a therapy for HF is an intriguing hypothesis. Inhibitors of JAK1/2 (e.g., ruxolitinib) and TET2 (e.g., azacytidine) have been developed for oncologic treatments and, interestingly, have been shown to improve cardiac arrhythmia and failure phenotypes in murine models[111,112]. Notably, the cardiac effects of ruxolitinib are mediated through inhibition of calmodulin-dependent protein kinase II (CaMKII), which is also a mechanism by which CHIP increases atrial fibrillation propensity in mice[113]. However, the molecular mechanisms by which these cancer therapeutics modulate cardiac function are still largely unknown. Therefore, whether these drugs can be safely and effectively repurposed for HF is unclear. Of note, clinical studies have not identified any significant increase in major cardiovascular events with JAK inhibitors, but there is a potential signal for cardiotoxicity with TET2 inhibitors[114-116].
Since the mechanism linking CHIP to cardiac aging and HF is largely mediated through the NLRP3-ILβ axis, directly targeting it could be an intermediary approach. The 2017 landmark study, Canakinumab Anti-inflammatory Thrombosis Outcome Study (CANTOS), showed that neutralizing IL1β led to a 15% reduction in major adverse cardiovascular events in patients with previous myocardial infarction and high CRP[117]. Interestingly, exploratory analyses found that canakinumab not only led to a dose-dependent reduction in HF hospitalizations and mortality[118], but targeted deep sequencing also revealed that subjects with TET2 CHIP had a greater response to canakinumab than those without it (62% vs. 7% reduction in MACE)[119]. While these data support the CHIP-IL1β mechanism, prospective studies of the IL1 inhibitor Anakinra have shown mixed effects in HF. While Anakinra lowered NTproBNP and improved exercise capacity at early time points, it did not lead to improved HF outcomes at later time points, despite reductions in CRP[120]. Directly targeting the NLRP3 inflammasome may be another approach. Fibrates, which are peroxisome proliferator-activated receptor alpha (PPAR) activators used to lower cholesterol, have been associated with reduced HF hospitalizations[121]. Interestingly, they have also been shown to inhibit the NLRP3 inflammasome and improve cardiac inflammation and function in a steatohepatitis-associated cardiomyopathy model[122]. The safety of novel NLRP3 inhibitors is now be testing in humans (NCT06336005), which could pave the way for this approach. Ultimately, understanding the pathobiology of CHIP in age-related cardiovascular disease, and identifying those who might benefit the most from CHIP-targeted therapeutics, are important to developing therapeutics that target the inflammaging that accompanies somatic mutations.
PROTEOMICS
Proteomics of human aging and heart failure
Proteomics is the comprehensive study of all proteins in a given biological matrix. Unlike one’s germline DNA, which is more or less fixed, proteins are downstream effectors of genes that are highly dynamic, both in terms of their expression levels and post-translational modifications. While this introduces various challenges in interpreting proteomic data, the dynamic nature of the proteome is potentially better aligned with the temporal physiologic and pathologic changes that occur with aging and disease. The incorporation of genetic instruments (e.g., pQTL, MR) into proteomic analyses can help infer causal versus (mal)adaptive compensatory roles of proteins associated with complex traits and can prioritize those with the highest druggable potential[123,124]. Notably, proteins currently represent the most common target in clinical biomarker and drug development.
In the past decade, dramatic advancements in high-throughput, multiplex proteomic platforms, including those based on mass spectrometry, modified aptamers (SOMAscan), and proximity extension assays (Olink) now enable simultaneous measurement of thousands of proteins (Olink ~5,400, SOMAscan ~11,000) with relatively small quantities (< 100 μL) of biospecimen. Paired with the extensive biobanking and phenotyping efforts of large population cohorts (e.g., UK Biobank), these commercial-based platforms have led to multiple large-scale proteomic studies in both aging and HF. Here, we focus on blood-based (plasma/serum) proteomic studies, but point out that these studies are not tissue-specific (e.g., heart), nor do they address the effects of post-translational modifications or the differences in platforms used[125,126]. Despite these limitations, blood-based proteomics has provided valuable insights into the shared biology of human aging and HF.
In the context of aging, while early studies adopting these technologies primarily focused on proteomic signatures associated with chronological age[127-130], more recent efforts have shifted toward using proteomic profiling to gain mechanistic insights into the clinical phenotypes (e.g., longevity, frailty)[131-135] and intrinsic variability of biological aging[129,136,137] [Table 2]. Despite differences in the cohorts, platforms, and aging phenotypes (i.e., chronologic age vs. longevity vs. frailty), there has been notable consistency in the proteomic signatures identified across these studies. In particular, aging phenotypes are consistently associated with proteins of TGFβ family signaling (FSTL3, MIC-1/GDF15, GDF11/8, TAGL, LEFTY2), ErbB family signaling (ERBB1, FBLN3), inflammation (sTNFR, TREM1, HAVCR2, a2-microglobulin, WFDC), insulin/growth signaling (IGFBP2, IGFBP6, IGFBP7), fibrosis (TIMP1, TSP2, MMPs), vasculogenesis (ANGPT2, SVEP1, VEGF), and metabolism (FABP3, FABP4). Many of these proteins are part of the SASP[73,138,139], suggesting that some of the proteomic signatures of aging may reflect downstream effects of primary senescence biology. Notably, despite these studies excluding subjects with HF, NTproBNP, a clinical biomarker of HF, has consistently emerged as one of the most highly associated proteins with chronologic age, longevity, and frailty. This suggests that either HF is not well adjudicated in these studies, which is unlikely given the consistency of this signal in different cohorts, or current methods are not sufficient to adequately control for the strength of shared biology between aging and HF.
Proteomic studies in human aging and heart failure. This table summarizes recent blood-based (plasma or serum) proteomic studies that test associations of circulating proteins with aging (chronological age, longevity, frailty) or incident HF (and HF endophenotypes). Only the top 30 most significant proteins from each study are included. Bold indicates proteins that were inversely associated with the phenotype
Study | Proteomic platform | Discovery cohort | Validation cohort | Protein associations (up to top 30 most significant) |
Chronological age | ||||
Menni et al., (2015)[127] | SOMAscan, 1.1 K | TwinsUK (n = 206) | AddNeuroMed, Alzheimer’s Research UK/Maudsley MRC Dementia Case Registry (n = 677) | CHRDL1, CCDC80, PTN, FSTL3, TIMP1, MMP12, Cystatin C, IGFBP6, ROR1, THBS4, HAVCR2, ADAMTS5, EDA2R |
Tanaka et al., (2018)[128] | SOMAscan, 1.3 K | BLSA, GESTALT (n = 240) | NA | MIC-1/GDF15, PTN, ADAMTS5, FSH, SOST, CHRDL1, NTproBNP, FBLN3, MMP12, Cathepsin V, FSTL3, b2-microglobulin, hCG, CAPG, sTNFR-I, TIMP1, NBL1, CCD80, Cystatin C, CHIT1, IGFBP2, RET, EPHA2, LUM, IL18BP, PPY, LTBP4, IGFBP6, DSC2, SMOC1 |
Lehallier et al., (2019)[129] | SOMAscan, (1.3 K, 4.0 K, 5.0 K) | INTERVAL, LonGenity (n = 4,263) | VASeattle, PRIN07, PRIN09, GEHA | SCARF2, ARFIP2, SOST, PTN, WFDCC2, AGRP, MIC-1/GDF15, MXRA8, IGDCC4, FSH, CD93, MSMP, NTRK3, CHAD, ADAMTS5, COL15A1, MLN, CDON, KLK3, SCG3, PTPRD, KLK7, C1QTNF3, DKL1, CTSF, SSVEP1, DSG2, RET, CCDC80 |
Sathyan et al., (2020)[130] | SOMAscan, 5.0 K | LonGenity (n = 1,025; n = 506 OPEL; n = 519 OPUS) | NA | PTN, WISP-2, CHRDL1, TAGL, RSPO1, FBLN3, ERBB1, MIC-1/GDF15, SMOC1, HE4, PGD2 synthase, Cystatin C, FSTL3, RNASE1, MSR1, URB, CCD80, a2-Antiplasmin, sTREM1, NTproBNP, SREC-II, b2-Microglobulin, ASB9, CDCP1, HPB6, ATS13, TNF sR-II, SVEP1, SAP33, TMEDA, SET |
