Constructing neural-like network channels from the surface to interface for enhanced high-rate cycling stability in Co-free Li-rich cathode
Abstract
Co-free Li-rich Mn-based cathode materials (LMNO) have gradually become powerful competitors with ultra-high specific discharge capacity and energy density. However, high-rate performance and severe voltage decay restrict the commercial application of LMNO. Herein, LiAl5O8 acts as a templating agent to construct 3D neural-like networks in LMNO, enabling fast ion diffusion and improving rate performance. Proton exchange is predominantly facilitated by the process of LiAl5O8 constructed to generate vacancies for oxygen preservation, while strong Al-O bonds stabilize interfacial lattice oxygen, effectively suppressing voltage decay due to structural evolution. As a result, the designed cathode exhibits a discharge specific capacity of 154.65 mAh g-1 at 5 C and 91.68% capacity retention after 400 cycles (vs. 66.67% of LMNO), effectively suppressing voltage decay with 90.90% voltage retention (vs. 81.08% of LMNO). The constructed neural-like network structure engineering provides an innovative direction for improving the high-rate performance and structural stability of LMNO.
Keywords
INTRODUCTION
The rapid development of power vehicles has put forward high requirements for energy density, security and production cost of lithium-ion batteries (LIBs)[1-4]. Therefore, it is critical to explore higher energy density cathode materials[5-8]. Among the cathode materials for LIBs, Li-rich Mn-based oxide cathodes (LLOs), xLi2MnO3·(1-x)LiTMO2 (0 < x < 1, TM = Mn, Ni, Co, etc.), have received extensive attention because of their high specific capacity, low cost and high voltage[9-11]. Nevertheless, structural instability by transition metal migration and irreversible oxygen release of LLOs during charging/discharging cycles, particularly poor rate performance, results in impeding its practical application[12-14]. These problems can be explained by mechanisms that have been experimentally demonstrated. Firstly, the redox of oxygen and more interfacial side reactions at high voltages cause excessive consumption of Li[15-17]. Secondly, continuous oxygen release and structure collapse in LLOs lead to performance degradation, particularly the characteristic voltage fading[18-20]. Eventually, the inherent limitation of Li+ migration kinetics in LLOs is the primary reason for their inability to meet rate performance requirements[21,22]. Therefore, it is imperative to conquer these challenges and upgrade the performance of LLOs to meet the high-performance requirements of power vehicles and enhance the overall performance.
To tackle the aforementioned challenges, various strategies have been explored, such as inactive element doping[23-26], structural induction[27-29], and surface engineering[30-32]. However, the effectiveness of doping alone is often limited, as it overlooks material surface side reactions that can hinder achieving higher cycle stability. Consequently, many researchers are inclined towards structural induction or surface engineering techniques to enhance the performance of LLOs. For instance, Yang et al. developed twin structures with a 3D Li+ diffusion channel, which not only improves the rate performance but also effectively suppresses the migration of manganese ions and voltage decay[16]. The downside is that the potential instability of twins resulting from internal stress anisotropy is not taken into consideration[16]. Zheng et al. utilized Li+/Ni2+ antisite defects and surface-induced spinel phases in LLOs to enhance Li+ diffusion on the surface and reduce the formation energy of oxygen vacancies, ultimately improving the rate performance and lattice oxygen stability[33]. Nevertheless, it is not enough to focus only on one-sided ion diffusion. Due to synergistic lithium-ion diffusion on the surface/bulk and structural stability, predominantly driven by subtle structural inductions and surface engineering, mainly contributes to the rate performance and overall stability of LLOs. It is well known that LiAl5O8 is an inverse spinel structure with stress equalization and a 3D Li+ transport channel; thus, it exhibits both structural stability and a high rate of Li+ diffusion[34-36]. Meanwhile, Al can alter the Li@Mn6 superstructure units to heighten oxygen reversibility and reduce phase transitions during long cycling, and stronger Al-O bonds will improve lattice oxygen stability and transition metals migration barriers to suppress voltage decay[23,37,38].
Herein, we proposed an overall strategy to construct a neural-like network structure at the grain boundaries of primary particles and the surface of materials with LiAl5O8. The initial cathode [Co-free Li-rich Mn-based cathode materials (LMNO)] is immersed into Al(NO3)3 solution for ultrasound-induced osmotic reaction, and the Al in solution will react with Li in LMNO to form LiAl5O8 at high temperature. With the gradual penetration of LiAl5O8 into the bulk of LMNO, a neural network-like structure of LiAl5O8 is formed, effectively enhancing the overall Li+ diffusion rate and rate performance of LMNO. Meanwhile, the introduction of Al into the lattice forms strong Al-O bonds, which stabilizes interfacial lattice oxygen and effectively prevents voltage decay caused by structural evolution. The designed cathode LMNO-A shows a specific capacity of 281.55 mAh g-1 at 0.1 C (1 C = 250 mA g-1) and 154.65 mAh g-1 at 5 C. After 400 cycles at 5 C, it demonstrates a capacity retention of 91.68% and a voltage retention of 90.90%. Moreover, the in-situ electrochemical impedance spectroscopy (EIS) test shows the average Li+ diffusion rate of LMNO-A during the first charge/discharge is 3.10 × 10-16 cm2 s-1, which is higher than 1.22 × 10-16 cm2 s-1 of LMNO. This work provides a simple and reproducible modification strategy for improving the high-rate performance and significantly suppressing voltage degradation of the LMNO cathode.
