Can age be a modifiable risk factor? the impact of dietary patterns on the molecular mechanisms that underlie cardiovascular aging
Abstract
Aging is the number one risk factor for the development of cardiovascular disease (CVD). Therefore, an evaluation of therapies for the prevention of CVD should focus on factors that slow down aging, particularly cardiovascular aging. There are various proposed mechanisms that advance cardiovascular age; in this review, we focus on chronic inflammation, oxidative stress and epigenetics as the primary drivers of aging. Furthermore, we will evaluate several dietary patterns on their impact on these aging mechanisms. The traditional "heart-healthy" dietary patterns such as the Mediterranean diet, plant-based diet and intermittent fasting will be evaluated for their performance to slow down the aforementioned aging mechanisms. The aim of this review will be to guide practitioners and patients on the dietary components that can slow down the effects of aging to prevent CVD.
Keywords
INTRODUCTION
Aging can be characterized by a time-related decline of physiological function required for survival and/or reproduction[1]. Importantly, biological aging is intricately linked to the manifestation and sequelae of cardiovascular disease (CVD), as age is the strongest determinant of CVD among known risk factors[2,3]. In less than one decade, one in every five individuals will be at least 65 years of age and contribute to nearly one half of all CVD events, while access to cost-effective preventive treatments and risk stratification tools may remain a public health challenge[4,5]. Correspondingly, a suboptimal diet is the number one leading cause of morbidity and mortality from noncommunicable diseases in the United States and worldwide[6]. Thus, the continued promotion of a healthy diet to prevent the manifestation and sequelae of CVD should be a priority to support optimal cardiovascular health throughout the life course.
There are many mechanisms by which the combination of a suboptimal diet and age can adversely influence the cardiovascular system, most notably resulting in the development of atherosclerosis, decreased arterial compliance, and diastolic dysfunction, all of which are associated with and/or may increase the risk for myocardial infarction, stroke, and heart failure. For example, high adherence to a vegetarian diet is associated with a 16% lower risk of incident CVD, 6 mmHg lower systolic blood pressure, and 77% lower risk of diastolic dysfunction[7-9]. Many different mechanisms of aging are likely contributors to the interplay of diet and CVD, including chronic inflammation, mitochondrial dysfunction, oxidative stress, and epigenetics[10,11]. All of these latter mechanisms may underly the pathophysiological connection of risk factors, such as dyslipidemia, hypertension, insulin resistance, and endothelial dysfunction, with CVD events and mortality[10].
Overall, the delay of cellular processes associated with aging and of the development of risk factors through dietary approaches is a foundation for the prevention of CVD [Figure 1]. Many dietary patterns, including the Mediterranean, dietary approaches to stop hypertension (DASH), vegetarian, and vegan, as well as intermittent fasting, can be exceedingly beneficial to uniformly reduce CVD risk regardless of age, sex, and/or ethnicity[12-14]. In the present review, we summarize the recent evidence regarding the interplay of dietary patterns, aging, and CVD risk. Through this process, we hope to highlight the importance of an optimal diet for the promotion of cardiovascular health, across the cellular, clinical, and public health spectrums.
CHRONIC INFLAMMATION
The relationship between inflammation and cardiovascular disease is complex. There are several proposed mechanisms at the cellular and molecular level that contribute to immune cell dysregulation, vascular remodeling as well as endothelial and myocyte dysfunction [Figure 2]. These cellular mechanisms often present as inflammatory phenotypes, including dyslipidemia, obesity, insulin resistance, and diabetes, which contribute to hypertension, atherosclerosis, left ventricular dysfunction, and cardiovascular events such as myocardial infarction or stroke.
Inflammation overview
The immune dysfunction associated with aging and inflammaging refers to a chronic state of inflammation with elevated levels of phagocytic cells and increased secretion of inflammatory markers such as IL-1, IL-6, IL-8, IL-13, IL-18, TNF-α, TNF-β, and CRP [Figure 3]. The specific stimuli responsible for this heightened level of inflammation are variable but can be grossly categorized as external and internal stressors that one experiences throughout life[15]. External stressors include environmental exposures such as viral or bacterial pathogens, particle pollution, physical activity, mental health, and dietary patterns[15,16]. Internal stimuli refer to cell products resulting from cell turnover, cell damage, and metabolic dysfunction[15]. Dietary patterns serve as a bridge between internal and external factors, as dietary habits contribute to metabolic dysfunction and lead to comorbidities such as dyslipidemia, insulin resistance, hypertension, and obesity that lead to a chronic inflammatory state[17].
Cell turnover/damage
Cellular aging occurs when turnover processes, such as autophagy and apoptosis, are disrupted, leading to misfolded proteins and other cellular debris that activate signaling pathways, including damage-associated molecular patterns (DAMPs)[16]. This leads to degradation by phagocytes which secrete cytokines and chemokines that recruit other lymphocytes, driving a chronic inflammatory response[18,19]. This inflammatory response is directly related to cellular senescence and the senescence-associated secretory phenotype (SASP), which is a state of cell cycle arrest due to mechanisms such as telomere shortening, DNA damage, epigenetic modifications, and exposure to DAMPs. SASP is associated with atherosclerosis and heart failure[15,16,20].
Senescence/SASP
SASP is a cellular mechanism that underscores the connection between microbiology and inflammatory factors and cardiovascular disease, as it is directly associated with hypertension and atherosclerosis. Senescence within endothelial cells, vascular smooth muscle cells, macrophages, and T-cells leads to overexpression of cell cycle checkpoint proteins such as p16INK4A, p53, p21, and senescence-associated
Matrix metalloproteinases degrade the intercellular and extracellular matrix by cleaving proteins that regulate structure and cell differentiation. This leads to cellular aging via intimal thickening and arterial stiffening, resulting in hypertension and atherosclerosis. MMPs can further increase arterial fibrosis and atherosclerosis by activating TGF-b15 while reducing the expression of vasodilators[29].
Other proinflammatory markers associated with SASP increase platelet reactivity and plaque instability[29]. Senescent cells within atherosclerotic plaque secrete chemokines including MMP-12, MMP-13, and IL-1a, which cause degradation within the plaque while activating neighboring immune cells, increasing inflammation and promoting further atherosclerosis[24-26]. Given the significant impact of senescence and the SASP on hypertension and atherosclerosis, studies such as the CANTOS trial have assessed the benefit of using medical therapy to specifically target inflammatory pathways[16].
Inflammatory phenotypes
Hyperlipidemia
Inflammatory cytokines are associated with common disease states that contribute to cardiovascular inflammation and cellular aging. Hypercholesterolemia, specifically elevated levels of low-density lipoproteins (LDL), creates a chronic inflammatory response that leads to atherosclerosis. When excess LDL cholesterol circulates, it accumulates in the sub-endothelial layer of the arterial wall and becomes oxidized, adhering to the endothelial cells. This leads to the recruitment of leukocytes and macrophages that invade endothelial cells via ICAM-1 and VCAM-1. Macrophages then phagocytize oxidized LDL cholesterol and are transformed into foam cells which secrete IL-1, IL-6, TNF-a, and other chemokines that upregulate CRP[18,19,30,31]. This inflammation ultimately creates a cycle in which vascular smooth muscle cells proliferate, along with further lipid and foam cell accumulation, forming atherosclerotic plaque[19].
Obesity
The mechanism for chronic inflammation due to obesity is primarily driven by excess adipocytes. Adipose tissue produces inflammatory adipokines such as leptin, fatty acid-binding protein 4, and resistin, which have been associated with cardiac hypertrophy, fibrosis, and atherosclerosis[32]. Adipocytes can also secrete IL-1b, IL-6, IFN-, and monocyte chemoattractant protein (MCP-1) which lead to recruitment of local and systemic immune cells. These cells secrete molecules such as TNF-a, which contribute to systemic inflammation and impede insulin signaling pathways, leading to insulin resistance[16,32-34].
When storage of this adipose tissue becomes dysregulated, hyperplasia and hypertrophy occur. This leads to apoptosis and infiltration by macrophages, neutrophils, and lymphocytes which secrete IL-1, IL-6, and TNF-a, contributing to upregulation of CRP[32,35]. This impaired adipogenesis leads to increased circulation of free fatty acids and excess deposition of lipids which can negatively affect cardiac myocytes by altering the structure and metabolic function of these cells, a concept known as lipotoxicity[33,36]. Excess free fatty acids can contribute to systemic inflammation and impair insulin signal pathways, contributing to insulin resistance[33,35].
Diabetes & insulin resistance
Insulin resistance and diabetes are closely associated with obesity, contributing to proinflammatory states. Hyperglycemia is classically associated with elevated levels of advanced glycation end products that accumulate within blood vessels and contribute to atherosclerotic disease. Insulin resistance has been associated with higher levels of prothrombotic molecules as well as an increase in ICAM-1 and VCAM-1, suggesting it also increases the risk of thrombosis and atherosclerosis[36].
Insulin resistance further contributes to hypertension by decreasing the release of nitric oxide from endothelial cells and increasing smooth muscle proliferation[36]. Insulin resistance also decreases the activity of lipoprotein lipase and leads to an increase in free fatty acids as well as inflammatory molecules such as
Dietary patterns and inflammation
Recent studies have focused on the impact of different dietary patterns on inflammation as well as cardiovascular outcomes. The following discussion will review the effect of the Mediterranean dietary pattern, plant-based dietary patterns, as well as intermittent fasting on inflammatory factors, proinflammatory conditions, and cardiovascular disease [Table 1].
Primary effects of dietary patterns on inflammation
Diet | Mediterranean diet | Plant-based dietary pattern | Intermittent fasting |
Primary effect on inflammatory markers | ↓CRP, IL-8, IL-6, TNF-a, MCP-1 | ↓CRP | ↓CRP, IL-6 |
Proposed mechanisms | ● High in anti-inflammatory polyphenols and long-chain fatty acids ● Direct attenuation of immune cell pathways ● Anti - platelet effects ● Alteration of GI flora | ● High in anti-inflammatory nutrients, polyphenols, and long-chain fatty acids ● Decreased exposure to proinflammatory metabolites of animal products | ● Highly effective for weight loss ● Increased release of adiponectin, with vasorelaxant effect and decreased adhesion of monocytes to endothelial cells ● Improved insulin sensitivity |
Limitations | ● Difficult to standardize across trials | ● Difficult to standardize across trials ● Can increase the risk of nutrient deficiency | ● Different temporal fasting patterns ● Lack of dietary guidance ● Long-term sustainability |
Mediterranean dietary pattern
The Mediterranean dietary pattern (MDP) consists of vegetables, fruits, low-fat dairy products, beans, nuts, olive oil, lean protein, and whole grains. In a recent meta-analysis, 13 studies between 2006 and 2019 involving more than 11,500 participants were analyzed to assess the effect of a MDP on inflammatory markers in adults[38]. The effect of dietary patterns on CRP, IL-6, TNF-a, IL-1b, IL-2, IL-18, IL-22, IL-23, MCP-1, ICAM-1, and VCAM-1 were evaluated. The results demonstrated a decrease in inflammatory markers associated with the MDP. There was strong evidence to suggest decreases in CRP (SMD = -0.26; 95%CI: -0.41, -0.11; P < 0.001) as well as a decrease in IL-6, TNF-a, IL-8, and MCP-1[38].
These benefits were attributed to the high consumption of monounsaturated fatty acids and polyphenols as well as high concentrations of long-chain n-3 fatty acids, which possess anti-inflammatory properties and can inhibit the expression of inflammatory molecules such as COX-2. Additionally, nuts and legumes have elevated levels of phytochemicals and dietary fiber, which can decrease CRP and other cytokines. A proposed mechanism for this reduction in inflammation is an antioxidant effect that suppresses the production of ROS and leads to decreased production of cytokines. Overall, this meta-analysis underscores the anti-inflammatory properties of the MDP by providing evidence of a decrease in CRP as well as other inflammatory molecules[38].