Longevity | ||||
Orwoll et al., (2020)[131] | SOMAscan, 4.0 K | MrOs (n = 2,473) | NA | C9, S100A9, CD163, CRP, IGHM, C7, FCG3A, LG3BP, NRP1, CD166, PHLD, b2-microglobulin, a2-microglobulin, MMP2, vWF, CSF-1R, HPRT, CFAD, CD5L, FCGBP, IGHG3, Prothrombin, Cystatin C, PTGDS, MUC18 |
Liu et al., (2024)[134] | SOMAscan, 5.0 K | CHS (n = 3,067) | AGES-Reykjavik (n = 4,690) | MIC-1/GDF15, NTproBNP, ERBB1, TSP2, MMP-12, Amyloid-like protein 1, CILP2, Endostatin, NOTUM, CD14, Protein C, SRSF7, TWSG1, PXDN, b2-Microglobulin, RNase 1, NG36, SET, Epididymal secretory protein E1, EPHAA, HE4, Angiopoietin-2, aTL3, sTNFR-II, BAGE2, TRA2B, MXRA8, Cystatin C, FBLN3, EWS |
Frailty | ||||
Sathyan et al., (2020)[232] | SOMAscan, 5.0 K | LonGenity (n = 880) | NA | ANTR2, FABP3, FABP4, ERBB1, NELL1, MXRA8, CA2D3, Leptin, DNER, PTGD2, NCAM-120, CSPG3, Coag Factor IXab, GDF11/8, Contatin-1, NG36, ADAM22, ENPP5, HS6ST3, IL-1Ra, FSTL3, PXDN, HTRA1, ARFP2, ANTR1, FPRP, SSRF7, Coag Factor IV, NEO1, Tenascin-X |
Liu et al., (2022)[134] | SOMAscan, 4.0 K | CHS (n = 2,854) | FHS (n = 1,130) | MIC-1/GDF15, PTN, TIMP1, Troponin T, IL-7Ra, NET1, PARC, DAN, ANGPT2, FSTL3, URB, CD59, RSPO3, Carbonic anhydrase 3, CDON, SMOC1, SHP-2, RANTES, Cathepsin D, PHI, Endostatin, eIF-5, ERBB1, CRK, Cripto, GSTP1, Ubiquitin, C1R, MMP7 |
Liu et al., (2023)[133] | SOMAscan, 4.0 K | ARIC (n = 3,838 cross-section; n = 1,725 longitudinal) | CHS (n = 2,570 cross-sectional; | TREM1, FABP3, TAGL, FABP4, CAPG, NBL1, RNASE1, MYL6B, CBLN4, HSPB6, HAVCR2, NDST1, MIC-1/GDF15, WFDC2, FSTL3, LEFTY2, PCCDHGA12, sTNFR-1, TFF2, VEGFA, PTN, NET1, DNAJB9, SUMF1, Angiopoietin-2, SELH, COL28A1, REG3A, NTproBNP, WFDC1 |
Mortality | ||||
Kuo et al., (2024)[135] | Olink, 3,072 | UKBiobank (n = 53,021) | NA | MIC-1/GDF15, WFDC2, NEFL, EDA2R, PLAUR, TFFR, sTNFR-I, MMP12, LTBP2, AREG, HGF, ADM, IGFBP4, VSIG4, TGFA, CEACAM5, PIGR, IL6, FGL1, LAMP3, THBS2, CLEC3B, IFI30, OSM, IL32RA, NTproBNP, CSF1, Angiopoietin2 |
Incident Heart Failure | ||||
Ferreiera et al., 2019[140] | Olink, CVD II, CVD III, inflammation panels | HOMAGE (n = 877) | HOMAGE (n = 556) | NTproBNP, TRAILR2, sTNFR-1, GAL-9, GFG23, REN, MIC-1/GDF15, FABP4, SLAMF7, CCL16, TWEAK, KIM1, CD4, VSIG2, PON3, PLFG, MMP-12, ADM, RARRESS2, CEACAM8, SLAMF1, AGRP, TNFR2, IGFBP7, UPAR, PAR1, PLC, ACE2, IL16, TFF3, OPN, SPON2, ILLR-4AR, TNFRSF14 |
Nayor et al., 2020[141] | SOMAscan, 5.0 K | FHS (n = 1,913) | HUNT (2,515) | NTproBNP, ERBB1, MBL, TRMC, GDF-11/8, TSP2, NRP1, vWF, ApoE4, ApoE3, RGMA, NOTCH 1, MnSOD, RGMC, IL-1Ra, NEGR1, APO L1, ISLLR2, VEGFA sR2, S100A2, TNFa, MIC-1/GDF15, Cathepsin D, NCAM-120, DKK4, IgG, C7, PEX5, BMPR1A |
Girerd et al., 2023[142] | O-link, CVD II, CVD III, inflammation panels | ARIC (n = 500); FHS (n = 382); HOMAGE (1,433) | NA | NTproBNP, BNP, 4E-BP1, HGF, Gal-9, TGFA, THBS2, U-PAR (significant in all three cohorts) |
Emilsson et al., 2023[143] | SOMAscan, 5.0 K | AGES-Reykjavik (n = 5,457) | CHS (n = 3,484) | NTproBNP, MIC-1/GDF15, CTSH, Cystatin C, RNASE1, Angiopoietin-2, GABARAPL1, MMP12, SVEP1, b2-microglobulin, RELT, MFAP2, PXDN, FABP3, TMPO, ATP5J, COL28A1, FABP4, WFDC2, TFF3, TMED10, MMP7, SPON1, PLAUR, ADM, TNNI3, CCL15, COL6A3, PCDHGA10, TAGL, MDM1 |
Shah et al., 2024[144] | SOMAscan, 5.0 K Olink, 3,072 | ARIC mid-life (n = 10,638), ARIC late-life (n = 4,483), HUNT (n = 3,262) | NA | NTproBNP, SVEP1, WFDCC2, Angiopoietin-2, MIC-1/GDF15, SPON1, THBS2, CILP2, GHR, FSTL3, TAGL, IGFBP2, APOF, TREM1, FSTL1, TIMP4, C9, ANGPTL3, SLIT2, IGFBP7, sTNFR-1, MFAP4, CCL15, ITIH3, NRP1, CELA1, ERBB1, CACNA2D3, CLEC3B, PTPRD, ATP1B1 |
Incident frailty and heart failure | ||||
Ramonfaur et al., 2024[145] | SOMAscan, 5.0 K | ARIC mid-life (n = 10,638) ARIC late-life (n = 3,908) | CHF (n = 3,189) | COLL28A1, COL6A3, WFDC2, PXDN, LEFTY2, TAGL, GABARAP, CST3, sTNFR -I, NBL1, FSTL3, MIC-1/GDF15, TMED10, RNAse1, FBLN3, TWSG1, TREM1, WFDC1 |
In favor of the latter hypothesis is the striking observation that even after adjusting for age, the majority of circulating proteins associated with incident HF are similar to those associated with aging [Table 2][140-144]. Indeed, a recent large-scale plasma proteomics study of nearly 15,000 subjects identified a similar proteomic signature for incident HF and frailty that largely mirrored circulating proteins implicated in independent aging and HF studies. Of these, FSTL3/GDF15 (TGFβ family signaling), FBLN3 (ErbB family signaling), and TREM1/WFDC1 (inflammation) emerged as potential causal effectors for both frailty and HF (or HF endophenotypes)[145].
In summary, the similar proteomic signatures associated with aging and HF, despite controlling for each other, highlight how strong the systemic biology is between these entities. In many ways, the signatures could reflect downstream effects of senescence and somatic mutation-induced inflammation identified at the genomic level. However, we propose that it likely also reflects the maladaptive compensatory mechanisms that underlie aging and HF (patho)physiology [Figure 3]. Next, we discuss the two candidate processes that exhibit the most consistent positive and negative associations with aging and HF.
Preclinical evidence supporting translation of proteomic candidates in heart failure
Transforming growth factor-β superfamily signaling
While many circulating proteins are associated with both aging and HF, the most highly associated with both have consistently been FSTL3 and GDF15 [Table 2], which are key members of the TGFβ family. In humans, the TGFβ family consists of more than 30 ligands, including TGFβs, activins, lefty,
FSTL3 is a secreted glycoprotein that neutralizes circulating activins, TGFβs, GDF11, and GDF8. It is a downstream target of Smad 2/3 signaling and functions as a negative feedback loop for ActRII and TGFBR2 receptor signaling. Thus, increased circulating FSTL3 can be interpreted as an indicator of increased signaling through these receptors after binding their cognate ligands. Notably, all four ligands have been implicated in cardiac aging and HF, with the first three also being components of the SASP[148]. GDF11 and activins have recently garnered significant attention in cardiac aging and HF, and thus, our discussion will focus on these ligands in addition to GDF15.
GDF11’s role in cardiac aging and HF emerged from a heterochronic parabiosis study in mice, in which it was identified as a potential rejuvenating factor that reverses age-related cardiac hypertrophy, sarcopenia, and impaired neurogenesis[149-151]. Follow-up work, however, identified specificity issues with the aptamer used to identify GDF11, suggesting that it also binds the closely homologous GDF8, which was likely responsible for the anti-hypertrophic effects observed in these original studies[152,153]. A series of studies have confirmed that GDF11 does reverse cardiac hypertrophy, but at higher doses, it becomes deleterious, causing severe cachexia and increased mortality[154,155]. The effects of GDF11 on cardiac function are not clear with studies showing that it both worsens and improves cardiac function in animal models of HF[156-159]. In humans, while GDF11 was initially thought to decline with aging, highly specific LC-MS/MS have found that circulating GDF11 levels do not change with age, nor do they relate to increased HF risk in older adults[153,160]. However, consistent with plasma/serum proteomics studies [Table 2], LC-MS/MS assays have confirmed that FSTL3 is strongly associated with HF in older adults, particularly HFpEF[160]. Based on the mixed preclinical and clinical evidence, GDF11 is not currently being tested as a therapeutic for HF. If it is to be pursued in the future, careful dose optimization and monitoring will be required to ensure safety with its catabolic effects.
Activins, specifically activin A, also increase FSTL3 expression and have been proposed to be one of the major TGFβ family ligands responsible for the increased circulating FSTL3 signal observed in human aging and HF[161]. Circulating activin A consistently increases with chronologic age in both sexes, particularly in the last decades of life[162], and in those with frailty[163]. Similar findings are also observed in mice, where circulating activin A levels increase with age and correspond to age-associated increases in cardiac FSTL3 expression[161]. Moreover, in both naturally aged mice and progeria models (Ercc1 Δ/-), inhibition of activin A, through either antibodies against its receptors (ActRII) or soluble ActRII ligand traps, improves age-associated cardiac dysfunction and remodeling[161,164]. These findings extend to HF in both humans and rodent models. Circulating activin A levels correlate with worse HF severity in humans[84,161,165]. Conversely, inhibition of activin A or ActRII improves cardiac function and remodeling in multiple animal models of HF, including TAC, LAD ligation, aging, cancer, dilated cardiomyopathy, and PPCM[84,161,166-168]. The mechanisms underlying the benefits of activin-A/ActRII inhibition in HF are multifold, encompassing effects on proteostasis, Ca2+ handling, fibrosis, energetics, metabolism, and senescence. It is important to note that while chronic increases in activin A are sufficient to induce cardiac dysfunction[161,169], acute increases have been shown to improve cardiomyocyte survival in ischemic injury[170]. While this may seem contradictory, it likely follows the paradigms underlying aging and HF, in which chronic activation of survival pathways becomes maladaptive. Targeting activin-A/ActRII biology in HF follows the recent success of inhibiting it in pulmonary artery hypertension (PAH)[171,172]. Sotatercept, an ActRIIA-Fc that has been FDA-approved for PAH and has been shown to have anti-inflammatory and anti-proliferative effects on vascular cells[173], is now being tested in HFpEF (NCT04945460), while bimagrumab, an antibody to ActRII which has beneficial metabolic remodeling and antifibrotic effects[174,175], is being tested in obesity/cardiometabolic disease (NCT05616013).
Unlike GDF11 and activin A, growth differentiation factor 15 (GDF15) is a distant member of the TGFβ superfamily that does not bind to any of its 13 receptors, but rather the orphan receptor, GDNF-family receptor a-like (GFRAL)[176]. GDF15 is expressed at very low levels under physiological conditions, but is markedly upregulated following injury. As highlighted in the proteomics of human aging and HF [Table 2], GDF15 is strongly associated with aging, frailty, and HF, and is a predictor of all-cause mortality[177-179]. However, it remains unclear whether GDF15 is just a biomarker of cellular injury and inflammation in these contexts or if it plays a functional role that can be targeted for therapeutic development. Multiple aspects of GDF15’s biology suggest that similar to activins and senescence, it likely follows the same evolutionary principles that dictate aging and disease[180]. GDF15 exhibits potent anti-inflammatory and catabolic effects[181,182], and has been shown to increase longevity in mice[182]. In the cardiovascular system, GDF15 increases endothelial senescence/dysfunction and cardiac fibrosis[183-185], but in HF models, induced by LPS, TAC, or ischemia/reperfusion, GDF15 is generally cardioprotective[181,186-188]. However, emerging evidence suggests that inhibiting GDF15 could conversely improve cardiac cachexia and HF progression by blocking atrophy pathways induced by GDF15[189-192]. While the catabolic effects of GDF15 largely mirror that of GDF11, a notable difference between these TGFβ family members is that GDF15 levels are consistently and highly increased in aging and HF, while GDF11 levels are generally unchanged in these contexts. This makes the former perhaps more relevant to aging and HF pathobiology. While no clinical trials targeting GDF15 are currently underway in HF, both antagonistic and agonist tools are being explored for other clinical indications. Visugromab, a GDF15 neutralizing antibody, is being tested in patients with advanced stages of cancer (NCT06059547, NCT04725474), while LY3463251, a long-acting GDF15 receptor agonist, is being investigated as an anti-obesity treatment, though dose-dependent nausea and emesis may be a limiting factor with the latter[193].
ErbB family signaling
While proteins implicated in TGFβ family signaling are consistently among the most positively associated with human aging and HF, the only protein that is negatively associated with both is ErbB1. The ErbB proteins represent a family of four closely related tyrosine kinase receptors, which include ErbB1 (also known as epidermal growth factor receptor EGFR), ErbB2, ErbB3, and ErbB4. The ErbB receptors bind a large family of ligands, including EGF, neuregulins (NRGs) 1-4, TGF, amphiregulin, betacellulin, epiregulin, and epigen. The ErbB family of ligands-receptors are essential in cardiac development, but have also been implicated in disease, including HF[194,195].
NRGs, in particular, have gained attention in HF research, given their therapeutic potential. NRG-1 is the most abundantly expressed member of this family in the heart. There are two variants of NRG1, α and β, with NRG1β being the more active isoform. NRG1 binds directly to ErbB3 and ErbB4, which in coordination with the orphan receptor ErbB2, form homo- and hetero-dimers. Signaling pathways through ErbB include PI3K-AKT, ERK-1/2, and Hippo-YAP, which regulate cardiomyocyte survival, growth, and proliferation[196-198]. NRG1 also plays a central role in the development and function of non-cardiomyocyte lineages, including neurons, endothelial cells, fibroblasts, and immune cells[199-204], and serves as a central regulator of the intercellular crosstalk and interplay between cardiomyocytes, the microvasculature, and conduction system in the heart.
While NRG1/ErbB signaling has been studied extensively in early cardiac development, its role in cardiac aging is less well understood. Sustained higher circulating levels of NRG1 have been found in long-lived species such as the naked mole rat[205], suggesting that it may play a role in promoting survival and longevity. NRG has also been shown to attenuate vascular senescence[206], and in the aged heart, downregulation of NRG/ErbB signaling is associated with mitochondrial dysfunction and cardiomyocyte apoptosis[207]. As a mediator of the adaptive response to physiological stress, NRG1 also contributes to physiological cardiac hypertrophy in both pregnancy and exercise[208,209].
The potential therapeutic role of NRG/ErbB signaling in HF emerged from clinical safety data indicating an increased risk of HF and systolic dysfunction in patients with breast cancer being treated with trastuzumab (a humanized monoclonal antibody targeting ErbB2)[210,211]. This observation was supported by evidence from transgenic animal models, which showed that cardiac or cardiomyocyte-specific genetic ablation of ErbB2 or ErbB4 was sufficient to induce dilated cardiomyopathy[212-214]. NRG1/ErbB signaling was subsequently shown to be reduced in both human and experimental HF models[215,216], and activating cardiac ErbB signaling via recombinant NRG1 improved cardiac function and survival in multiple animal models of HF[217]. The mechanisms by which NRG1/ErbB improves cardiac function are multifold, including its effects on cardiomyocyte Ca2+ signaling, sarcomere function, hypertrophy, metabolism, survival, and regeneration, as well as its impact on angiogenesis and fibrosis[196,197,202,204,206,218,219].
Based on the collective preclinical and clinical evidence, multiple clinical trials testing the safety and efficacy of recombinant NRG1 have been implemented in HF[220,221]. In patients with chronic HF, short-term treatment of rhNRG1 (Neucardin) improved hemodynamics, LVEF, and some metrics of adverse LV remodeling. A larger full-length recombinant NRG-1B3 (cimaglermin) has also been tested in chronic HFrEF, and has similarly shown improvements in LVEF, but dose-limiting toxicity with liver injury[222]. Studies in rodent and swine models suggest that the cardiac benefits of NRG-1B3 are likely mediated through improvements in cardiomyocyte mitochondrial function and reduced cardiac fibroblast activation[223,224]. Lastly, in attempts to avoid potential cancer-promoting effects of ErbB2 activation, engineered ErbB3-NRG1 antibody constructs (JK07) have been designed to bias signaling toward ErbB4. An early phase I study shows this approach is safe, with signals suggesting it increases LVEF in patients with chronic HFrEF[225]. Similar to NRG-1B3, preclinical data from rodents and non-human primate HF models suggest that JK07 reduces myocardial fibrosis and improves diastolic function[226]. Phase II/III studies testing the efficacy of recombinant NRG1 (NCT03388593) and JK07 (NCT06369298) in chronic HFrEF are currently underway.
SUMMARY
Advancements in our understanding of the biology of aging are changing the perception of age in HF. The ability to modify biological aging and effectively alter HF pathophysiology offers the exciting possibility that aging itself could represent a new area for therapeutic target discovery for HF. While Geroscience is gaining traction in cardiovascular disease research[14,38], ensuring its success and sustainability in the field will inevitably require that certain metrics be achieved in the coming years. Given that the overarching mission of Geroscience is to tackle chronic diseases by targeting aging biology, it is imperative that the investment into this emerging area of interdisciplinary research fulfills its promise of developing effective gerotherapeutics. HF, with its overwhelming prevalence in the elderly population and its markedly similar (patho)biology and physiology to aging, perhaps represents the ideal challenge for Geroscience. As highlighted in this review, prioritizing those targets with strong evidence based on the shared biology of human aging and HF will ultimately enhance the translational success of this Geroscience approach and hopefully change the trajectory of HF in older adults.
DECLARATIONS
Author’s contributions
Conceptualized the review: Castro C, Roh J
Wrote and edited the manuscript: Castro C, Delwarde C, Shi Y, Roh J
Designed figures: Shi Y, Roh J
Availability of data and materials
Not applicable.
Financial support and sponsorship
This work is supported by the National Institute of Health (R01-HL170058), MGH Transformative Scholars Award, Hassenfeld Scholars Award, and Fred and Ines Yeatts Fund for Innovative Research to Roh J.
Conflicts of interest
Roh J receives research support from Keros Therapeutics, Genentech, and Amgen, all of which are unrelated to this work. All other authors declared that there are no conflicts of interest.
Ethical approval and consent to participate
Not applicable.
Consent for publication:
Not applicable.