Another proposed mechanism for the beneficial impact of MDP on inflammation suggests direct attenuation of immune cell pathways by decreasing the level of circulating immune cells and modulating adhesion, reducing the risk of endothelial dysfunction[39]. This hypothesis was illustrated in a RCT that followed 106 individuals and noted significant decreases in proinflammatory factors such as CD40 and adhesion molecules such as ICAM-1 in the MDP group (P < 0.05)[40]. Another large study of 14,586 individuals cited a link between cardiovascular disease risk and white blood cell count as well as platelet count, which were inversely correlated with adherence to the MDP (P = 0.008 and P < 0.0001, respectively)[41]. Components of olive oil such as oleocanthal and alperujo may also decrease platelet adhesion, providing significant anti-inflammatory and cardioprotective properties[39].
It is further suggested that following a MDP can alter the microbiota of the gut, leading to lower levels of cholesterol and increased short-chain fatty acids that contribute to reduced cardiovascular risk[42,43]. There are ongoing investigations to determine the exact mechanisms by which dietary patterns such as MDP have a dynamic and beneficial effect on gastrointestinal flora composition. One proposed hypothesis suggests that MDP may select against bacteria that utilize simple sugars and oligosaccharides while promoting the growth of bacteria that utilize polyphenols, polysaccharides, and soluble fibers[42,44].
Given the reduction in inflammatory factors and risk of atherosclerosis, studies have further sought to quantify the effect of MDP on major adverse cardiac events (MACE). The PREDIMED trial noted a relative decrease in the risk of major cardiac events such as myocardial infarction, stroke, and death from heart disease by 30% in the MDP supplemented with olive oil or tree nuts when compared to the control group[12]. In another trial, 605 individuals who previously suffered a myocardial infarction consumed either MDP or a Western diet for 4 years. The group following a MDP had significantly fewer cardiac deaths, cardiovascular events, and hospitalizations[45]. Moreover, the REGARDS trial showed a trend toward lower risk of SCD in a large sample of individuals who followed a Mediterranean diet when compared to other dietary patterns[46]
This data is promising and suggests that the MDP decreases the level of inflammatory modulators that contribute to hypertension and atherosclerosis. The mechanisms for this anti-inflammatory effect are most likely due to increased consumption of polyphenols and long-chain fatty acids, direct attenuation of immune cell pathways, anti-platelet effects, and alteration of GI flora. The above studies underscore the culmination of this anti-inflammatory effect, with a decrease in molecules such as CRP, IL-6, TNF-a, IL-8, and MCP-1 that are associated with atherosclerotic disease. In addition to these microbiological effects, there is substantial evidence to support the benefits of the MDP on inflammatory conditions such as hypertension, hyperlipidemia, and diabetes mellitus, which can lead to improved cardiovascular outcomes[45-51].
Plant-based dietary patterns
The plant-based dietary pattern (PBP), which includes vegan and vegetarian diets, are nutrient-rich dietary patterns that have been shown to improve risk factors for cardiovascular disease[7]. There are ongoing investigations to determine the exact mechanisms by which this dietary pattern impacts inflammation and cardiovascular disease. Current theories suggest that PBP provides high levels of antioxidants, decreased inflammatory molecules, reduced cholesterol levels, and improved insulin sensitivity.
Recent studies assessed the relationship between PBP and inflammatory markers associated with cardiovascular aging. A cross-sectional study published in Nature sought to define the relationship between these markers and PBP[52]. A study in Nature evaluated 36 participants in the PBP group compared to 36 individuals in the omnivorous diet group, who were between the ages of 30 and 60 years without evidence of cancer, diabetes, or cardiovascular disease. There were no significant differences in CRP, ICAM-1, IL18, or IL-1 between the two groups in the short term. However, in “long-time” consumers of PBP, diet adherence for at least 4.8 years was associated with a decrease in the CRP level[52]. This was consistent with a prior review that demonstrated lower CRP concentrations in individuals who followed a PBP for at least two years[53]. A meta-analysis performed shortly after these studies reviewed 1073 publications and 21 cross-sectional studies to compare various inflammatory markers between individuals following a PBP and omnivorous diet. Results demonstrated lower levels of CRP in individuals following a PBP (-0.54 mg/L,
PBP can reduce inflammation via high levels of antioxidants; however, this dietary pattern also excludes harmful metabolic compounds from animal products. One example of a harmful molecule associated with animal products, specifically red meat, is trimethylamine N-oxide (TMAO), a derivative of carnitine. TMAO is associated with inflammatory cytokines and adhesion molecules, as well as atherosclerosis and heart failure. Prior studies documenting the cardioprotective benefit of a PBP have documented lower levels of TMAO production[55]. There are also studies suggesting that a typical Western diet high in carbohydrates and fats derived from animals can lead to inflammation via increased oxidative stress and reactive oxygen species (ROS)[55]. The increase in ROS and decrease in nitric oxide production over time can lead to a chronic inflammatory state that contributes to endothelial dysfunction, hypertension, and atherosclerosis[55]. In fact, many animal-derived food products incorporated into the Western diet have higher levels of catalytic molecules such as iron, myoglobin, and hemoglobin, which over time can lead to cholesterol accumulation in vascular smooth muscle via lipid peroxidation, contributing to atherosclerosis[55]. In contrast, PBP has high levels of phenol-type compounds, typically derived from fruits and vegetables, which can neutralize oxidative anions and lipid peroxides while also binding ions such as iron or copper, diminishing the oxidative effect of these compounds[55]. Flavonoids are one example of these compounds, decreasing the production of ROS while reducing the level of lipid hydroperoxides[55]. By decreasing the level of lipid oxidation, these compounds may decrease the risk of cardiovascular disease.
Other well-known antioxidants that are prominent in PBP are carotenoids and vitamin A, which protect the retina and optic nerve from ROS. Lycopene is a carotenoid that can increase the antioxidant property of HDL, decreasing the risk of stress to endothelial cells[55]. Vitamin C also acts as an anti-inflammatory molecule by donating electrons and neutralizing superoxide radicals as well as hydrogen peroxide. Studies have shown an association between vitamin C consumption and improved blood pressure and endothelial function[56,57].
PBP does appear to decrease levels of inflammatory molecules such as CRP. This is likely a result of increased consumption of anti-inflammatory nutrients as well as decreased exposure to inflammatory, catalytic molecules derived from processed carbohydrates and animal products that lead to increased inflammation and oxidate stress. This anti-inflammatory effect appears to be stronger over a long-term duration. However, there is a need for additional clinical studies to better understand the effect on other inflammatory cytokines.
Intermittent fasting
Intermittent fasting (IF) refers to a dietary pattern that involves consuming fewer calories on certain days of the week (alternate day fasting, ADF) or during specific times throughout the day (time-restricted eating, TRE). In ADF, individuals may consume fewer calories on some days, followed by more liberal consumption on other days. In TRE, individuals typically consume food during an 8, 12, or 16-h window, as this is believed to be metabolically advantageous[58].
There have been recent studies to investigate the effect of IF on inflammatory markers classically associated with atherosclerosis, such as CRP and IL-6. One study examined these inflammatory biomarkers in 40 participants who were fasting during Ramadan, from sunrise until sunset, and compared them to 28 non-fasting control participants. Blood draws were performed one week prior and 3 weeks after Ramadan. The results showed a significant decrease in IL-6 and CRP (P < 0.001)[59]. An RCT of 32 participants following ADF for 12 weeks demonstrated a 13%-17% reduction in CRP compared to controls[60]. A meta-analysis of 18 RCTs analyzing the reduction in CRP among IF and energy-restricted diets compared to controls also showed a significant reduction in the weighted mean difference, with IF being superior to an energy-restricted diet[61].
A relevant biomarker of IF is adiponectin, an anti-inflammatory plasma protein produced by adipocytes that has a cardioprotective effect by decreasing the adhesion of monocytes to endothelial cells[19]. One proposed mechanism for this is via inhibition of VCAM-1, endothelial-leukocyte adhesion molecule 1 (ELAM-1), and ICAM-1 on endothelial cells[19]. Adiponectin is also thought to have antioxidant and vasorelaxant qualities[62]. Low adiponectin levels have been observed in insulin resistance and atherosclerosis[19]. Two recent studies have shown significant increases in adiponectin associated with IF. One study followed 32 participants who were randomly assigned to ADF or a control group and noted an increase in plasma adiponectin (6 ± 10%, P < 0.01) along with a significant decrease in CRP (13 ± 17%,
Overall, intermittent fasting appears to reduce inflammation and oxidative stress, portending improvement in inflammatory phenotypes such as hypertension, obesity, insulin resistance, and hyperlipidemia. Despite this promising evidence, there remains a need for additional large RCTs to assess the long-term impact of IF on inflammation as it pertains to cardiovascular risk[65,66].
Intermediate endpoints
It is important to consider intermediate endpoints associated with the molecular mechanisms of cardiovascular aging. While there is limited data directly comparing the three dietary patterns discussed, there is literature available to assess the effects of these dietary patterns on arterial stiffness, blood pressure, and diastolic dysfunction.
There are several studies that demonstrate a decrease in arterial stiffness with higher compliance to MDP, PBP, or IF[67-69]. However, the design of the studies is heterogenous, making it difficult to create a direct comparison among the dietary patterns.
Similarly, there have been studies assessing the impact of these three dietary patterns on left ventricular systolic and diastolic function. One cross-sectional study showed that higher levels of adherence to the MDP can lead to improved LV structure and function compared to lower adherence[70]. A RCT completed during the PREDIMED trial revealed decreased levels of N-terminal pro-brain natriuretic peptide compared to low-fat diets, which may also suggest improvement in diastolic function[71]. A different cross-sectional study of 133 individuals over the age of 60 noted a lower prevalence of diastolic dysfunction in individuals who adhered to a PBP compared to a non-PBP[72]. There are limited studies on the effect of IF on left ventricular function; however, animal studies suggest it could improve systolic function and decrease left ventricular remodeling[73].
Meta-analyses have also revealed modest improvement in systolic and diastolic blood pressure in patients following a PBP, with one study noting an average decrease in systolic blood pressure of 2.66 mmHg and 1.69 mmHg decrease in diastolic blood pressure[74]. A comparative study showed a larger decrease in systolic and diastolic blood pressure in the Mediterranean diet group (-9.3 and -7.3 mmHg, respectively) compared to the PBP group (-3.4 and -4.1 mmHg)[75]. A separate study followed 1422 individuals who adhered to IF for one year and found that the average systolic blood pressure decreased from 131.6 mmHg to 120.7
This data suggests that all three dietary patterns may have a beneficial effect on these intermediate endpoints. It further suggests that MDP and IF may provide a larger decrease in blood pressure when compared to PBP. However, there are fewer data available directly comparing the effect of these dietary patterns on arterial stiffness and diastolic dysfunction. Moreover, the indices used to compare these two endpoints are variable, which further complicates a comparative analysis. This could provide a basis for future research trials, as more RCTs directly comparing the effect of these dietary patterns on arterial stiffness and diastolic dysfunction may provide valuable insight.
Conclusion
The MDP, PBP, and IF approaches all have favorable anti-inflammatory profiles. This is significant, as many of these inflammatory markers can be connected to inflammatory disease processes. The specific mechanisms by which these dietary patterns provide anti-inflammatory effects may be different and the amount of data available differs among the dietary regimens. There is also limited data that directly compare the anti-inflammatory effect among these dietary patterns. Although further studies should be performed to compare these dietary patterns, the available evidence can be used to compare these dietary regimens and provide an overall assessment regarding their efficacy.
Of the three approaches evaluated, the MDP appears to have the strongest body of evidence favoring its anti-inflammatory and cardioprotective effects. There are a greater number of large RCTs available to assess the effect of the MDP on various inflammatory markers compared to other dietary patterns. Moreover, the MDP was associated with decreases in several major inflammatory markers associated with inflammaging, including but not limited to CRP, IL-6, and TNF-α. By comparison, the PBP appears to have strong evidence favoring a reduction in CRP over long-term follow-up but has less effect on other markers despite its nutrient-rich, antioxidant properties. Similarly, the IF approach does not appear to impact as many inflammatory markers. The MDP also appears to reduce inflammation via multiple mechanisms, which may provide a more robust anti-inflammatory effect in comparison to the other two patterns. One such mechanism is shared between the PBP and MDP, as both emphasize the consumption of fruits, vegetables, legumes, nuts, and whole grains, providing high levels of antioxidant compounds such as phenols and flavonoids.