Copyright
© The Author(s) 2025.
REFERENCES
1. Bozkurt B, Coats AJ, Tsutsui H, et al. Universal definition and classification of heart failure: a report of the heart failure society of America, heart failure association of the European society of cardiology, Japanese heart failure society and writing committee of the universal definition of heart failure. J Cardiac Fail. 2021; doi: 10.1016/j.cardfail.2021.01.022.
2. Savarese G, Becher PM, Lund LH, Seferovic P, Rosano GMC, Coats AJS. Global burden of heart failure: a comprehensive and updated review of epidemiology. Cardiovasc Res. 2023;118:3272-87.
3. Martin SS, Aday AW, Almarzooq ZI, et al. 2024 heart disease and stroke statistics: a report of US and global data from the American heart association. Circulation. 2024;149:e347-913.
4. Bozkurt B, Ahmad T, Alexander KM, et al. Heart failure epidemiology and outcomes statistics: a report of the heart failure society of America. J Card Fail. 2023;29:1412-51.
5. Curtis LH, Greiner MA, Hammill BG, et al. Early and long-term outcomes of heart failure in elderly persons, 2001-2005. Arch Intern Med. 2008;168:2481-8.
6. Mann DL, Bristow MR. Mechanisms and models in heart failure: the biomechanical model and beyond. Circulation. 2005;111:2837-49.
7. Maurer MS, Schwartz JH, Gundapaneni B, et al. Tafamidis treatment for patients with transthyretin amyloid cardiomyopathy. N Engl J Med. 2018;379:1007-16.
8. Olivotto I, Oreziak A, Barriales-Villa R, et al. Mavacamten for treatment of symptomatic obstructive hypertrophic cardiomyopathy (EXPLORER-HCM): a randomised, double-blind, placebo-controlled, phase 3 trial. Lancet. 2020;396:759-69.
9. McMurray JJV, Solomon SD, Inzucchi SE, et al. Dapagliflozin in patients with heart failure and reduced ejection fraction. N Engl J Med. 2019;381:1995-2008.
10. Anker SD, Butler J, Filippatos G, et al. Empagliflozin in heart failure with a preserved ejection fraction. N Engl J Med. 2021;385:1451-61.
11. Kosiborod MN, Abildstrøm SZ, Borlaug BA, et al. Semaglutide in patients with heart failure with preserved ejection fraction and obesity. N Engl J Med. 2023;389:1069-84.
12. López-Otín C, Blasco MA, Partridge L, Serrano M, Kroemer G. Hallmarks of aging: an expanding universe. Cell. 2023;186:243-78.
13. Li H, Hastings MH, Rhee J, Trager LE, Roh JD, Rosenzweig A. Targeting age-related pathways in heart failure. Circ Res. 2020;126:533-51.
14. Goyal P, Maurer MS, Roh J. Aging in heart failure: embracing biology over chronology: JACC family series. JACC Heart Fail. 2024;12:795-809.
15. Sierra F. The emergence of geroscience as an interdisciplinary approach to the enhancement of health span and life span. Cold Spring Harb Perspect Med. 2016;6:a025163.
16. Dock W. Presbycardia or aging of the myocardium. NY State J Med. 1945;45:983-6.
17. Tromp J, Paniagua SMA, Lau ES, et al. Age dependent associations of risk factors with heart failure: pooled population based cohort study. BMJ. 2021;372:n461.
18. Strait JB, Lakatta EG. Aging-associated cardiovascular changes and their relationship to heart failure. Heart Fail Clin. 2012;8:143-64.
19. Lakatta EG, Levy D. Arterial and cardiac aging: major shareholders in cardiovascular disease enterprises: part II: the aging heart in health: links to heart disease. Circulation. 2003;107:346-54.
20. Pandey A, Kraus WE, Brubaker PH, Kitzman DW. Healthy aging and cardiovascular function: invasive hemodynamics during rest and exercise in 104 healthy volunteers. JACC Heart Fail. 2020;8:111-21.
21. Roh J, Rhee J, Chaudhari V, Rosenzweig A. The role of exercise in cardiac aging: from physiology to molecular mechanisms. Circ Res. 2016;118:279-95.
22. Abdellatif M, Rainer PP, Sedej S, Kroemer G. Hallmarks of cardiovascular ageing. Nat Rev Cardiol. 2023;20:754-77.
23. Xie S, Xu SC, Deng W, Tang Q. Metabolic landscape in cardiac aging: insights into molecular biology and therapeutic implications. Signal Transduct Target Ther. 2023;8:114.
24. Williams GC. Pleiotropy, natural selection, and the evolution of senescence. Eolution. 1957;11:398-411.
26. Hua Y, Zhang Y, Ceylan-Isik AF, Wold LE, Nunn JM, Ren J. Chronic AKT activation accentuates aging-induced cardiac hypertrophy and myocardial contractile dysfunction: role of autophagy. Basic Res Cardiol. 2011;106:1173-91.
28. Anderson R, Lagnado A, Maggiorani D, et al. Length-independent telomere damage drives post-mitotic cardiomyocyte senescence. EMBO J. 2019;38:e100492.
29. Hartupee J, Mann DL. Neurohormonal activation in heart failure with reduced ejection fraction. Nat Rev Cardiol. 2017;14:30-8.
30. Campisi J, d'Adda di Fagagna F. Cellular senescence: when bad things happen to good cells. Nat Rev Mol Cell Biol. 2007;8:729-40.
31. Kenyon C, Chang J, Gensch E, Rudner A, Tabtiang R. A C. elegans mutant that lives twice as long as wild type. Nature. 1993;366:461-4.
32. Kenyon C. The first long-lived mutants: discovery of the insulin/IGF-1 pathway for ageing. Philos Trans R Soc Lond B Biol Sci. 2011;366:9-16.
33. Zhang WB, Ye K, Barzilai N, Milman S. The antagonistic pleiotropy of insulin-like growth factor 1. Aging Cell. 2021;20:e13443.
34. Milman S, Huffman DM, Barzilai N. The somatotropic axis in human aging: framework for the current state of knowledge and future research. Cell Metab. 2016;23:980-9.
35. Vasan RS, Sullivan LM, D'Agostino RB, et al. Serum insulin-like growth factor I and risk for heart failure in elderly individuals without a previous myocardial infarction: the Framingham Heart Study. Ann Intern Med. 2003;139:642-8.
36. Abdellatif M, Madeo F, Kroemer G, Sedej S. Spermidine overrides INSR (insulin receptor)-IGF1R (insulin-like growth factor 1 receptor)-mediated inhibition of autophagy in the aging heart. Autophagy. 2022;18:2500-2.
37. Abdellatif M, Trummer-Herbst V, Heberle AM, et al. Fine-tuning cardiac insulin-like growth factor 1 receptor signaling to promote health and longevity. Circulation. 2022;145:1853-66.
38. Forman DE, Kuchel GA, Newman JC, et al. Impact of geroscience on therapeutic strategies for older adults with cardiovascular disease: JACC scientific statement. J Am Coll Cardiol. 2023;82:631-47.
39. Dowden H, Munro J. Trends in clinical success rates and therapeutic focus. Nat Rev Drug Discov. 2019;18:495-6.
40. Vatner SF. Why so few new cardiovascular drugs translate to the clinics. Circ Res. 2016;119:714-7.
41. Roh J, Hill JA, Singh A, Valero-Muñoz M, Sam F. Heart failure with preserved ejection fraction: heterogeneous syndrome, diverse preclinical models. Circ Res. 2022;130:1906-25.
42. Haldar SM. Keeping translational research grounded in human biology. J Clin Invest. 2024;134:e178332.
43. Nelson MR, Tipney H, Painter JL, et al. The support of human genetic evidence for approved drug indications. Nat Genet. 2015;47:856-60.
44. Rosenzweig A. The growing importance of basic models of cardiovascular disease. Circ Res. 2022;130:1743-6.
45. Kaplanis J, Gordon A, Shor T, et al. Quantitative analysis of population-scale family trees with millions of relatives. Science. 2018;360:171-5.
46. Deelen J, Beekman M, Uh HW, et al. Genome-wide association meta-analysis of human longevity identifies a novel locus conferring survival beyond 90 years of age. Hum Mol Genet. 2014;23:4420-32.
47. Broer L, Buchman AS, Deelen J, et al. GWAS of longevity in CHARGE consortium confirms APOE and FOXO3 candidacy. J Gerontol A Biol Sci Med Sci. 2015;70:110-8.
48. Yashin AI, Wu D, Arbeeva LS, et al. Genetics of aging, health, and survival: dynamic regulation of human longevity related traits. Front Genet. 2015;6:122.
49. Zeng Y, Nie C, Min J, et al. Novel loci and pathways significantly associated with longevity. Sci Rep. 2016;6:21243.
50. Gurinovich A, Song Z, Zhang W, et al. Effect of longevity genetic variants on the molecular aging rate. Geroscience. 2021;43:1237-51.
51. Deelen J, Evans DS, Arking DE, et al. A meta-analysis of genome-wide association studies identifies multiple longevity genes. Nat Commun. 2019;10:3669.
52. Atkins JL, Pilling LC, Ble A, et al. Longer-lived parents and cardiovascular outcomes: 8-year follow-up in 186,000 U.K. biobank participants. J Am Coll Cardiol. 2016;68:874-5.
53. Smith NL, Felix JF, Morrison AC, et al. Association of genome-wide variation with the risk of incident heart failure in adults of European and African ancestry: a prospective meta-analysis from the cohorts for heart and aging research in genomic epidemiology (CHARGE) consortium. Circ Cardiovasc Genet. 2010;3:256-66.