While all three dietary strategies appear to have significant anti-inflammatory properties, there is strong evidence to suggest that the MDP provides a more robust reduction in inflammation and oxidative stress, likely contributing to the improvement in inflammatory states that are associated with cardiovascular diseases such as hypertension, obesity, insulin resistance, and hyperlipidemia. This supports the idea that the anti-inflammatory qualities of the MDP may reduce the risk of cardiovascular disease outcomes.
OXIDATIVE STRESS
Oxidative stress refers to the imbalance between production and neutralization or elimination of reactive oxygen species (ROS). ROS include both free radicals and molecules that have the potential to become free radicals. Free radicals are molecules that contain unpaired electrons in their outer orbit, rendering them unstable and prone to react with other molecules by exchange of electrons. Non-free radical ROS can be reduced to form free radicals. Free radicals include superoxide, hydroxyl, peroxyl, hydroperoxyl and alkoxyl radicals. Other species that can generate free radicals include hydrogen peroxide, hydroxide ions, and peroxides[77]. Exchanging electrons by free radicals alters the structure of important molecules, including DNA, proteins, and lipids[78]. Some ROS-induced changes are necessary for certain biological processes, such as signaling. However, they can also cause damage to these molecules, which ultimately can accumulate and lead to further cellular damage[79,80].
The primary location of ROS generation is the mitochondria, by electron leakage from the electron transport chain (ETC). This occurs when NADH or FADH donate electrons to oxygen or water, producing hydrogen peroxide or superoxide[81,82]. However, additional processes in the cell can generate ROS, including β-oxidation of fatty acids, which produces hydrogen peroxide that occurs in the peroxisome[83].
Oxidative stress has been linked to the central mechanisms of cardiovascular aging, including atherosclerosis, diastolic dysfunction and decreased arterial compliance/endothelial dysfunction. The most common risk factors for cardiovascular disease- smoking, diabetes, hyperlipidemia, and hypertension- have all been associated with increased levels of oxidative stress[84-87].
Endothelial dysfunction has been linked to oxidative stress in multiple studies; oxidative stress contributes to endothelial dysfunction by negatively impacting flow-mediated vasodilation in elderly patients[88]. Higher levels of ROS in elderly patients were associated with measures of endothelial dysfunction[89]. Oxidative stress also leads to decreased bioavailability of nitric oxide, a key molecule in endothelial function[90].
Oxidative stress is also involved in the pathogenesis of atherosclerosis. Oxidized LDL (ox-LDL) is both a product of and an accelerator of oxidative stress; it is formed when LDL becomes oxidized and has been shown to impair the antioxidant properties of cells[91]. Increased oxidative stress activates signaling molecules that facilitate the uptake of ox-LDL to form foam cells, ultimately causing endothelial damage and inciting atherosclerotic changes[92]. Ox-LDL is also associated with higher degrees of arterial stiffness, independent of other traditional risk factors[93]. Decreased antioxidant systems in arterial walls have also been shown to accelerate atherogenesis[94-96].
Oxidative stress has been implicated in the development of myocardial dysfunction associated with heart failure. ROS impair excitation-coupling, leading to dysfunction in myocyte contractility[97]. Mitochondrial ROS have also been shown to contribute to cardiac fibrosis and hypertrophy[98]. Oxidative stress has also been linked to the dysfunction of calcium transport in the sarcoplasmic reticulum of myocytes, leading to impaired relaxation through increased myofilament sensitivity to calcium[99-101]. Diastolic dysfunction is also associated with endothelial dysfunction related to oxidative stress, as described above[102].
Dietary patterns exert their effects on cardiovascular aging in part through the modulation of levels of oxidative stress. The relationship between dietary patterns and oxidative stress has been defined through numerous human studies, while the mechanisms of this relationship have been elucidated mainly through animal models. The most studied dietary patterns include calorie restriction (CR), the Mediterranean dietary pattern (MDP), and western (high fat, high carbohydrate) diets; however, there have been an increasing number of studies investigating intermittent fasting (IF).
Dietary impact on oxidative stress
The main mechanisms by which dietary patterns lead to changes in levels of oxidative stress include enhanced mitochondrial efficiency, decreased ROS, and the antioxidant system [Figure 4].
Mitochondrial efficiency refers to maintaining mitochondrial function and ATP production while reducing levels of ROS produced and oxygen consumed. This occurs mainly through improvements in the electron transport chain (ETC) and through mitochondrial biogenesis, the process of generating new mitochondria[103]. PGC-1 alpha is a transcription factor that regulates mitochondrial biogenesis.
As mentioned above, ROS can be generated as byproducts of most metabolic processes in many cellular locations. The most studied process relevant to dietary effects of oxidative stress is oxidative phosphorylation of sugars and β-oxidation of free fatty acids. These produce the most studied ROS relevant to dietary patterns and aging, hydrogen peroxide and superoxide.
The antioxidants discussed in this review refer to molecules that catalyze the neutralization of ROS or protect structures against oxidation. The concentration of antioxidants is known to decrease with age[104]. The main antioxidant enzymes that are impacted by dietary patterns include manganese superoxide dismutase (MnSOD), catalase (CAT), glutathione peroxidase (GPx), superoxide dismutase (SOD), coenzyme Q (CoQ), quinone oxido-reductase (NQO), and NADH b5 reductase (CYB5R). The latter three also serve to protect membranes against oxidation which generates ROS[105].
Calorie restriction
Calorie restriction (CR) improves mitochondrial efficiency by improving the efficiency of the ETC. In numerous studies, CR has been shown to reduce proton leak from the ETC, thus reducing generation of ROS[106-108]. Long-term CR decreased the number of free radicals produced per unit of electron flow[109]. One study found this phenomenon occurred through lowering membrane potential, leading to reduced oxygen consumption and fewer ROS while maintaining the same levels of ATP production[110]. CR was also shown to attenuate the age-related decline in quality and quantity of ETC complexes, I-IV and citrate synthase[111].
Mitochondrial efficiency is also improved through mitochondrial biogenesis. CR attenuates the age-related decline in mitochondrial biogenesis through multiple pathways: (1) increase in peroxisome proliferator-activated receptor- coactivator (PGC1-alpha) gene activity that acts through the enzyme SIRT-1 to induce mitochondrial biogenesis, a finding which has been corroborated in humans; and (2) through increased endothelial NOS expression which also induces creation of new mitochondria[111-113].
CR also reduces production of free radicals and their damaging effects. CR decreased the amount of free radicals produced by the ETC per unit of electron flow[109]. CR has also been shown to decrease the bioactivity of superoxides, as well as decrease the activity of a major producer of superoxide ions, NADPH oxidase[114]. CR also alters membrane lipid composition such that they are less susceptible to peroxidation by ROS[115].
Finally, CR increases antioxidant systems, such as MnSOD[114]. In another study, CR was associated with less age-related decline in superoxide dismutase, CAT and GPx in mouse livers[116].
Overall, these mechanisms are supported by trials in humans that demonstrated CR reduced markers of elevated oxidative stress, namely F2-isoprostanes and advanced oxidation protein products (AOPP). The CALERIE 2 trial demonstrated that 2 years of CR reduced levels of F2-isoprostanes[108,117]. Another study showed reduced levels of AOPP in young overweight women[118].
While there is robust evidence for oxidative stress in CR, it may not be a feasible long-term dietary strategy due to difficulty with adherence. IF has emerged as an alternative strategy that may have similar benefits.
Intermittent fasting
Intermittent fasting (IF) has fewer studies elucidating the impact on oxidative stress. Some evidence has shown potential favorable effects by reducing ROS and increasing antioxidant systems. When modeled via every other day feeding in mice, IF increased levels of CoQ, NQO, and CYB5R[105]. IF has also been shown to induce SIRT-1 activity which upregulates antioxidant enzymes[119]. In a mouse model, IF reduced markers of oxidative stress in mouse hearts[120]. In humans, IF decreased AOPP in young overweight women[118]. Another study in overweight adults with asthma showed IF led to decreases in other markers of oxidative stress (8-isoprostane, nitrotyrosine, protein carbonyls and 4-hydroxynonel adducts)[121]. 8-isoprostane, a marker of oxidative stress to lipids, was reduced in TRE[122]. A study in adults with metabolic syndrome found a reduction in malondialdehyde, another marker of oxidative stress[123]. In another study, this effect persisted, independent of the amount of weight lost[124]. These early studies have shown promising effects of IF on reducing oxidative stress, but more data are needed to fully elucidate and support the beneficial effects of this dietary pattern.
Mediterranean dietary pattern
The Mediterranean dietary pattern (MDP) has been shown to decrease oxidative stress through an increase in antioxidant systems, reduction in ROS production, and mitochondrial biogenesis. The effects of the MDP on oxidative stress have mainly focused on the individual components central to this dietary pattern, such as nuts, oils, and vegetables.
Two components found in abundance in the MDP, oleic acid in olive oil and nuts and spermidine, or wheat germ, both increased mitochondrial efficiency. Oleic acid increased mitochondrial biogenesis by upregulating PGC1-alpha gene and SIRT-1[125]. Spermidine was found to increase mitochondrial turnover[126].
MDP has also been shown to decrease ROS production in human subjects[127,128]. Phenols, found in olive oil, have been shown to inhibit LDL oxidation, thus reducing ROS production[129]. Multiple studies have also found that the addition of unsaturated fats can protect against the oxidating effects of a high-fat diet (HFD)[125,130]. The MDP was found to decrease LDL oxidation, a source of ROS and a contributor to atherosclerosis in the PREDIMED study[131].
Numerous components in the MDP have also demonstrated antioxidant properties. Brazil nuts increased GPx activity[132]. Tomato juice also increases the activity of three other enzymes: superoxide dismutase, GPx, and CAT[133]. Nuts, fruits, and vegetables present in the MDP also supply the antioxidant CoQ[134]. Phenols were also shown to have antioxidant properties by scavenging free radicals[129]. Other dietary compounds abundant in the MDP, such as pomegranate juice, grapes and rice bran oil, have been shown to increase total plasma antioxidant capacity, a measure of the amount of antioxidant neutralization[135-138]. Total plasma antioxidant capacity has also been linked to a reduction in LDL and triglycerides in these same studies.
These data support reduction in oxidative stress as a main mechanism in attenuation of cardiovascular aging by the MDP. This differs from other dietary patterns described as the effects are exerted by individual dietary components rather than a distinct homogenous pattern. It is important to note that there is data supporting the reduction in oxidative stress by the pattern as a whole rather than the sum of its parts[98].
Western dietary pattern
The western dietary pattern is high in saturated fats, calories, and refined sugars. This leads to an increase in oxidative stress that has been demonstrated to accelerate the processes of cardiovascular aging. The western dietary pattern mainly leads to an increase in ROS generation but has also been shown to affect mitochondrial efficiency and antioxidant systems. Excess fat consumption leads to increased β-oxidation of free fatty acids, a major source of ROS[139]. HFDs also have higher rates of lipid peroxidation by ROS[116]. Saturated fats, abundant in the western dietary pattern, downregulate ROS scavenger molecules and induce ROS generation. While natural aging leads to a decline in mitochondrial biogenesis, high fat, high calorie western dietary pattern leads to an accelerated deterioration in this process[139]. Overall, the western dietary pattern provides a contrast to the dietary patterns described above, increasing levels of oxidative stress by reversing the mechanisms described above.
Conclusion
Dietary patterns cause alterations in levels of oxidative stress, ultimately leading to differing effects on cardiovascular aging. Increased oxidative stress has been shown to lead to accelerated atherosclerosis, endothelial dysfunction, increased arterial stiffness, and diastolic dysfunction. CR, IF, and the MDP have all been shown to decrease levels of oxidative stress, while the western dietary pattern increases levels of oxidative stress. Both the MDP and CR have been extensively studied and have significant support for their effects on reducing oxidative stress. Research on IF is scarce, but some studies have demonstrated promise towards the improvement of oxidative stress in humans, although it is unclear if this is the main mechanism of its benefit.
EPIGENETICS
Epigenetics refers to the modification of the genome and gene expression without alteration of the genetic code itself. Common examples of epigenetic modifications include DNA methylation, histone modification and non-coding RNAs such as microRNA (miRNA). These epigenetic alterations play an important role in facilitating differences between the genome and phenotypic expression and are influenced by environmental factors such as aging, diet and exercise[140]. Maternal diet and nutrient supplementation can lead to direct epigenetic changes in offspring predisposing to cardiovascular risk factors and disease[141,142]. Epigenetic changes have been shown to play a significant role in components of cardiovascular aging, including atherosclerosis, cardiac hypertrophy and decreased vascular compliance[143-145]. This section will further characterize the impact of epigenetic modifications on cardiovascular aging. In addition, we will examine the effect of specific dietary patterns on epigenetics in the setting of cardiovascular disease.
DNA methylation
DNA methylation is the most well-studied epigenetic modification in human disease. In humans, this modification most commonly occurs at 5th position carbon in a cytosine at cytosine-guanosine dinucleotides (CpG) and is facilitated by DNA methyltransferases (DNMT)[140]. DNA hypermethylation is typically associated with the repression of gene expression in CpG-rich gene promoters. DNA methylation also occurs in gene bodies, where it likely plays a role in alternative splicing of exons and variable gene expression[146]. DNMTs catalyze the methylation of specific genes and maintenance of DNA methylation within newly replicated DNA. DNA demethylation can also occur within the genome, either through the failure of methylation in replicated DNA or the oxidation of 5-methylcystosine to 5-hydroxymethylcytosine by ten-eleven translocation enzymes (TET)[143,147]. Both DNA hyper and hypomethylation play roles in human disease. Hypermethylation leads to decreased expression of essential protective genes, while hypomethylation can lead to inappropriate gene expression and reveal abnormal transcription sites[148].
DNA methylation plays a role in both cardiovascular disease and aging. Several models have been created that estimate age based on methylation patterns at specific DNA sites, known as “epigenetic age.” While methylation patterns often correlate with chronological age, rapid progression of epigenetic age is associated with many chronic conditions, including cardiovascular disease[149]. DNA methylation plays an important role in the regulation of atherosclerosis. Differential hyper and hypo-methylation is demonstrated at numerous CpG sites when comparing healthy vascular tissue and atherosclerotic plaques. Genes affected included several homeobox genes previously implicated in vascular diseases and TBX20, which was associated with upregulation of PPAR-ϒ and endothelial protection[150]. Chronic inflammation is involved in development of atherosclerosis, and DNA methylation is associated with regulation of inflammatory signal transduction pathways and expression of molecules such as TNF-a, IL-4, IL-6 and ICAM-1[148,151,152]. DNA methylation is altered in coronary artery disease (CAD), including in genes associated with lipid metabolism[153]. Variable methylation patterns have also been implicated in the pathogenesis of dyslipidemia, with one study noting altered LDL and VLDL levels associated with altered methylation at CpG sites within the carnitine palmitoyltransferase-1A gene[154]. Abnormal DNA methylation is also noted to be linked to cardiac hypertrophy, with both catecholamines and elevated left ventricular pressure leading to altered DNA methylation in cardiac tissue. Expression of both DNMTs and TETs are likely key regulators in this process and have been shown to be implicated in the expression of genes implicated in cardiac hypertrophy such as Myh6 and Xirp2[155,156]. Lastly, cardiac risk factors such as hypertension, elevated BMI, hyperlipidemia and insulin resistance lead to specific DNA methylation patterns with aging[157].
Histone modification and chromatin remodeling
Histone proteins bind directly to DNA to form chromatin, the basic structure of DNA within the cell nucleus. Chromatin is made up of individual nucleosomes, which are comprised of 147 DNA base pairs interacting with 8 histone proteins (2 each of H2A, H2B, H3 and H4)[158,159]. Histones can be directly modified through mechanisms such as methylation, acetylation, phosphorylation, etc. These modifications play a key role in altering the structure of chromatin and exposing DNA sites within the nucleosome for replication, transcription and repair[158]. Histone methylation is facilitated by different histone methyltransferases, either activating or suppressing a gene[159]. Histone acetylation is facilitated by histone acetyltransferase (HAT), exposing nucleosomal DNA to cellular machinery and leading to gene expression. This process is reversed through histone deacetylases (HDAC), and the balance of HAT and HDAC activity is closely related to DNA transcription. Phosphorylation is another common histone modification associated with both DNA expression and cellular repair mechanisms[158,159] .
Post-translation histone modification plays a key role in differential gene expression in cardiovascular aging. Alterations in histone deacetylation are related to the progression of atherosclerosis. Both HDAC over-expression and under-expression are linked to atherosclerosis, suggesting that interactions between histone acetylation and phenotypic expression are likely quite complex[160]. Methylation of H3K4 is associated with upregulation of numerous proinflammatory genes in macrophages, which play a key role in development of atherosclerotic plaques. These changes are associated with upregulation of several proinflammatory genes, such as TNF-α and IL-6[160,161]. Conversely, trimethylation of H3K27 has an inverse relationship with atherosclerosis in both human and murine models[160]. HDACs play a key role in the development of vascular hypertrophy and pathogenesis of hypertension, with one example being the facilitation of vascular smooth muscle proliferation by interaction of GATA-binding factor 6 and HDAC4[162]. HDACs also play a key role in the pathogenesis of LV hypertrophy and impaired myocardial relaxation, a process that was able to be lessened by a novel HDAC inhibitor in a murine model[163]. SIRT proteins associated with HDAC3 have been shown to have a protective effect and inhibit cardiac hypertrophy[155]. The role of histone methylation in this setting is quite complex and can promote or inhibit cardiac hypertrophy. Increased activity of JMJD2A, a demethylase of trimethylated H3K9, is associated with cardiac hypertrophy in both human and mouse models[164]. There is also evidence to suggest that additional histone modifications such as phosphorylation play a key role in cardiac hypertrophy, but more research is needed to characterize the mechanism of these changes[155].
Non-coding RNAs
Non-coding RNAs are products of transcribed DNA that do not encode proteins but play an essential role in regulating DNA transcription and expression[165,166]. Short non-coding RNAs are less than 200 base pairs, of which the most well-studied is miRNA. MiRNAs are approximately 20 base pairs in length and work by binding mRNA, leading to mRNA degradation and regulation of protein expression following transcription. Other non-coding RNAs include long-noncoding RNA (lncRNA) and circular RNA (circRNA). LncRNAs are typically greater than 200 base pairs in length and play complex roles in the regulation of chromatin, mRNA, and interprotein interactions. CircRNAs are the product of mRNA splicing, created through the joining of 5′ and 3′ ends of exons to form a closed loop. CircRNA function is not completely understood but is involved in the regulation of miRNAs[165,166].
Non-coding RNAs have been shown to be involved in the pathogenesis of cardiovascular disease, but their specific mechanism of action in cardiovascular aging is poorly understood. Several miRNAs are differentially expressed in atherosclerosis, leading to alteration in cellular pathways and endothelial, vascular smooth muscle and macrophage dysfunction[160,165,167]. miR-29 and miR-34a are upregulated in atherosclerosis, with miRNA-34a being linked to SIRT and histone deacetylation[167]. On the other hand, miR-24 has been shown to be protective against atherosclerosis through regulation of the CXCL12-CXCR4 axis[165]. LncRNA and circRNA have also been shown to have implications in atherosclerosis through the regulation of miRNAs and maintenance of vascular smooth muscle and endothelium[160,165,166]. miRNAs also play a role in myocardial hypertrophy and have been shown to be involved in several signaling pathways including thyroid hormone, IGF-1, TGF-β, calcineurin and NF-κB [168]. MiR-21 and miR-22 play key roles in fibroblast activity and cardiac hypertrophy[169,170]. Further, miR-34a is upregulated in cardiac aging and likely involved in dysregulation of myocardial repair[169]. Differential expression of lncRNAs has also been implicated in cardiac hypertrophy through several different mechanisms[166]. Much of the current literature focuses on the relationships between non-coding RNA expression and presence of cardiovascular disease. Additional studies are needed to characterize the specific mechanisms by which various non-coding RNAs are expressed and subsequently lead to a specific cardiovascular phenotype.
Dietary patterns, nutriepigenetics and cardiovascular aging
Epigenetic factors play a role in the regulation of cellular processes and cardiovascular homeostasis. Epigenetic changes are closely influenced by environmental and lifestyle factors. Nutriepigenetics describes the complex interaction between dietary patterns and epigenetic modifications. Dietary patterns lead to epigenetic change through several mechanisms, including alteration of cellular signaling in metabolic pathways, as well as direct epigenetic modifications by bioactive compounds[171]. With increasing evidence examining the relationship between food, epigenetics and cardiovascular health, dietary patterns are an important modifiable risk factor to help attenuate the effects of cardiovascular aging [Figure 5]. This section will examine the impact of Western, Mediterranean and intermittent fasting diet patterns on epigenetic changes and their relationship to cardiovascular aging.
Western dietary pattern
A western dietary pattern contains high dietary fat (HFD) content, which can contribute to several epigenetic changes related to cardiovascular disease as seen in animal models. Increased DNA methylation in mouse adipose tissue following 5-month exposure to HFD compared to standard diet led to an increase in expression of DNA methyltransferase 3a and methyl-CpG-binding domain protein 3, suggesting these proteins may be involved in increased DNA methylation following HFD[172]. Ciccarone et al. showed differential DNA methylation and hydroxymethylation as well as upregulation of Tet protein expression in murine cardiac tissue in response to a HFD[173]. These changes were associated with upregulation of markers associated with cardiac hypertrophy[173].
The effects of a HFD are similarly shown to lead to differential DNA methylation in human models. A
A HFD is also noted to have effects on both histone modification and non-coding RNA expression. A HFD led to hypercitrullination of histone H3 in LDL receptor knockout mice, increasing neutrophilic adhesion to vascular endothelium. Osaka et al. suggest that this process is likely related to vascular inflammation and atherogenesis[176]. A HFD increases histone deacetylation in mice via upregulation of HDAC3 and 6, which leads to decreased expression of methionine sulfoxide reductase A (MsrA). This decrease in MsrA expression leads to decreased homocysteine metabolism to H2S, leading to oxidative stress, vascular inflammation and hypertension[177]. An additional study in mice demonstrated cardiac hypertrophy and differential miRNA expression in cardiac tissue, correlating with percentage of dietary fat and length of HFD exposure[178].
Dietary salt has also been linked with nutriepigenetic changes related to cardiovascular aging, though there are fewer data when compared to a HFD. Dasinger et al. demonstrate that the administration of a high salt diet in a mouse model led to significant differential DNA methylation in both peripheral and renal T cells, likely contributing to hypertension and cellular inflammation[179]. Another murine model with SIRT3 knockout mice showed that SIRT3 plays a role in preventing hepatic inflammation, circulating inflammatory markers and hypertension[180]. SIRT3 was shown to be downregulated in response to high salt diet vs control, suggesting a key link between histone deacetylation and the development of cardiovascular disease in response to dietary sodium content. A high salt diet was also noted to cause differential expression of miRNA related to pathways associated with cardiac fibrosis in mice following nephrectomy[181].
Studies in humans have focused on salt reduction but provide valuable insight into the reversal of changes associated with high dietary sodium. One study assessed the effect of a low sodium diet on DNA methylation in a cohort of hypertensive participants, with significant improvement in BP noted over the study. Kidambi et al. showed 117 and 71 differential methylated genes in T cells and arteriolar tissue, respectively, with 4 common genes noted (P value = 0.009)[182]. In a RCT of hypertensive African American participants, a low sodium diet was shown to cause differential expression of 15 miRNA molecules including miR-143-3p. These changes were accompanied by a reduction in systolic blood pressure from 148.89 ± 13.47 to 143.66 ± 11.82 (P < 0.001) and pulse wave variation from 11.75 ± 2.07 to 11.21 ± 1.77
A western dietary pattern apparently contributes to the epigenetics changes leading to enhanced cardiovascular risk and premature cardiovascular aging. However, some of the evidence reviewed shows that simple modifications to the traditional western dietary pattern, including limiting dietary fat and salt, can help mitigate and potentially reverse some of the adverse effects of the western diet. It is important to characterize the negative epigenetic effects of the western diet, as it may yield diagnostic and therapeutic targets in future studies. Additional investigation is needed to characterize further epigenetic mechanisms through which the western dietary pattern leads to cardiovascular aging.