54. Shah S, Henry A, Roselli C, et al. Genome-wide association and Mendelian randomisation analysis provide insights into the pathogenesis of heart failure. Nat Commun. 2020;11:163.
55. Levin MG, Tsao NL, Singhal P, et al. Genome-wide association and multi-trait analyses characterize the common genetic architecture of heart failure. Nat Commun. 2022;13:6914.
56. Joseph J, Liu C, Hui Q, et al. Genetic architecture of heart failure with preserved versus reduced ejection fraction. Nat Commun. 2022;13:7753.
57. Rasooly D, Peloso GM, Pereira AC, et al. Genome-wide association analysis and mendelian randomization proteomics identify drug targets for heart failure. Nat Commun. 2023;14:3826.
58. Yousefzadeh M, Henpita C, Vyas R, Soto-Palma C, Robbins P, Niedernhofer L. DNA damage-how and why we age? Elife. 2021;10:e62852.
59. Vijg J, Dong X. Pathogenic mechanisms of somatic mutation and genome mosaicism in aging. Cell. 2020;182:12-23.
60. Choudhury S, Huang AY, Kim J, et al. Somatic mutations in single human cardiomyocytes reveal age-associated DNA damage and widespread oxidative genotoxicity. Nat Aging. 2022;2:714-25.
61. Jaiswal S, Fontanillas P, Flannick J, et al. Age-related clonal hematopoiesis associated with adverse outcomes. N Engl J Med. 2014;371:2488-98.
62. Nachun D, Lu AT, Bick AG, et al. Clonal hematopoiesis associated with epigenetic aging and clinical outcomes. Aging Cell. 2021;20:e13366.
63. Mack TM, Raddatz MA, Pershad Y, et al. Epigenetic and proteomic signatures associate with clonal hematopoiesis expansion rate. Nat Aging. 2024;4:1043-52.
64. Zhang CR, Ostrander EL, Kukhar O, et al. Txnip enhances fitness of Dnmt3a-mutant hematopoietic stem cells via p21. Blood Cancer Discov. 2022;3:220-39.
65. Singh S, Sarkar T, Gudmundsson KO, et al. Id1 promotes clonal hematopoiesis in mice with Tet2 loss of function. bioRxiv. 2024; doi: 10.1101/2024.11.19.624318.
66. Jaiswal S, Natarajan P, Silver AJ, et al. Clonal hematopoiesis and risk of atherosclerotic cardiovascular disease. N Engl J Med. 2017;377:111-21.
67. Yu B, Roberts MB, Raffield LM, et al. Supplemental association of clonal hematopoiesis with incident heart failure. J Am Coll Cardiol. 2021;78:42-52.
68. Pascual-Figal DA, Bayes-Genis A, Díez-Díez M, et al. Clonal hematopoiesis and risk of progression of heart failure with reduced left ventricular ejection fraction. J Am Coll Cardiol. 2021;77:1747-59.
69. Schuermans A, Honigberg MC, Raffield LM, et al. Clonal hematopoiesis and incident heart failure with preserved ejection fraction. JAMA Netw Open. 2024;7:e2353244.
70. Abplanalp WT, Cremer S, John D, et al. Clonal hematopoiesis-driver DNMT3A mutations alter immune cells in heart failure. Circ Res. 2021;128:216-28.
72. Mehdizadeh M, Aguilar M, Thorin E, Ferbeyre G, Nattel S. The role of cellular senescence in cardiac disease: basic biology and clinical relevance. Nat Rev Cardiol. 2022;19:250-64.
73. Coppé JP, Patil CK, Rodier F, et al. Senescence-associated secretory phenotypes reveal cell-nonautonomous functions of oncogenic RAS and the p53 tumor suppressor. PLoS Biol. 2008;6:2853-68.
74. Xu M, Pirtskhalava T, Farr JN, et al. Senolytics improve physical function and increase lifespan in old age. Nat Med. 2018;24:1246-56.
75. Baker DJ, Childs BG, Durik M, et al. Naturally occurring p16Ink4a-positive cells shorten healthy lifespan. Nature. 2016;530:184-9.
76. Childs BG, Baker DJ, Wijshake T, Conover CA, Campisi J, van Deursen JM. Senescent intimal foam cells are deleterious at all stages of atherosclerosis. Science. 2016;354:472-7.
77. Kovacic JC, Moreno P, Nabel EG, Hachinski V, Fuster V. Cellular senescence, vascular disease, and aging: part 2 of a 2-part review: clinical vascular disease in the elderly. Circulation. 2011;123:1900-10.
78. Lewis-McDougall FC, Ruchaya PJ, Domenjo-Vila E, et al. Aged-senescent cells contribute to impaired heart regeneration. Aging Cell. 2019;18:e12931.
79. Lyu G, Guan Y, Zhang C, et al. TGF-β signaling alters H4K20me3 status via miR-29 and contributes to cellular senescence and cardiac aging. Nat Commun. 2018;9:2560.
80. Li T, Meng Y, Ding P, et al. Pathological implication of CaMKII in NF-κB pathway and SASP during cardiomyocytes senescence. Mech Ageing Dev. 2023;209:111758.
81. Gevaert AB, Shakeri H, Leloup AJ, et al. Endothelial senescence contributes to heart failure with preserved ejection fraction in an aging mouse model. Circ Heart Fail. 2017;10:e003806.
82. Redgrave RE, Dookun E, Booth LK, et al. Senescent cardiomyocytes contribute to cardiac dysfunction following myocardial infarction. NPJ Aging. 2023;9:15.
83. Meyer K, Hodwin B, Ramanujam D, Engelhardt S, Sarikas A. Essential role for premature senescence of myofibroblasts in myocardial fibrosis. J Am Coll Cardiol. 2016;67:2018-28.
84. Roh JD, Castro C, Yu A, et al. Placental senescence pathophysiology is shared between peripartum cardiomyopathy and preeclampsia in mouse and human. Sci Transl Med. 2024;16:eadi0077.
85. Walaszczyk A, Dookun E, Redgrave R, et al. Pharmacological clearance of senescent cells improves survival and recovery in aged mice following acute myocardial infarction. Aging Cell. 2019;18:e12945.
86. Salerno N, Marino F, Scalise M, et al. Pharmacological clearance of senescent cells improves cardiac remodeling and function after myocardial infarction in female aged mice. Mech Ageing Dev. 2022;208:111740.
87. Jia K, Dai Y, Liu A, et al. Senolytic agent navitoclax inhibits angiotensin II-induced heart failure in mice. J Cardiovasc Pharmacol. 2020;76:452-60.
88. Lérida-Viso A, Estepa-Fernández A, Morellá-Aucejo Á, et al. Pharmacological senolysis reduces doxorubicin-induced cardiotoxicity and improves cardiac function in mice. Pharmacol Res. 2022;183:106356.
89. Kirkland JL, Tchkonia T. Senolytic drugs: from discovery to translation. J Intern Med. 2020;288:518-36.
90. Nambiar A, Kellogg D 3rd, Justice J, et al. Senolytics dasatinib and quercetin in idiopathic pulmonary fibrosis: results of a phase I, single-blind, single-center, randomized, placebo-controlled pilot trial on feasibility and tolerability. EBioMedicine. 2023;90:104481.
91. Gonzales MM, Garbarino VR, Kautz TF, et al. Senolytic therapy in mild Alzheimer's disease: a phase 1 feasibility trial. Nat Med. 2023;29:2481-8.
92. Lee E, Carreras-Gallo N, Lopez L, et al. Exploring the effects of dasatinib, quercetin, and fisetin on DNA methylation clocks: a longitudinal study on senolytic interventions. Aging. 2024;16:3088-106.
93. Barzilai N, Crandall JP, Kritchevsky SB, Espeland MA. Metformin as a tool to target aging. Cell Metab. 2016;23:1060-5.
94. Martin-Montalvo A, Mercken EM, Mitchell SJ, et al. Metformin improves healthspan and lifespan in mice. Nat Commun. 2013;4:2192.
95. Abdelgawad IY, Agostinucci K, Sadaf B, Grant MKO, Zordoky BN. Metformin mitigates SASP secretion and LPS-triggered hyper-inflammation in Doxorubicin-induced senescent endothelial cells. Front Aging. 2023;4:1170434.
96. Moiseeva O, Deschênes-Simard X, St-Germain E, et al. Metformin inhibits the senescence-associated secretory phenotype by interfering with IKK/NF-κB activation. Aging Cell. 2013;12:489-98.
97. Slater RE, Strom JG, Methawasin M, et al. Metformin improves diastolic function in an HFpEF-like mouse model by increasing titin compliance. J Gen Physiol. 2019;151:42-52.
98. Gundewar S, Calvert JW, Jha S, et al. Activation of AMP-activated protein kinase by metformin improves left ventricular function and survival in heart failure. Circ Res. 2009;104:403-11.
99. Crowley MJ, Diamantidis CJ, McDuffie JR, et al. Clinical outcomes of metformin use in populations with chronic kidney disease, congestive heart failure, or chronic liver disease: a systematic review. Ann Intern Med. 2017;166:191-200.
100. Halabi A, Sen J, Huynh Q, Marwick TH. Metformin treatment in heart failure with preserved ejection fraction: a systematic review and meta-regression analysis. Cardiovasc Diabetol. 2020;19:124.
101. Li JZ, Li YR. Cardiovascular protection by metformin: latest advances in basic and clinical research. Cardiology. 2023;148:374-84.
102. Chin DWL, Yoshizato T, Virding Culleton S, et al. Aged healthy mice acquire clonal hematopoiesis mutations. Blood. 2022;139:629-34.
103. Sano S, Oshima K, Wang Y, et al. Tet2-mediated clonal hematopoiesis accelerates heart failure through a mechanism involving the IL-1β/NLRP3 inflammasome. J Am Coll Cardiol. 2018;71:875-86.
104. Sano S, Wang Y, Yura Y, et al. JAK2V617F-mediated clonal hematopoiesis accelerates pathological remodeling in murine heart failure. JACC Basic Transl Sci. 2019;4:684-97.
105. Sano S, Oshima K, Wang Y, Katanasaka Y, Sano M, Walsh K. CRISPR-mediated gene editing to assess the roles of Tet2 and Dnmt3a in clonal hematopoiesis and cardiovascular disease. Circ Res. 2018;123:335-41.
106. Fuster JJ, MacLauchlan S, Zuriaga MA, et al. Clonal hematopoiesis associated with TET2 deficiency accelerates atherosclerosis development in mice. Science. 2017;355:842-7.
107. Cheng X, Zhao H, Wen X, Li G, Guo S, Zhang D. NLRP3-inflammasome inhibition by MCC950 attenuates cardiac and pulmonary artery remodelling in heart failure with preserved ejection fraction. Life Sci. 2023;333:122185.
108. Wang Y, Sano S, Yura Y, et al. Tet2-mediated clonal hematopoiesis in nonconditioned mice accelerates age-associated cardiac dysfunction. JCI Insight. 2020;5:135204.
109. Fuster JJ, Zuriaga MA, Zorita V, et al. TET2-loss-of-function-driven clonal hematopoiesis exacerbates experimental insulin resistance in aging and obesity. Cell Rep. 2020;33:108326.
110. Borlaug BA, Jensen MD, Kitzman DW, Lam CSP, Obokata M, Rider OJ. Obesity and heart failure with preserved ejection fraction: new insights and pathophysiological targets. Cardiovasc Res. 2023;118:3434-50.
111. Reyes Gaido OE, Pavlaki N, Granger JM, et al. An improved reporter identifies ruxolitinib as a potent and cardioprotective CaMKII inhibitor. Sci Transl Med. 2023;15:eabq7839.
112. Russell-Hallinan A, Neary R, Watson CJ, Baugh JA. Repurposing from oncology to cardiology: low-dose 5-azacytidine attenuates pathological cardiac remodeling in response to pressure overload injury. J Cardiovasc Pharmacol Ther. 2021;26:375-85.
113. Lin AE, Bapat AC, Xiao L, et al. Clonal hematopoiesis of indeterminate potential with loss of Tet2 enhances risk for atrial fibrillation through Nlrp3 inflammasome activation. Circulation. 2024;149:1419-34.
114. Hoisnard L, Lebrun-Vignes B, Maury S, et al. Adverse events associated with JAK inhibitors in 126,815 reports from the WHO pharmacovigilance database. Sci Rep. 2022;12:7140.
115. Hoisnard L, Pina Vegas L, Dray-Spira R, Weill A, Zureik M, Sbidian E. Risk of major adverse cardiovascular and venous thromboembolism events in patients with rheumatoid arthritis exposed to JAK inhibitors versus adalimumab: a nationwide cohort study. Ann Rheum Dis. 2023;82:182-8.
116. Naffakh N, Williams M, Patel P, et al. Incidence of cardiac events in patients with MDS or AML receiving azacitidine or decitabine within a large community health system. J Clin Oncol. 2023;41:16.
117. Ridker PM, Everett BM, Thuren T, et al. Antiinflammatory therapy with canakinumab for atherosclerotic disease. N Engl J Med. 2017;377:1119-31.
118. Everett BM, Cornel JH, Lainscak M, et al. Anti-inflammatory therapy with canakinumab for the prevention of hospitalization for heart failure. Circulation. 2019;139:1289-99.
119. Svensson EC, Madar A, Campbell CD, et al. TET2-driven clonal hematopoiesis and response to canakinumab: an exploratory analysis of the CANTOS randomized clinical trial. JAMA Cardiol. 2022;7:521-8.
120. Mahfooz K, Rana A, Palagati K, et al. Anakinra in heart failure: a systematic review and meta-analysis of randomized controlled trials. Med Sci. 2022;11:4.
121. Ferreira JP, Vasques-Nóvoa F, Ferrão D, et al. Fenofibrate and heart failure outcomes in patients with type 2 diabetes: analysis from ACCORD. Diabetes Care. 2022;45:1584-91.
122. Kanno K, Koseki M, Chang J, et al. Pemafibrate suppresses NLRP3 inflammasome activation in the liver and heart in a novel mouse model of steatohepatitis-related cardiomyopathy. Sci Rep. 2022;12:2996.
123. Zheng J, Haberland V, Baird D, et al. Phenome-wide mendelian randomization mapping the influence of the plasma proteome on complex diseases. Nat Genet. 2020;52:1122-31.
124. Sun BB, Chiou J, Traylor M, et al. Plasma proteomic associations with genetics and health in the UK Biobank. Nature. 2023;622:329-38.
125. Eldjarn GH, Ferkingstad E, Lund SH, et al. Large-scale plasma proteomics comparisons through genetics and disease associations. Nature. 2023;622:348-58.
126. Katz DH, Robbins JM, Deng S, et al. Proteomic profiling platforms head to head: leveraging genetics and clinical traits to compare aptamer- and antibody-based methods. Sci Adv. 2022;8:eabm5164.
127. Menni C, Kiddle SJ, Mangino M, et al. Circulating proteomic signatures of chronological age. J Gerontol A Biol Sci Med Sci. 2015;70:809-16.
128. Tanaka T, Biancotto A, Moaddel R, et al. Plasma proteomic signature of age in healthy humans. Aging Cell. 2018;17:e12799.
129. Lehallier B, Gate D, Schaum N, et al. Undulating changes in human plasma proteome profiles across the lifespan. Nat Med. 2019;25:1843-50.
130. Sathyan S, Ayers E, Gao T, et al. Plasma proteomic profile of age, health span, and all-cause mortality in older adults. Aging Cell. 2020;19:e13250.
131. Orwoll ES, Wiedrick J, Nielson CM, et al. Proteomic assessment of serum biomarkers of longevity in older men. Aging Cell. 2020;19:e13253.
132. Liu X, Pan S, Xanthakis V, et al. Plasma proteomic signature of decline in gait speed and grip strength. Aging Cell. 2022;21:e13736.
133. Liu X, Axelsson GT, Newman AB, et al. Plasma proteomic signature of human longevity. Aging Cell. 2024;23:e14136.
134. Liu F, Austin TR, Schrack JA, et al. Late-life plasma proteins associated with prevalent and incident frailty: a proteomic analysis. Aging Cell. 2023;22:e13975.
135. Kuo CL, Chen Z, Liu P, et al. Proteomic aging clock (PAC) predicts age-related outcomes in middle-aged and older adults. Aging Cell. 2024;23:e14195.
136. Schaum N, Lehallier B, Hahn O, et al. Ageing hallmarks exhibit organ-specific temporal signatures. Nature. 2020;583:596-602.
137. Oh HS, Rutledge J, Nachun D, et al. Organ aging signatures in the plasma proteome track health and disease. Nature. 2023;624:164-72.
138. Basisty N, Kale A, Jeon OH, et al. A proteomic atlas of senescence-associated secretomes for aging biomarker development. PLoS Biol. 2020;18:e3000599.
139. Roh JD, Kitchen RR, Guseh JS, et al. Plasma proteomics of COVID-19-associated cardiovascular complications: implications for pathophysiology and therapeutics. JACC Basic Transl Sci. 2022;7:425-41.
140. Ferreira JP, Verdonschot J, Collier T, et al. Proteomic bioprofiles and mechanistic pathways of progression to heart failure. Circ Heart Fail. 2019;12:e005897.
141. Nayor M, Short MI, Rasheed H, et al. Aptamer-based proteomic platform identifies novel protein predictors of incident heart failure and echocardiographic traits. Circ Heart Fail. 2020;13:e006749.
142. Girerd N, Levy D, Duarte K, et al. Protein biomarkers of new-onset heart failure: insights from the heart omics and ageing cohort, the atherosclerosis risk in communities study, and the framingham heart study. Circ Heart Fail. 2023;16:e009694.
143. Emilsson V, Jonsson BG, Austin TR, et al. Proteomic prediction of incident heart failure and its main subtypes. Eur J Heart Fail. 2024;26:87-102.
144. Shah AM, Myhre PL, Arthur V, et al. Large scale plasma proteomics identifies novel proteins and protein networks associated with heart failure development. Nat Commun. 2024;15:528.
145. Ramonfaur D, Buckley LF, Arthur V, et al. High throughput plasma proteomics and risk of heart failure and frailty in late life. JAMA Cardiol. 2024;9:649-58.
146. Morikawa M, Derynck R, Miyazono K. TGF-β and the TGF-β family: context-dependent roles in cell and tissue physiology. Cold Spring Harb Perspect Biol. 2016;8:a021873.
147. Chang C. Agonists and antagonists of TGF-β family ligands. Cold Spring Harb Perspect Biol. 2016;8:a021923.
148. Acosta JC, Banito A, Wuestefeld T, et al. A complex secretory program orchestrated by the inflammasome controls paracrine senescence. Nat Cell Biol. 2013;15:978-90.