Mediterranean dietary pattern
The MDP has been shown to have prominent effects on DNA methylation at sites related to cardiovascular risk factors and aging. Ma et al. assessed the relationship between peripheral blood DNA methylation and diet quality, defined by Mediterranean-style Diet Score (MDS) and Alternative Healthy Eating Index (AHEI)[184]. Higher diet quality was associated with significantly increased DNA methylation at 30 CpG dinucleotides in genes associated with all-cause mortality, dyslipidemia, elevated BMI and Type II diabetes in European Americans[184]. In a cross-sectional analysis of 1995 participants in the Framingham Heart Study Offspring Cohort, increased diet quality as defined by MDS was associated with decreased epigenetic aging as defined by 3 standardized measures of epigenetic aging and DNA methylation (All
A MDP is linked to altered expression of miRNA related to inflammation and cardiovascular aging. One study evaluated a subset of the CORDIOPREV trial cohort, demonstrating that a MDP may be superior to a low-fat diet in regulation of endothelial function defined by flow-mediated dilation (FMD) of the brachial artery[188]. The authors further describe differential miRNA expression between a MDP and a low-fat diet in samples showing both severe and non-severe endothelial dysfunction. A MDP led to downregulation of the proapoptotic and proinflammatory miRNA (miR181c-5p and miRNA let-7e-5p) while increasing levels of miRNA (miR-939-5p and miR-25-5p) that inhibit gene expression associated with atherosclerosis, cellular senescence and inflammation. Another study assessed the impact of 8-week exposure to MDP on individuals with metabolic syndrome, finding altered levels of miRNA expression related to diet quality in peripheral leukocytes[189]. Marques-Rocha et al. go on to discuss the correlation between high diet quality and increased expression of the miRNA let-7b, which is likely protective against endothelial dysfunction and atherosclerotic disease[189].
In an additional analysis of participants within the PREDIMED trial, a MDP supplemented either with olive oil or tree nuts was shown to cause differential alterations in DNA methylation of genes associated with inflammation, metabolism and cardiovascular risk in white blood cells[190]. While both were associated with positive cardiovascular outcomes, this study indicates that dietary fat composition will impact epigenetic factors differently. Extra virgin olive oil, an important component of the MDP, contains phenols that lead to numerous epigenetic alterations associated with anti-inflammatory and cardioprotective effects[191,192]. Other diet components of the MDP, such as grains, fruits, vegetables and wine, are rich in polyphenols like resveratrol. These polyphenolic compounds, along with polyunsaturated fats, are bioactive and are associated with direct epigenetic changes and anti-inflammatory phenotype[193].
A MDP leads to epigenetic changes that may be protective against changes associated with cardiovascular aging. There is still a wide gap in the exact mechanisms through which epigenetic modifications contribute to these changes, and additional studies are needed to identify additional epigenetic markers, target genes and their relationship with specific cardiovascular outcomes. Differences in mRNA expression between MDP supplemented with either nuts or olive oil have also been characterized, which suggests the presence of heterogenous epigenetic changes even within subtypes of the MDP and needs further examination[194].
Intermittent fasting
Intermittent fasting is noted to have positive effects on several cardiovascular risk factors in humans, including blood pressure, insulin resistance, obesity and dyslipidemia[195]. Intermittent fasting may lead to increased circulating ketone bodies in the setting of carbohydrate restriction. Ketones can cause changes in cellular signaling as well as direct histone modifications such as β-hydroxybutyrylation, methylation and acetylation[196]. Both SIRT1 and SIRT2 levels are increased in adipose tissue in response to intermittent fasting and lead to histone deacetylation of several genes[197]. SIRT1 plays a key role in reduction of inflammation and downregulation of inflammatory pathways such as NFkB and IL-1β, while SIRT2 is implicated in reduced oxidative stress. In a cohort of 9 overweight adults, time-restricted eating with a
Conclusion
Epigenetics is clearly influenced by diet and plays an essential role in normal human development and homeostasis. Nutriepigenetic changes are demonstrated to impact pathways involved in cardiovascular aging through DNA methylation, histone modification and non-coding RNAs. Of the evidence reviewed in this section, the MDP may be an epigenetic modifier to protect against cardiovascular aging. Simple modifications of the traditional western diet, such as limiting salt and fat, may also lessen cardiovascular risk factors through epigenetic modification. While early data is provocative, most studies are defined by simple correlations between epigenetic changes and disease. There is still a gap in evidence regarding the specific mechanisms of dietary epigenetic change and how these changes directly influence cardiovascular phenotype. Additional evidence is needed to characterize these changes as they pertain to dietary patterns including the western diet, MDP and intermittent fasting. Further, other diet patterns were disproportionately under-represented in the scientific literature and thus provided an area for additional research. A plant-based dietary pattern has similar benefits to the MDP, but currently lacks quality studies assessing epigenetic mechanisms and cardiovascular impact. Lastly, additional research is needed to assess the impact of maternal diet on epigenetics and cardiovascular disease in offspring. Maternal exposure to both famine and overnutrition has been shown to alter cardiovascular risk in offspring, but there is little data assessing the epigenetic mechanisms of this phenomenon or if any of the beneficial dietary patterns discussed would provide a cardioprotective benefit to subsequent generations. In conclusion, evidence supports diet as an intervention to protect against the epigenetic component of cardiovascular aging, but more evidence is needed to support specific dietary patterns and specific mechanisms of nutriepigentic change.
DISCUSSION
Age is the main risk factor for the development of cardiovascular disease and is thought to be a non-modifiable one at that. If we can understand the molecular and biological mechanisms that underlie aging, perhaps we can develop preventative and therapeutic interventions to combat CVD.
In this review, we highlighted three of the most well-established mechanisms that drive cardiovascular aging- chronic inflammation, oxidative stress and epigenetics- and discussed the present evidence of the impact of healthy and unhealthy dietary patterns on these aging mechanisms. The Mediterranean Dietary pattern has been evaluated the most and has demonstrated beneficial effects on all aging mechanisms. There are other dietary patterns- plant-based, intermittent fasting- that require extensive evaluation and studies; however, some early results have demonstrated benefits.
Practitioners should be aware of the beneficial effects of healthy dietary patterns and the harms of others when guiding their patients on optimal dietary practices. This review helps to shed light on the Mediterranean dietary pattern as a promising tool to slow down cardiovascular aging. It is the least restrictive pattern and also has the largest body of evidence. Therefore, we would recommend the Mediterranean dietary pattern to most patients with an eye towards future studies on the evaluation of intermittent fasting and plant-based dietary patterns.
DECLARATIONS
Authors’ contributions
Chronic inflammation: Lewis SA
Oxidative stress: Koch RL
Epigenetics: Britt WM
Tables and Figures: Razavi AC, Koch RL, Lewis SA, Britt WM
Manuscript conception, organization and design: D’Souza MS, Patel P, Sperling LS
All authors reviewed and approved the final version of the manuscript.
Availability of data and materials
Not applicable.
Financial support and sponsorship
None.
Conflicts of interest
All authors declared that there are no conflicts of interest.
Ethical approval and consent to participate
Not applicable.
Consent for publication
Not applicable.
Copyright
© The Author(s) 2023.
REFERENCES
1. Gilbert SF. Developmental biology. Sunderland: Sinauer Associates, Inc.; 2010.
2. North BJ, Sinclair DA. The intersection between aging and cardiovascular disease. Circ Res 2012;110:1097-108.
3. Goff Jr DC, Lloyd-Jones DM, Bennett G, et al. 2013 ACC/AHA guideline on the assessment of cardiovascular risk: a report of the American College of Cardiology/American Heart Association Task Force on Practice Guidelines. Circulation 2014;129:S49-S73.
4. Heidenreich PA, Trogdon JG, Khavjou OA, et al. Forecasting the future of cardiovascular disease in the United States: a policy statement from the American Heart Association. Circulation 2011;123:933-44.
5. Fleg JL, Aronow WS, Frishman WH. Cardiovascular drug therapy in the elderly: benefits and challenges. Nat Rev Cardiol 2011;8:13-28.
6. Afshin A, Sur PJ, Fay KA, et al. Health effects of dietary risks in 195 countries, 1990-2017: a systematic analysis for the Global Burden of Disease Study 2017. Lancet 2019;393:1958-72.
7. Kim H, Caulfield LE, Garcia-Larsen V, Steffen LM, Coresh J, Rebholz CM. Plant-based diets are associated with a lower risk of incident cardiovascular disease, cardiovascular disease mortality, and all-cause mortality in a general population of middle-aged adults. J Am Heart Assoc 2019;8:e012865.
8. Yokoyama Y, Nishimura K, Barnard ND, et al. Vegetarian diets and blood pressure: a meta-analysis. JAMA Int Med 2014;174:577-87.
9. Razavi AC, Bazzano LA, He J, et al. Consumption of animal and plant foods and risk of left ventricular diastolic dysfunction: the Bogalusa Heart Study. ESC Heart Fail 2020;7:2700-10.
10. Pietri P, Stefanadis C. Cardiovascular aging and longevity: JACC state-of-the-art review. J Am Coll Cardiol 2021;77:189-204.
12. Estruch R, Ros E, Salas-Salvadó J, et al. Primary prevention of cardiovascular disease with a Mediterranean diet supplemented with extra-virgin olive oil or nuts. New Engl J Med 2018;379:1388.
13. Appel LJ, Brands MW, Daniels SR, Karanja N, Elmer PJ, Sacks FM. Dietary approaches to prevent and treat hypertension: a scientific statement from the American Heart Association. Hypertension 2006;47:296-308.
14. Dybvik JS, Svendsen M, Aune D. Vegetarian and vegan diets and the risk of cardiovascular disease, ischemic heart disease and stroke: a systematic review and meta-analysis of prospective cohort studies. Eur J Nutr ;2022:1-19.
15. Franceschi C, Salvioli S, Garagnani P, de Eguileor M, Monti D, Capri M. Immunobiography and the heterogeneity of immune responses in the elderly: a focus on inflammaging and trained immunity. Front Immunol 2017;8:982.
16. Ferrucci L, Fabbri E. Inflammageing: chronic inflammation in ageing, cardiovascular disease, and frailty. Nat Rev Cardiol 2018;15:505-22.
17. Petrie JR, Guzik TJ, Touyz RM. Diabetes, hypertension, and cardiovascular disease: clinical insights and vascular mechanisms. Can J Cardiol 2018;34:575-84.
18. Bobryshev YV, Ivanova EA, Chistiakov DA, Nikiforov NG, Orekhov AN. Macrophages and their role in atherosclerosis: pathophysiology and transcriptome analysis. Biomed Res Int 2016;2016:9582430.
19. Malinowski B, Zalewska K, Węsierska A, et al. Intermittent fasting in cardiovascular disorders-an overview. Nutrients 2019;11:673.
20. Gude NA, Broughton KM, Firouzi F, Sussman MA. Cardiac ageing: extrinsic and intrinsic factors in cellular renewal and senescence. Nat Rev Cardiol 2018;15:523-42.
21. Gorenne I, Kavurma M, Scott S, Bennett M. Vascular smooth muscle cell senescence in atherosclerosis. Cardiovasc Res 2006;72:9-17.
22. Minamino T, Miyauchi H, Yoshida T, Ishida Y, Yoshida H, Komuro I. Endothelial cell senescence in human atherosclerosis: role of telomere in endothelial dysfunction. Circulation 2002;105:1541-4.
23. Morgan RG, Ives SJ, Lesniewski LA, et al. Age-related telomere uncapping is associated with cellular senescence and inflammation independent of telomere shortening in human arteries. Am J Physiol Heart Circ Physiol 2013;305:H251-8.