149. Loffredo FS, Steinhauser ML, Jay SM, et al. Growth differentiation factor 11 is a circulating factor that reverses age-related cardiac hypertrophy. Cell. 2013;153:828-39.
150. Sinha M, Jang YC, Oh J, et al. Restoring systemic GDF11 levels reverses age-related dysfunction in mouse skeletal muscle. Science. 2014;344:649-52.
151. Katsimpardi L, Litterman NK, Schein PA, et al. Vascular and neurogenic rejuvenation of the aging mouse brain by young systemic factors. Science. 2014;344:630-4.
152. Egerman MA, Cadena SM, Gilbert JA, et al. GDF11 increases with age and inhibits skeletal muscle regeneration. Cell Metab. 2015;22:164-74.
153. Schafer MJ, Atkinson EJ, Vanderboom PM, et al. Quantification of GDF11 and myostatin in human aging and cardiovascular disease. Cell Metab. 2016;23:1207-15.
154. Smith SC, Zhang X, Zhang X, et al. GDF11 does not rescue aging-related pathological hypertrophy. Circ Res. 2015;117:926-32.
155. Harper SC, Johnson J, Borghetti G, et al. GDF11 decreases pressure overload-induced hypertrophy, but can cause severe cachexia and premature death. Circ Res. 2018;123:1220-31.
156. Kraler S, Balbi C, Vdovenko D, et al. Circulating GDF11 exacerbates myocardial injury in mice and associates with increased infarct size in humans. Cardiovasc Res. 2023;119:2729-42.
157. Zimmers TA, Jiang Y, Wang M, et al. Exogenous GDF11 induces cardiac and skeletal muscle dysfunction and wasting. Basic Res Cardiol. 2017;112:48.
158. Chen L, Luo G, Liu Y, et al. Growth differentiation factor 11 attenuates cardiac ischemia reperfusion injury via enhancing mitochondrial biogenesis and telomerase activity. Cell Death Dis. 2021;12:665.
159. Zhu J, Zhang N, Zhao Y, et al. Deficiency of GDF-11 accelerates TAC-induced heart failure by impairing cardiac angiogenesis. JACC Basic Transl Sci. 2023;8:617-35.
160. Kizer JR, Patel S, Ganz P, et al. Circulating growth differentiation factors 11 and 8, their antagonists follistatin and follistatin-like-3, and risk of heart failure in elders. J Gerontol A Biol Sci Med Sci. 2024;79:glad206.
161. Roh JD, Hobson R, Chaudhari V, et al. Activin type II receptor signaling in cardiac aging and heart failure. Sci Transl Med. 2019;11:eaau8680.
162. Baccarelli A, Morpurgo PS, Corsi A, et al. Activin A serum levels and aging of the pituitary-gonadal axis: a cross-sectional study in middle-aged and elderly healthy subjects. Exp Gerontol. 2001;36:1403-12.
163. Chang HC, Wang X, Gu X, et al. Correlation of serum VEGF-C, ANGPTL4, and activin A levels with frailty. Exp Gerontol. 2024;185:112345.
164. Clavere NG, Alqallaf A, Rostron KA, et al. Inhibition of activin A receptor signalling attenuates age-related pathological cardiac remodelling. Dis Model Mech. 2022;15:dmm049424.
165. Yndestad A, Ueland T, Øie E, et al. Elevated levels of activin A in heart failure: potential role in myocardial remodeling. Circulation. 2004;109:1379-85.
166. Castillero E, Akashi H, Najjar M, et al. Activin type II receptor ligand signaling inhibition after experimental ischemic heart failure attenuates cardiac remodeling and prevents fibrosis. Am J Physiol Heart Circ Physiol. 2020;318:H378-90.
167. Zhou X, Wang JL, Lu J, et al. Reversal of cancer cachexia and muscle wasting by ActRIIB antagonism leads to prolonged survival. Cell. 2010;142:531-43.
168. Fukushima N, Matsuura K, Akazawa H, et al. A crucial role of activin A-mediated growth hormone suppression in mouse and human heart failure. PLoS One. 2011;6:e27901.
169. MacDonnell S, Megna J, Ruan Q, et al. Activin A directly impairs human cardiomyocyte contractile function indicating a potential role in heart failure development. Front Cardiovasc Med. 2022;9:1038114.
170. Oshima Y, Ouchi N, Shimano M, et al. Activin A and follistatin-like 3 determine the susceptibility of heart to ischemic injury. Circulation. 2009;120:1606-15.
171. Yung LM, Yang P, Joshi S, et al. ACTRIIA-Fc rebalances activin/GDF versus BMP signaling in pulmonary hypertension. Sci Transl Med. 2020;12:eaaz5660.
172. Hoeper MM, Badesch DB, Ghofrani HA, et al. Phase 3 trial of sotatercept for treatment of pulmonary arterial hypertension. N Engl J Med. 2023;388:1478-90.
173. Joshi SR, Liu J, Bloom T, et al. Sotatercept analog suppresses inflammation to reverse experimental pulmonary arterial hypertension. Sci Rep. 2022;12:7803.
174. Nunn E, Jaiswal N, Gavin M, et al. Antibody blockade of activin type II receptors preserves skeletal muscle mass and enhances fat loss during GLP-1 receptor agonism. Mol Metab. 2024;80:101880.
175. Swan J, Szabó Z, Peters J, et al. Inhibition of activin receptor 2 signalling ameliorates metabolic dysfunction-associated steatotic liver disease in western diet/L-NAME induced cardiometabolic disease. Biomed Pharmacother. 2024;175:116683.
176. Mullican SE, Lin-Schmidt X, Chin CN, et al. GFRAL is the receptor for GDF15 and the ligand promotes weight loss in mice and nonhuman primates. Nat Med. 2017;23:1150-7.
177. Rothenbacher D, Dallmeier D, Christow H, Koenig W, Denkinger M, Klenk J. Association of growth differentiation factor 15 with other key biomarkers, functional parameters and mortality in community-dwelling older adults. Age Ageing. 2019;48:541-6.
178. Daniels LB, Clopton P, Laughlin GA, Maisel AS, Barrett-Connor E. Growth-differentiation factor-15 is a robust, independent predictor of 11-year mortality risk in community-dwelling older adults: the Rancho Bernardo Study. Circulation. 2011;123:2101-10.
179. Wesseling M, de Poel JHC, de Jager SCA. Growth differentiation factor 15 in adverse cardiac remodelling: from biomarker to causal player. ESC Heart Fail. 2020;7:1488-501.
180. Conte M, Giuliani C, Chiariello A, Iannuzzi V, Franceschi C, Salvioli S. GDF15, an emerging key player in human aging. Ageing Res Rev. 2022;75:101569.
181. Moon JS, Goeminne LJE, Kim JT, et al. Growth differentiation factor 15 protects against the aging-mediated systemic inflammatory response in humans and mice. Aging Cell. 2020;19:e13195.
182. Wang X, Chrysovergis K, Kosak J, et al. hNAG-1 increases lifespan by regulating energy metabolism and insulin/IGF-1/mTOR signaling. Aging. 2014;6:690-704.
183. Park H, Kim CH, Jeong JH, Park M, Kim KS. GDF15 contributes to radiation-induced senescence through the ROS-mediated p16 pathway in human endothelial cells. Oncotarget. 2016;7:9634-44.
184. Mazagova M, Buikema H, Landheer SW, et al. Growth differentiation factor 15 impairs aortic contractile and relaxing function through altered caveolar signaling of the endothelium. Am J Physiol Heart Circ Physiol. 2013;304:H709-18.
185. Guo H, Zhao X, Li H, et al. GDF15 promotes cardiac fibrosis and proliferation of cardiac fibroblasts via the MAPK/ERK1/2 pathway after irradiation in rats. Radiat Res. 2021;196:183-91.
186. Abulizi P, Loganathan N, Zhao D, et al. Growth differentiation factor-15 deficiency augments inflammatory response and exacerbates septic heart and renal injury induced by lipopolysaccharide. Sci Rep. 2017;7:1037.
187. Xu J, Kimball TR, Lorenz JN, et al. GDF15/MIC-1 functions as a protective and antihypertrophic factor released from the myocardium in association with SMAD protein activation. Circ Res. 2006;98:342-50.
188. Dogon G, Rigal E, Potel E, et al. Growth/differentiation factor 15 (GDF15) expression in the heart after myocardial infarction and cardioprotective effect of pre-ischemic rGDF15 administration. Sci Rep. 2024;14:12949.
189. Takaoka M, Tadross JA, Al-Hadithi A, et al. GDF15 antagonism limits severe heart failure and prevents cardiac cachexia in mice. bioRxiv. 2022; doi: 10.1101/2022.09.06.506633.
190. Khetarpal SA, Li H, Vitale T, et al. Cardiomyocyte PGC-1α enables physiological adaptations to endurance exercise through suppression of GDF15 and cardiac atrophy. bioRxiv. 2024; doi: 10.1101/2024.01.30.578093.
191. Kim-Muller JY, Song L, LaCarubba Paulhus B, et al. GDF15 neutralization restores muscle function and physical performance in a mouse model of cancer cachexia. Cell Rep. 2023;42:111947.
192. Albuquerque B, Chen X, Hirenallur-Shanthappa D, et al. Neutralization of GDF15 prevents anorexia and weight loss in the monocrotaline-induced cardiac cachexia rat model. Cells. 2022;11:1073.