24. Sun Y, Wang X, Liu T, Zhu X, Pan X. The multifaceted role of the SASP in atherosclerosis: from mechanisms to therapeutic opportunities. Cell Biosci 2022;12:74.
25. Childs BG, Baker DJ, Wijshake T, Conover CA, Campisi J, van Deursen JM. Senescent intimal foam cells are deleterious at all stages of atherosclerosis. Science 2016;354:472-7.
26. Stojanović SD, Fuchs M, Kunz M, et al. Inflammatory drivers of cardiovascular disease: molecular characterization of senescent coronary vascular smooth muscle cells. Front Physiol 2020;11:520.
27. Demaria M, Ohtani N, Youssef SA, et al. An essential role for senescent cells in optimal wound healing through secretion of PDGF-AA. Dev Cell 2014;31:722-33.
28. Liberale L, Montecucco F, Tardif JC, Libby P, Camici GG. Inflamm-ageing: the role of inflammation in age-dependent cardiovascular disease. Eur Heart J 2020;41:2974-82.
29. Wang M, Kim SH, Monticone RE, Lakatta EG. Matrix metalloproteinases promote arterial remodeling in aging, hypertension, and atherosclerosis. Hypertension 2015;65:698-703.
30. Jones NL, Reagan JW, Willingham MC. The pathogenesis of foam cell formation: modified LDL stimulates uptake of co-incubated LDL via macropinocytosis. Arterioscler Thromb Vasc Biol 2000;20:773-81.
31. Singh SK, Suresh MV, Voleti B, Agrawal A. The connection between C-reactive protein and atherosclerosis. Ann Med 2008;40:110-20.
32. Anthony SR, Guarnieri AR, Gozdiff A, Helsley RN, Phillip Owens A, Tranter M. Mechanisms linking adipose tissue inflammation to cardiac hypertrophy and fibrosis. Clin Sci 2019;133:2329-44.
33. Park YM, Myers M, Vieira-Potter VJ. Adipose tissue inflammation and metabolic dysfunction: role of exercise. Mo Med 2014;111:65-72.
35. Khafagy R, Dash S. Obesity and cardiovascular disease: the emerging role of inflammation. Front Cardiovasc Med 2021;8:768119.
36. Ormazabal V, Nair S, Elfeky O, Aguayo C, Salomon C, Zuñiga FA. Association between insulin resistance and the development of cardiovascular disease. Cardiovasc Diabetol 2018;17:122.
37. Kaur N, Guan Y, Raja R, Ruiz-Velasco A, Liu W. Mechanisms and therapeutic prospects of diabetic cardiomyopathy through the inflammatory response. Front Physiol 2021;12:694864.
38. Wu PY, Chen KM, Tsai WC. The mediterranean dietary pattern and inflammation in older adults: a systematic review and meta-analysis. Adv Nutr 2021;12:363-73.
39. Tsigalou C, Konstantinidis T, Paraschaki A, Stavropoulou E, Voidarou C, Bezirtzoglou E. Mediterranean diet as a tool to combat inflammation and chronic diseases. an overview. Biomedicines 2020;8:201.
40. Mena MP, Sacanella E, Vazquez-Agell M, et al. Inhibition of circulating immune cell activation: a molecular antiinflammatory effect of the Mediterranean diet. Am J Clin Nutr 2009;89:248-56.
41. Bonaccio M, Di Castelnuovo A, De Curtis A, et al. Adherence to the mediterranean diet is associated with lower platelet and leukocyte counts: results from the moli-sani study. Blood 2014;123:3037-44.
42. Merra G, Noce A, Marrone G, et al. Influence of mediterranean diet on human gut microbiota. Nutrients 2020;13:7.
43. Meslier V, Laiola M, Roager HM, et al. Mediterranean diet intervention in overweight and obese subjects lowers plasma cholesterol and causes changes in the gut microbiome and metabolome independently of energy intake. Gut 2020;69:1258-68.
44. Kimble R, Gouinguenet P, Ashor A, et al. Effects of a mediterranean diet on the gut microbiota and microbial metabolites: A systematic review of randomized controlled trials and observational studies. Crit Rev Food Sci Nutr 2022;2022:1-22.
45. Lorgeril M, Salen P, Martin JL, Monjaud I, Delaye J, Mamelle N. Mediterranean diet, traditional risk factors, and the rate of cardiovascular complications after myocardial infarction: final report of the Lyon Diet Heart Study. Circulation 1999;99:779-85.
46. Shikany JM, Safford MM, Soroka O, et al. Mediterranean diet score, dietary patterns, and risk of sudden cardiac death in the regards study. J Am Heart Assoc 2021;10:e019158.
47. Esposito K, Maiorino MI, Di Palo C, Giugliano D. Adherence to a mediterranean diet and glycaemic control in type 2 diabetes mellitus. Diabet Med 2009;26:900-7.
48. Esposito K, Marfella R, Ciotola M, et al. Effect of a mediterranean-style diet on endothelial dysfunction and markers of vascular inflammation in the metabolic syndrome: a randomized trial. JAMA 2004;292:1440-6.
49. Estruch R, Martínez-González MA, Corella D, et al. Effects of a mediterranean-style diet on cardiovascular risk factors: a randomized trial. Ann Intern Med 2006;145:1-11.
50. Martín-Peláez S, Fito M, Castaner O. Mediterranean diet effects on type 2 diabetes prevention, disease progression, and related mechanisms: a review. Nutrients 2020;12:2236.
51. Sleiman D, Al-Badri MR, Azar ST. Effect of mediterranean diet in diabetes control and cardiovascular risk modification: a systematic review. Front Public Health 2015;3:69.
52. Menzel J, Biemann R, Longree A, et al. Associations of a vegan diet with inflammatory biomarkers. Sci Rep 2020;10:1933.
53. Haghighatdoost F, Bellissimo N, Totosy de Zepetnek JO, Rouhani MH. Association of vegetarian diet with inflammatory biomarkers: a systematic review and meta-analysis of observational studies. Public Health Nutr 2017;20:2713-21.
54. Menzel J, Jabakhanji A, Biemann R, Mai K, Abraham K, Weikert C. Systematic review and meta-analysis of the associations of vegan and vegetarian diets with inflammatory biomarkers. Sci Rep 2020;10:21736.
55. Szabo Z, Koczka V, Marosvolgyi T, et al. Possible biochemical processes underlying the positive health effects of plant-based diets-a narrative review. Nutrients 2021;13:2593.
56. Ashor AW, Lara J, Mathers JC, Siervo M. Effect of vitamin C on endothelial function in health and disease: a systematic review and meta-analysis of randomised controlled trials. Atherosclerosis 2014;235:9-20.
57. Juraschek SP, Guallar E, Appel LJ, Miller ER 3rd. Effects of vitamin C supplementation on blood pressure: a meta-analysis of randomized controlled trials. Am J Clin Nutr 2012;95:1079-88.
58. D’Souza MS, Dong TA, Ragazzo G, et al. From fad to fact: evaluating the impact of emerging diets on the prevention of cardiovascular disease. Am J Med 2020;133:1126-34.
59. Aksungar FB, Topkaya AE, Akyildiz M. Interleukin-6, C-reactive protein and biochemical parameters during prolonged intermittent fasting. Ann Nutr Metab 2007;51:88-95.
60. Varady KA, Bhutani S, Klempel MC, et al. Alternate day fasting for weight loss in normal weight and overweight subjects: a randomized controlled trial. Nutr J 2013;12:146.
61. Wang X, Yang Q, Liao Q, et al. Effects of intermittent fasting diets on plasma concentrations of inflammatory biomarkers: A systematic review and meta-analysis of randomized controlled trials. Nutrition 2020;79-80:110974.
62. Liu Y, Vu V, Sweeney G. Examining the potential of developing and implementing use of adiponectin-targeted therapeutics for metabolic and cardiovascular diseases. Front Endocrinol 2019;10:842.
63. Feizollahzadeh S, Rasuli J, Kheirouri S, Alizadeh M. Augmented plasma adiponectin after prolonged fasting during ramadan in men. Health Promot Perspect 2014;4:77-81.
64. Cho Y, Hong N, Kim KW, et al. The effectiveness of intermittent fasting to reduce body mass index and glucose metabolism: a systematic review and meta-analysis. J Clin Med 2019;8:1645.
65. Horne BD, Muhlestein JB, May HT, et al. Relation of routine, periodic fasting to risk of diabetes mellitus, and coronary artery disease in patients undergoing coronary angiography. Am J Cardiol 2012;109:1558-62.
66. Nematy M, Alinezhad-Namaghi M, Rashed MM, et al. Effects of Ramadan fasting on cardiovascular risk factors: a prospective observational study. Nutr J 2012;11:69.
67. Mayra ST, Johnston CS. Arterial stiffness and cardiometabolic health in omnivores and vegetarians: a cross-sectional pilot study. BMC Res Notes 2022;15:69.
68. Zuo L, He F, Tinsley GM, Pannell BK, Ward E, Arciero PJ. Comparison of high-protein, intermittent fasting low-calorie diet and heart healthy diet for vascular health of the obese. Front Physiol 2016;7:350.
69. Machado d’Almeida K, Ronchi Spillere S, Zuchinali P, Corrêa Souza G. Mediterranean diet and other dietary patterns in primary prevention of heart failure and changes in cardiac function markers: a systematic review. Nutrients 2018;10:58.
70. Chrysohoou C, Pitsavos C, Metallinos G, et al. Cross-sectional relationship of a Mediterranean type diet to diastolic heart function in chronic heart failure patients. Heart Vessels 2012;27:576-84.
71. Papadaki A, Martínez-González MÁ, Alonso-Gómez A, et al. Mediterranean diet and risk of heart failure: results from the PREDIMED randomized controlled trial. Eur J Heart Fail 2017;19:1179-85.
72. Varadarajan P, Pai RG, Fraser GE, et al. Left ventricular diastolic abnormalities in vegetarians as compared with non-vegetarians. Br J Nutr 2022:1-28.
73. Okoshi K, Cezar MDM, Polin MAM, et al. Influence of intermittent fasting on myocardial infarction-induced cardiac remodeling. BMC Cardiovasc Disord 2019;19:126.
74. Lee KW, Loh HC, Ching SM, Devaraj NK, Hoo FK. Effects of vegetarian diets on blood pressure lowering: a systematic review with meta-analysis and trial sequential analysis. Nutrients 2020;12:1604.
75. Barnard ND, Alwarith J, Rembert E, et al. A mediterranean diet and low-fat vegan diet to improve body weight and cardiometabolic risk factors: a randomized, cross-over trial. J Am Nutr Assoc 2022;41:127-39.
76. de Toledo F, Grundler F, Bergouignan A, Drinda S, Michalsen A. Safety, health improvement and well-being during a 4 to 21-day fasting period in an observational study including 1422 subjects. PLoS One 2019;14:e0209353.
77. Phaniendra A, Jestadi DB, Periyasamy L. Free radicals: properties, sources, targets, and their implication in various diseases. Indian J Clin Biochem 2015;30:11-26.
78. Pham-Huy LA, He H, Pham-Huy C. Free radicals, antioxidants in disease and health. Int J Biomed Sci 2008;4:89.
79. Sohal RS. Role of oxidative stress and protein oxidation in the aging process. Free Radic Biol Med 2002;33:37-44.
81. Brieger K, Schiavone S, Miller FJ, Krause KH. Reactive oxygen species: from health to disease. Swiss Med Wkly 2012;142:w13659.
82. Tahara EB, Navarete FD, Kowaltowski AJ. Tissue-, substrate-, and site-specific characteristics of mitochondrial reactive oxygen species generation. Free Radic Biol Med 2009;46:1283-97.
84. Guzik TJ, Sadowski J, Guzik B, et al. Coronary artery superoxide production and nox isoform expression in human coronary artery disease. Arterioscler Thromb Vasc Biol 2006;26:333-9.
85. Steffen Y, Vuillaume G, Stolle K, et al. Cigarette smoke and LDL cooperate in reducing nitric oxide bioavailability in endothelial cells via effects on both eNOS and NADPH oxidase. Nitric Oxide 2012;27:176-84.
87. Li H, Förstermann U. Uncoupling of endothelial NO synthase in atherosclerosis and vascular disease. Curr Opin Pharmacol 2013;13:161-7.
88. Donato AJ, Eskurza I, Silver AE, et al. Direct evidence of endothelial oxidative stress with aging in humans: relation to impaired endothelium-dependent dilation and upregulation of nuclear factor-κB. Circ Res 2007;100:1659-66.
89. Rodríguez-Mañas L, El-Assar M, Vallejo S, et al. Endothelial dysfunction in aged humans is related with oxidative stress and vascular inflammation. Aging Cell 2009;8:226-38.
90. Taddei S, Virdis A, Ghiadoni L, et al. Age-related reduction of NO availability and oxidative stress in humans. Hypertension 2001;38:274-9.
91. Fujimoto H, Kobayashi H, Ohno M. Age-induced reduction in mitochondrial manganese superoxide dismutase activity and tolerance of macrophages against apoptosis induced by oxidized low density lipoprotein. Circ J 2010;74:353-60.
92. Kattoor AJ, Pothineni NVK, Palagiri D, Mehta JL. Oxidative stress in atherosclerosis. Curr Atheroscler Rep 2017;19:42.
93. Brinkley TE, Nicklas BJ, Kanaya AM, et al. Plasma oxidized low-density lipoprotein levels and arterial stiffness in older adults: the health, aging, and body composition study. Hypertension 2009;53:846-52.
94. Madamanchi NR, Runge MS. Mitochondrial dysfunction in atherosclerosis. Circ Res 2007;100:460-73.
95. Yang H, Roberts LJ, Shi MJ, et al. Retardation of atherosclerosis by overexpression of catalase or both Cu/Zn-superoxide dismutase and catalase in mice lacking apolipoprotein E. Circ Res 2004;95:1075-81.
96. Cheng F, Torzewski M, Degreif A, Rossmann H, Canisius A, Lackner KJ. Impact of glutathione peroxidase-1 deficiency on macrophage foam cell formation and proliferation: implications for atherogenesis. PloS one 2013;8:e72063.
97. Maack C, Böhm M. Targeting mitochondrial oxidative stress in heart failure throttling the afterburner. J Am Coll Cardiol 2011;58:83-6.
98. Dai DF, Johnson SC, Villarin JJ, et al. Mitochondrial oxidative stress mediates angiotensin II-induced cardiac hypertrophy and Galphaq overexpression-induced heart failure. Circ Res 2011;108:837-46.
99. Qin F, Siwik DA, Lancel S, et al. Hydrogen peroxide-mediated SERCA cysteine 674 oxidation contributes to impaired cardiac myocyte relaxation in senescent mouse heart. J Am Heart Assoc 2013;2:e000184.
100. Gonzalez DR, Treuer AV, Castellanos J, Dulce RA, Hare JM. Impaired S-nitrosylation of the ryanodine receptor caused by xanthine oxidase activity contributes to calcium leak in heart failure. J Biol Chem 2010;285:28938-45.
101. Erickson JR, Mei-ling AJ, Guan X, et al. A dynamic pathway for calcium-independent activation of CaMKII by methionine oxidation. Cell 2008;133:462-74.
102. Raad M, AlBadri A, Wei J, et al. Oxidative Stress is associated with diastolic dysfunction in women with ischemia with no obstructive coronary artery disease. J Am Heart Assoc 2020;9:e015602.
103. Brand M. The efficiency and plasticity of mitochondrial energy transduction. Biochem Soc Trans 2005;33:897-904.
104. Wei YH, Lee HC. Oxidative stress, mitochondrial DNA mutation, and impairment of antioxidant enzymes in aging. Exp Biol Med 2002;227:671-82.
105. Rodríguez-Bies E, Navas P, López-Lluch G. Age-dependent effect of every-other-day feeding and aerobic exercise in ubiquinone levels and related antioxidant activities in mice muscle. J Gerontol Ser A 2015;70:33-43.
106. Hagopian K, Harper ME, Ram JJ, Humble SJ, Weindruch R, Ramsey JJ. Long-term calorie restriction reduces proton leak and hydrogen peroxide production in liver mitochondria. Am J Physiol Endocrinol Metab 2005;288:E674-84.
107. Bevilacqua L, Ramsey JJ, Hagopian K, Weindruch R, Harper ME. Effects of short-and medium-term calorie restriction on muscle mitochondrial proton leak and reactive oxygen species production. Am J Physiol Endocrinol Metab 2004;286:E852-E61.
108. Redman LM, Smith SR, Burton JH, Martin CK, Il’yasova D, Ravussin E. Metabolic slowing and reduced oxidative damage with sustained caloric restriction support the rate of living and oxidative damage theories of aging. Cell Metab 2018;27:805-815.e4.
109. Gredilla R, Sanz A, Lopez-Torres M, Barja G. Caloric restriction decreases mitochondrial free radical generation at complex I and lowers oxidative damage to mitochondrial DNA in the rat heart. FASEB J 2001;15:1589-91.
110. López-Lluch G, Hunt N, Jones B, et al. Calorie restriction induces mitochondrial biogenesis and bioenergetic efficiency. Proc Natl Acad Sci USA 2006;103:1768-73.
111. Baker DJ, Betik AC, Krause DJ, Hepple RT. No decline in skeletal muscle oxidative capacity with aging in long-term calorically restricted rats: effects are independent of mitochondrial DNA integrity. J Gerontol Ser A 2006;61:675-84.
112. Civitarese AE, Carling S, Heilbronn LK, et al. Calorie restriction increases muscle mitochondrial biogenesis in healthy humans. PLoS Med 2007;4:e76.
113. Nisoli E, Tonello C, Cardile A, et al. Calorie restriction promotes mitochondrial biogenesis by inducing the expression of eNOS. Science 2005;310:314-7.
114. Rippe C, Lesniewski L, Connell M, LaRocca T, Donato A, Seals D. Short-term calorie restriction reverses vascular endothelial dysfunction in old mice by increasing nitric oxide and reducing oxidative stress. Aging cell 2010;9:304-12.
115. Faulks SC, Turner N, Else PL, Hulbert AJ. Calorie restriction in mice: effects on body composition, daily activity, metabolic rate, mitochondrial reactive oxygen species production, and membrane fatty acid composition. J Gerontol A Biol Sci Med Sci 2006;61:781-94.
116. Pieri C, Falasca M, Marcheselli F, et al. Food restriction in female Wistar rats: V. Lipid peroxidation and antioxidant enzymes in the liver. Arch Gerontol Geriatr 1992;14:93-9.
117. Il’yasova D, Fontana L, Bhapkar M, et al. Effects of 2 years of caloric restriction on oxidative status assessed by urinary F2-isoprostanes: The CALERIE 2 randomized clinical trial. Aging Cell 2018;17:e12719.
118. Harvie MN, Pegington M, Mattson MP, et al. The effects of intermittent or continuous energy restriction on weight loss and metabolic disease risk markers: a randomized trial in young overweight women. Int J Obes 2011;35:714-27.
119. Fulco M, Cen Y, Zhao P, et al. Glucose restriction inhibits skeletal myoblast differentiation by activating SIRT1 through AMPK-mediated regulation of Nampt. Dev Cell 2008;14:661-73.
120. Castello L, Froio T, Maina M, et al. Alternate-day fasting protects the rat heart against age-induced inflammation and fibrosis by inhibiting oxidative damage and NF-kB activation. Free Radic Biol Med 2010;48:47-54.
121. Johnson JB, Summer W, Cutler RG, et al. Alternate day calorie restriction improves clinical findings and reduces markers of oxidative stress and inflammation in overweight adults with moderate asthma. Free Radic Biol Med 2007;42:665-74.
122. Cienfuegos S, Gabel K, Kalam F, et al. Effects of 4- and 6-h time-restricted feeding on weight and cardiometabolic health: a randomized controlled trial in adults with obesity. Cell Metab 2020;32:366-378.e3.
123. Guo Y, Luo S, Ye Y, Yin S, Fan J, Xia M. Intermittent fasting improves cardiometabolic risk factors and alters gut microbiota in metabolic syndrome patients. J Clin Endocrinol Metab 2021;106:64-79.
124. Sutton EF, Beyl R, Early KS, Cefalu WT, Ravussin E, Peterson CM. Early time-restricted feeding improves insulin sensitivity, blood pressure, and oxidative stress even without weight loss in men with prediabetes. Cell Metab 2018;27:1212-21.e3.
125. Yuzefovych L, Wilson G, Rachek L. Different effects of oleate vs. palmitate on mitochondrial function, apoptosis, and insulin signaling in L6 skeletal muscle cells: role of oxidative stress. Am J Physiol Endocrinol Metab 2010;299:E1096-105.
126. Eisenberg T, Abdellatif M, Schroeder S, et al. Cardioprotection and lifespan extension by the natural polyamine spermidine. Nat Med 2016;22:1428-38.
127. Marin C, Delgado-Lista J, Ramirez R, et al. Mediterranean diet reduces senescence-associated stress in endothelial cells. Age 2012;34:1309-16.
128. Cicero AF, Nascetti S, López-Sabater MC, et al. Changes in LDL fatty acid composition as a response to olive oil treatment are inversely related to lipid oxidative damage: The Eurolive study. J Am Coll Nutr 2008;27:314-20.
129. Visioli F, Poli A, Gall C. Antioxidant and other biological activities of phenols from olives and olive oil. Med Res Rev 2002;22:65-75.
130. Kwon B, Lee HK, Querfurth HW. Oleate prevents palmitate-induced mitochondrial dysfunction, insulin resistance and inflammatory signaling in neuronal cells. Biochim Biophys Acta 2014;1843:1402-13.
131. Fitó M, Guxens M, Corella D, et al. Effect of a traditional Mediterranean diet on lipoprotein oxidation: a randomized controlled trial. Arch Int Med 2007;167:1195-203.
132. Huguenin GV, Oliveira GM, Moreira AS, et al. Improvement of antioxidant status after Brazil nut intake in hypertensive and dyslipidemic subjects. Nutr J 2015;14:54.
133. Ghavipour M, Sotoudeh G, Ghorbani M. Tomato juice consumption improves blood antioxidative biomarkers in overweight and obese females. Clin Nutr 2015;34:805-9.
134. Gylling H, Plat J, Turley S, et al. Plant sterols and plant stanols in the management of dyslipidaemia and prevention of cardiovascular disease. Atherosclerosis 2014;232:346-60.
135. Bumrungpert A, Chongsuwat R, Phosat C, Butacnum A. Rice bran oil containing gamma-oryzanol improves lipid profiles and antioxidant status in hyperlipidemic subjects: a randomized double-blind controlled trial. J Altern Complement Med 2019;25:353-8.
136. Asemi Z, Samimi M, Tabassi Z, Shakeri H, Sabihi SS, Esmaillzadeh A. Effects of DASH diet on lipid profiles and biomarkers of oxidative stress in overweight and obese women with polycystic ovary syndrome: a randomized clinical trial. Nutrition 2014;30:1287-93.
137. Rahbar AR, Mahmoudabadi MM, Islam MS. Comparative effects of red and white grapes on oxidative markers and lipidemic parameters in adult hypercholesterolemic humans. Food Funct 2015;6:1992-8.
138. Sohrab G, Ebrahimof S, Sotoudeh G, et al. Effects of pomegranate juice consumption on oxidative stress in patients with type 2 diabetes: a single-blind, randomized clinical trial. Int J Food Sci Nutr 2017;68:249-55.
139. Jiang S, Liu H, Li C. Dietary regulation of oxidative stress in chronic metabolic diseases. Foods 2021;10:1854.
140. Tammen SA, Friso S, Choi SW. Epigenetics: the link between nature and nurture. Mol Aspects Med 2013;34:753-64.
141. Ho DH. Transgenerational epigenetics: the role of maternal effects in cardiovascular development. Integr Comp Biol 2014;54:43-51.