193. Benichou O, Coskun T, Gonciarz MD, et al. Discovery, development, and clinical proof of mechanism of LY3463251, a long-acting GDF15 receptor agonist. Cell Metab. 2023;35:274-86.e10.
194. Sanchez-Soria P, Camenisch TD. ErbB signaling in cardiac development and disease. Semin Cell Dev Biol. 2010;21:929-35.
195. Lemmens K, Doggen K, De Keulenaer GW. Role of neuregulin-1/ErbB signaling in cardiovascular physiology and disease: implications for therapy of heart failure. Circulation. 2007;116:954-60.
196. Baliga RR, Pimental DR, Zhao YY, et al. NRG-1-induced cardiomyocyte hypertrophy. role of PI-3-kinase, p70(S6K), and MEK-MAPK-RSK. Am J Physiol. 1999;277:H2026-37.
197. Bersell K, Arab S, Haring B, Kühn B. Neuregulin1/ErbB4 signaling induces cardiomyocyte proliferation and repair of heart injury. Cell. 2009;138:257-70.
198. Haskins JW, Nguyen DX, Stern DF. Neuregulin 1-activated ERBB4 interacts with YAP to induce Hippo pathway target genes and promote cell migration. Sci Signal. 2014;7:ra116.
199. Gassmann M, Casagranda F, Orioli D, et al. Aberrant neural and cardiac development in mice lacking the ErbB4 neuregulin receptor. Nature. 1995;378:390-4.
200. Vermeulen Z, Hervent AS, Dugaucquier L, et al. Inhibitory actions of the NRG-1/ErbB4 pathway in macrophages during tissue fibrosis in the heart, skin, and lung. Am J Physiol Heart Circ Physiol. 2017;313:H934-45.
201. Rentschler S, Zander J, Meyers K, et al. Neuregulin-1 promotes formation of the murine cardiac conduction system. Proc Natl Acad Sci USA. 2002;99:10464-9.
202. Okoshi K, Nakayama M, Yan X, et al. Neuregulins regulate cardiac parasympathetic activity: muscarinic modulation of beta-adrenergic activity in myocytes from mice with neuregulin-1 gene deletion. Circulation. 2004;110:713-7.
203. Shiraishi M, Yamaguchi A, Suzuki K. Nrg1/ErbB signaling-mediated regulation of fibrosis after myocardial infarction. FASEB J. 2022;36:e22150.
204. Lemmens K, Segers VF, Demolder M, De Keulenaer GW. Role of neuregulin-1/ErbB2 signaling in endothelium-cardiomyocyte cross-talk. J Biol Chem. 2006;281:19469-77.
205. Edrey YH, Casper D, Huchon D, et al. Sustained high levels of neuregulin-1 in the longest-lived rodents; a key determinant of rodent longevity. Aging Cell. 2012;11:213-22.
206. Shakeri H, Gevaert AB, Schrijvers DM, et al. Neuregulin-1 attenuates stress-induced vascular senescence. Cardiovasc Res. 2018;114:1041-51.
207. Rohrbach S, Niemann B, Abushouk AM, Holtz J. Caloric restriction and mitochondrial function in the ageing myocardium. Exp Gerontol. 2006;41:525-31.
208. Lemmens K, Doggen K, De Keulenaer GW. Activation of the neuregulin/ErbB system during physiological ventricular remodeling in pregnancy. Am J Physiol Heart Circ Physiol. 2011;300:H931-42.
209. Cai MX, Shi XC, Chen T, et al. Exercise training activates neuregulin 1/ErbB signaling and promotes cardiac repair in a rat myocardial infarction model. Life Sci. 2016;149:1-9.
210. Keulenaer GW, Doggen K, Lemmens K. The vulnerability of the heart as a pluricellular paracrine organ: lessons from unexpected triggers of heart failure in targeted ErbB2 anticancer therapy. Circ Res. 2010;106:35-46.
211. Slamon DJ, Leyland-Jones B, Shak S, et al. Use of chemotherapy plus a monoclonal antibody against HER2 for metastatic breast cancer that overexpresses HER2. N Engl J Med. 2001;344:783-92.
212. Ozcelik C, Erdmann B, Pilz B, et al. Conditional mutation of the ErbB2 (HER2) receptor in cardiomyocytes leads to dilated cardiomyopathy. Proc Natl Acad Sci USA. 2002;99:8880-5.
213. Crone SA, Zhao YY, Fan L, et al. ErbB2 is essential in the prevention of dilated cardiomyopathy. Nat Med. 2002;8:459-65.
214. García-Rivello H, Taranda J, Said M, et al. Dilated cardiomyopathy in Erb-b4-deficient ventricular muscle. Am J Physiol Heart Circ Physiol. 2005;289:H1153-60.
215. Rohrbach S, Niemann B, Silber RE, Holtz J. Neuregulin receptors erbB2 and erbB4 in failing human myocardium - depressed expression and attenuated activation. Basic Res Cardiol. 2005;100:240-9.
216. Ky B, Kimmel SE, Safa RN, et al. Neuregulin-1 beta is associated with disease severity and adverse outcomes in chronic heart failure. Circulation. 2009;120:310-7.
217. Liu X, Gu X, Li Z, et al. Neuregulin-1/erbB-activation improves cardiac function and survival in models of ischemic, dilated, and viral cardiomyopathy. J Am Coll Cardiol. 2006;48:1438-47.
218. Wang Y, Wei J, Zhang P, et al. Neuregulin-1, a potential therapeutic target for cardiac repair. Front Pharmacol. 2022;13:945206.
219. Gu X, Liu X, Xu D, et al. Cardiac functional improvement in rats with myocardial infarction by up-regulating cardiac myosin light chain kinase with neuregulin. Cardiovasc Res. 2010;88:334-43.
220. Gao R, Zhang J, Cheng L, et al. A Phase II, randomized, double-blind, multicenter, based on standard therapy, placebo-controlled study of the efficacy and safety of recombinant human neuregulin-1 in patients with chronic heart failure. J Am Coll Cardiol. 2010;55:1907-14.
221. Jabbour A, Hayward CS, Keogh AM, et al. Parenteral administration of recombinant human neuregulin-1 to patients with stable chronic heart failure produces favourable acute and chronic haemodynamic responses. Eur J Heart Fail. 2011;13:83-92.
222. Lenihan DJ, Anderson SA, Lenneman CG, et al. A phase I, single ascending dose study of Cimaglermin alfa (Neuregulin 1β3) in patients with systolic dysfunction and heart failure. JACC Basic Transl Sci. 2016;1:576-86.
223. Hill MF, Patel AV, Murphy A, et al. Intravenous glial growth factor 2 (GGF2) isoform of neuregulin-1β improves left ventricular function, gene and protein expression in rats after myocardial infarction. PLoS One. 2013;8:e55741.
224. Galindo CL, Kasasbeh E, Murphy A, et al. Anti-remodeling and anti-fibrotic effects of the neuregulin-1β glial growth factor 2 in a large animal model of heart failure. J Am Heart Assoc. 2014;3:e000773.
225. Tang WH, Steiner J, Kassi M, et al. Final analysis of safety and exploratory echo data in a phase 1, first-in-human, randomized, double-blind, placebo-controlled, single ascending dose study to evaluate JK07 in subjects with heart failure with reduced ejection fraction. J Card Fail. 2024;30:314.
226. Murphy SL, Tang WHW, Zhuang X, Li J. Abstract 18358: preclinical evaluation of the safety and activity of JK07, a novel bifunctional neuregulin antibody fusion which selectively activates ErbB4, for the treatment of heart failure. Circulation. 2023;148:A18358.
227. Pilling LC, Kuo CL, Sicinski K, et al. Human longevity: 25 genetic loci associated in 389,166 UK biobank participants. Aging. 2017;9:2504-20.
228. Joshi PK, Pirastu N, Kentistou KA, et al. Genome-wide meta-analysis associates HLA-DQA1/DRB1 and LPA and lifestyle factors with human longevity. Nat Commun. 2017;8:910.
229. Wright KM, Rand KA, Kermany A, et al. A prospective analysis of genetic variants associated with human lifespan. G3. 2019;9:2863-78.
230. Timmers PR, Mounier N, Lall K, et al. Genomics of 1 million parent lifespans implicates novel pathways and common diseases and distinguishes survival chances. Elife. 2019;8:e39856.
231. Timmers PRHJ, Wilson JF, Joshi PK, Deelen J. Multivariate genomic scan implicates novel loci and haem metabolism in human ageing. Nat Commun. 2020;11:3570.
Cite This Article
How to Cite
Download Citation
Export Citation File:
Type of Import
Tips on Downloading Citation
Citation Manager File Format
Type of Import
Direct Import: When the Direct Import option is selected (the default state), a dialogue box will give you the option to Save or Open the downloaded citation data. Choosing Open will either launch your citation manager or give you a choice of applications with which to use the metadata. The Save option saves the file locally for later use.
Indirect Import: When the Indirect Import option is selected, the metadata is displayed and may be copied and pasted as needed.
About This Article
Copyright
Data & Comments
Data
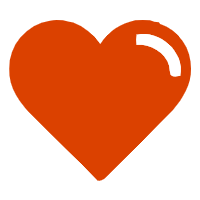
Comments
Comments must be written in English. Spam, offensive content, impersonation, and private information will not be permitted. If any comment is reported and identified as inappropriate content by OAE staff, the comment will be removed without notice. If you have any queries or need any help, please contact us at [email protected].