142. Cooper WN, Khulan B, Owens S, et al. DNA methylation profiling at imprinted loci after periconceptional micronutrient supplementation in humans: results of a pilot randomized controlled trial. FASEB J 2012;26:1782-90.
143. Zhang W, Song M, Qu J, Liu GH. Epigenetic modifications in cardiovascular aging and diseases. Circ Res 2018;123:773-86.
144. Prasher D, Greenway SC, Singh RB. The impact of epigenetics on cardiovascular disease. Biochem Cell Biol 2020;98:12-22.
145. Cao Y, Lu L, Liu M, et al. Impact of epigenetics in the management of cardiovascular disease: a review. Eur Rev Med Pharmacol Sci 2014;18:3097-104.
146. Jones PA. Functions of DNA methylation: islands, start sites, gene bodies and beyond. Nat Rev Genet 2012;13:484-92.
147. Shi Y, Zhang H, Huang S, et al. Epigenetic regulation in cardiovascular disease: mechanisms and advances in clinical trials. Signal Transduct Target Ther 2022;7:200.
148. Tabaei S, Tabaee SS. DNA methylation abnormalities in atherosclerosis. Artif Cells Nanomed Biotechnol 2019;47:2031-41.
149. Horvath S, Raj K. DNA methylation-based biomarkers and the epigenetic clock theory of ageing. Nat Rev Genet 2018;19:371-84.
150. Yamada Y, Horibe H, Oguri M, et al. Identification of novel hyper- or hypomethylated CpG sites and genes associated with atherosclerotic plaque using an epigenome-wide association study. Int J Mol Med 2018;41:2724-32.
151. Zhang Y, Zeng C. Role of DNA methylation in cardiovascular diseases. Clin Exp Hypertens 2016;38:261-7.
152. Han P, Li W, Yang J, et al. Epigenetic response to environmental stress: assembly of BRG1-G9a/GLP-DNMT3 repressive chromatin complex on Myh6 promoter in pathologically stressed hearts. Biochim Biophys Acta 2016;1863:1772-81.
153. Li W, Wang Y, Huang R, et al. Association of lipid metabolism-related gene promoter methylation with risk of coronary artery disease. Mol Biol Rep 2022;49:9373-8.
154. Frazier-Wood AC, Aslibekyan S, Absher DM, et al. Methylation at CPT1A locus is associated with lipoprotein subfraction profiles. J Lipid Res 2014;55:1324-30.
155. Lei H, Hu J, Sun K, Xu D. The role and molecular mechanism of epigenetics in cardiac hypertrophy. Heart Fail Rev 2021;26:1505-14.
156. Gilsbach R, Preissl S, Grüning BA, et al. Dynamic DNA methylation orchestrates cardiomyocyte development, maturation and disease. Nat Commun 2014;5:5288.
157. Zheng Y, Joyce BT, Hwang SJ, et al. Association of cardiovascular health through young adulthood with genome-wide dna methylation patterns in midlife: the cardia study. Circulation 2022;146:94-109.
158. Tessarz P, Kouzarides T. Histone core modifications regulating nucleosome structure and dynamics. Nat Rev Mol Cell Biol 2014;15:703-8.
159. Zhang Y, Sun Z, Jia J, et al. Overview of histone modification. Adv Exp Med Biol 2021;1283:1-16.
160. Tang H, Zeng Z, Shang C, Li Q, Liu J. Epigenetic regulation in pathology of atherosclerosis: a novel perspective. Front Genet 2021;12:810689.
161. Groh L, Keating ST, Joosten LAB, Netea MG, Riksen NP. Monocyte and macrophage immunometabolism in atherosclerosis. Semin Immunopathol 2018;40:203-14.
162. Kim GR, Cho SN, Kim HS, et al. Histone deacetylase and GATA-binding factor 6 regulate arterial remodeling in angiotensin II-induced hypertension. J Hypertens 2016;34:2206-19.
163. Jeong MY, Lin YH, Wennersten SA, et al. Histone deacetylase activity governs diastolic dysfunction through a nongenomic mechanism. Sci Transl Med 2018:2018;10.
164. Zhang QJ, Chen HZ, Wang L, Liu DP, Hill JA, Liu ZP. The histone trimethyllysine demethylase JMJD2A promotes cardiac hypertrophy in response to hypertrophic stimuli in mice. J Clin Invest 2011;121:2447-56.
165. Mushtaq I, Ishtiaq A, Ali T, Jan MI, Murtaza I. An overview of non-coding RNAs and cardiovascular system. Adv Exp Med Biol 2020;1229:3-45.
166. Das S, Shah R, Dimmeler S, et al. Noncoding RNAs in cardiovascular disease: current knowledge, tools and technologies for investigation, and future directions: a scientific statement from the american heart association. Circ Genom Precis Med 2020;13:e000062.
167. Ghebre YT, Yakubov E, Wong WT, et al. Vascular aging: implications for cardiovascular disease and therapy. Transl Med 2016;6:183.
168. Wang J, Yang X. The function of miRNA in cardiac hypertrophy. Cell Mol Life Sci 2012;69:3561-70.
170. Huang ZP, Chen J, Seok HY, et al. MicroRNA-22 regulates cardiac hypertrophy and remodeling in response to stress. Circ Res 2013;112:1234-43.
171. Amenyah SD, Ward M, Strain JJ, et al. Nutritional epigenomics and age-related disease. Curr Dev Nutr 2020;4:nzaa097.
172. Parrillo L, Costa V, Raciti GA, et al. Hoxa5 undergoes dynamic DNA methylation and transcriptional repression in the adipose tissue of mice exposed to high-fat diet. Int J Obes 2016;40:929-37.
173. Ciccarone F, Castelli S, Ioannilli L, Ciriolo MR. High dietary fat intake affects DNA methylation/hydroxymethylation in mouse heart: epigenetic hints for obesity-related cardiac dysfunction. Mol Nutr Food Res 2019;63:e1800970.
174. Jacobsen SC, Brøns C, Bork-Jensen J, et al. Effects of short-term high-fat overfeeding on genome-wide DNA methylation in the skeletal muscle of healthy young men. Diabetologia 2012;55:3341-9.
175. Perfilyev A, Dahlman I, Gillberg L, et al. Impact of polyunsaturated and saturated fat overfeeding on the DNA-methylation pattern in human adipose tissue: a randomized controlled trial. Am J Clin Nutr 2017;105:991-1000.
176. Osaka M, Deushi M, Aoyama J, Funakoshi T, Ishigami A, Yoshida M. High-fat diet enhances neutrophil adhesion in LDLR-null mice via hypercitrullination of histone H3. JACC Basic Transl Sci 2021;6:507-23.
177. Jung JK, Yoon GE, Jang G, Park KM, Kim I, Kim JI. Inhibition of HDACs (histone deacetylases) ameliorates high-fat diet-induced hypertension through restoration of the MsrA (methionine sulfoxide reductase a)/hydrogen sulfide axis. Hypertension 2021;78:1103-15.
178. Guedes EC, França GS, Lino CA, et al. MicroRNA expression signature is altered in the cardiac remodeling induced by high fat diets. J Cell Physiol 2016;231:1771-83.
179. Dasinger JH, Alsheikh AJ, Abais-Battad JM, et al. Epigenetic modifications in T cells: the role of dna methylation in salt-sensitive hypertension. Hypertension 2020;75:372-82.
180. Gao P, You M, Li L, et al. Salt-induced hepatic inflammatory memory contributes to cardiovascular damage through epigenetic modulation of SIRT3. Circulation 2022;145:375-91.
181. Amara VR, Surapaneni SK, Tikoo K. Dysregulation of microRNAs and renin-angiotensin system in high salt diet-induced cardiac dysfunction in uninephrectomized rats. PLoS One 2017;12:e0180490.
182. Kidambi S, Pan X, Yang C, et al. Dietary sodium restriction results in tissue-specific changes in dna methylation in humans. Hypertension 2021;78:434-46.
183. Chen L, He FJ, Dong Y, Huang Y, Harshfield GA, Zhu H. Sodium reduction, miRNA profiling and CVD risk in untreated hypertensives: a randomized, double-blind, placebo-controlled trial. Sci Rep 2018;8:12729.
184. Ma J, Rebholz CM, Braun KVE, et al. Whole blood DNA methylation signatures of diet are associated with cardiovascular disease risk factors and all-cause mortality. Circ Genom Precis Med 2020;13:e002766.
185. Kim Y, Huan T, Joehanes R, et al. Higher diet quality relates to decelerated epigenetic aging. Am J Clin Nutr 2022;115:163-70.
186. Do WL, Whitsel EA, Costeira R, et al. Epigenome-wide association study of diet quality in the Women’s Health Initiative and TwinsUK cohort. Int J Epidemiol 2021;50:675-84.
187. Arpón A, Riezu-Boj JI, Milagro FI, et al. Adherence to mediterranean diet is associated with methylation changes in inflammation-related genes in peripheral blood cells. J Physiol Biochem 2016;73:445-55.
188. Yubero-Serrano EM, Fernandez-Gandara C, Garcia-Rios A, et al. Mediterranean diet and endothelial function in patients with coronary heart disease: An analysis of the CORDIOPREV randomized controlled trial. PLoS Med 2020;17:e1003282.
189. Marques-Rocha JL, Milagro FI, Mansego ML, Zulet MA, Bressan J, Martínez JA. Expression of inflammation-related miRNAs in white blood cells from subjects with metabolic syndrome after 8 wk of following a Mediterranean diet-based weight loss program. Nutrition 2016;32:48-55.
190. Arpón A, Milagro FI, Razquin C, et al. Impact of consuming extra-virgin olive oil or nuts within a mediterranean diet on DNA methylation in peripheral white blood cells within the PREDIMED-navarra randomized controlled trial: a role for dietary lipids. Nutrients 2017;10:15.
191. Daimiel L, Micó V, Valls RM, et al. Impact of phenol-enriched virgin olive oils on the postprandial levels of circulating micrornas related to cardiovascular disease. Mol Nutr Food Res 2020;64:e2000049.
192. Fabiani R, Vella N, Rosignoli P. Epigenetic modifications induced by olive oil and its phenolic compounds: a systematic review. Molecules 2021;26:273.
193. Ramos-Lopez O, Milagro FI, Riezu-Boj JI, Martinez JA. Epigenetic signatures underlying inflammation: an interplay of nutrition, physical activity, metabolic diseases, and environmental factors for personalized nutrition. Inflamm Res 2021;70:29-49.
194. Castañer O, Corella D, Covas MI, et al. In vivo transcriptomic profile after a Mediterranean diet in high-cardiovascular risk patients: a randomized controlled trial. Am J Clin Nutr 2013;98:845-53.
195. Mattson MP, Longo VD, Harvie M. Impact of intermittent fasting on health and disease processes. Ageing Res Rev 2017;39:46-58.
196. Ruan HB, Crawford PA. Ketone bodies as epigenetic modifiers. Curr Opin Clin Nutr Metab Care 2018;21:260-6.
197. Asif S, Morrow NM, Mulvihill EE, Kim KH. Understanding Dietary Intervention-Mediated Epigenetic Modifications in Metabolic Diseases. Front Genet 2020;11:590369.
Cite This Article

How to Cite
Download Citation
Export Citation File:
Type of Import
Tips on Downloading Citation
Citation Manager File Format
Type of Import
Direct Import: When the Direct Import option is selected (the default state), a dialogue box will give you the option to Save or Open the downloaded citation data. Choosing Open will either launch your citation manager or give you a choice of applications with which to use the metadata. The Save option saves the file locally for later use.
Indirect Import: When the Indirect Import option is selected, the metadata is displayed and may be copied and pasted as needed.
About This Article
Copyright
Data & Comments
Data
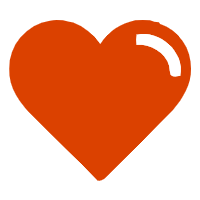
Comments
Comments must be written in English. Spam, offensive content, impersonation, and private information will not be permitted. If any comment is reported and identified as inappropriate content by OAE staff, the comment will be removed without notice. If you have any queries or need any help, please contact us at [email protected].