EXPERIMENTAL
Materials preparation
Mn0.75Ni0.25CO3 precursors were obtained by co-precipitation synthesis. The corresponding amounts of MnSO4·H2O and NiSO4·6H2O were calculated according to the chemical expressions of precursors and added to 500 mL of deionized water to obtain a 2 M solution. Na2CO3 was added to 500 mL of deionized water to obtain a 2 M solution as precipitant. NH3·H2O was added to 300 mL of deionized water to obtain 0.2 M solution as a complexing agent. The three solutions were pumped into the continuously stirred tank reactor, and the stirring speed was controlled at 800 r min-1 and the reaction temperature was 55 °C. The pH values of the nucleation, growth and aging stages were controlled at 7.8/8.0/8.2, respectively. The
The dried precursor and Li2CO3 were mixed and fully ground according to the molar ratio of 1:1.2 (5% excess). The mixture was then placed in a muffle furnace, pre-sintered for 5 h at 500 °C, and sintered for
To modify the LMNO particles, Al(NO3)3·9H2O and LMNO were dissolved in deionized water at molar ratios of Al to transition metal (TM: Mn and Ni) of 3:100, 5:100, and 20:100, respectively. The mixture was then treated with ultrasonic waves for 30 min to ensure uniform dispersion. Afterward, the modified particles were washed three times with deionized water and dried in a vacuum oven at 120 °C to obtain the precursor. Finally, the precursor was annealed in a muffle furnace at 750 °C for 5 h with a heating rate of
Additional experimental details on the structural characterization and electrochemical properties of the electrode materials are shown in the Supplementary Material.
RESULTS AND DISCUSSION
Neural-like network formed by LiAl5O8 permeation growth contributes to rapid Li+ migration. LMNO-A is formed through impregnation treatment and sintering of LMNO, as shown in Figure 1A. LiAl5O8 is produced on the surface and then extends along the grain boundaries of the primary particles, gradually penetrating the interior to create protected structures that resemble a neural-like network. Specifically,
Figure 1. (A) The schematic of the synthesis of LMNO and LMNO-A. (B) The structural model of LiAl5O8 and Li+ diffusion path in
Scanning electron microscopy (SEM) was utilized to examine the surface morphology of LMNO
Figure 2. The SEM images of (A) LMNO and (B) LMNO-A. The EDS images of (C) inside and (D) surface of LMNO-A. (E) The schematic of the structure inside LMNO and LMNO-A particles. (F) The cross-section SEM images of LMNO-A. The line scan at (G) surface and (H) inside of LMNO-A and the specific relative content (I) of Mn, Ni and Al. (J) The TEM images of LMNO-A.
The introduction of LiAl5O8 helps to increase the oxygen vacancies in the structure and inhibit irreversible oxygen release. From the structure perspective, the crystal structures of LMNO and LMNO-A were characterized by XRD and the results are shown in Figure 3A. All the peaks belong to the hexagonal
Figure 3. (A) The XRD patterns of LMNO and LMNO-A. The Rietveld refinement plots of (B) LMNO and (C) LMNO-A. The XPS spectra of (D) Al 2p and (E) O 1s. (F) The EPR spectrum of LMNO and LMNO-A. The XPS spectra of (G) Ni 2p and (H) Mn 2p. (I) The specific relative content of Mn4+, Ni2+ and lattice oxygen.
In conclusion, the aforementioned results are favorable for inhibiting the irreversible release of lattice oxygen caused by the over-activation of the Li2MnO3 phase and improving the structural stability during cycling and the ICE of the electrode material.
Electrochemical properties. Assembled button half-cells with the LMNO and LMNO-A as the cathode were activated in the voltage range of 2~4.8 V at 0.1 C (1 C = 250 mA g-1) as shown in Figure 4A. Both samples show the characteristic curve of LLO cathode materials, which included a continuous slope below 4.5 V associated with the oxidation of Ni2+/Ni4+, and a long charging plateau above 4.45 V related to the activation of the Li2MnO3 phase, where O2- is oxidized to O(2-n)- (0 < n < 2)[54-56]. The shorter charging plateau observed at 4.45 V in LMNO-A indicates a reduced activation of the Li2MnO3 phase. LMNO-A exhibits a reversible capacity of 281.55 mAh g-1, compared to LMNO’s reversible capacity of 268.74 mAh g-1, with an ICE of 80.85%. The dQ/dV curves of the two samples [Figure 4B] show a significant decrease in the oxidation peak intensity of LMNO-A at around 4.5 V. This indicates that the oxygen oxidation reaction is suppressed, thereby effectively reducing irreversible oxygen release and contributing to improved structural stability and cycling performance of the material[40,57]. Compared to LMNO, the reduction peak potential corresponding to Mn4+/3+ around 3.4 V is lower for LMNO-A, which can be attributed to an increased relative content of Mn3+[57]. Moreover, LMNO-A achieves a specific capacity of 259.86 mAh g-1 at 0.2 C and an energy density of 861.74 Wh kg-1 [Figure 4C]. Especially, the voltage decay of LMNO-A is only 2.21 mV per cycle, whereas LNMO experiences an undesired voltage decay of up to 4.81 mV per cycle [Figure 4D and
Figure 4. (A) The initial activation curve of LMNO and LMNO-A. (B) The dQ/dV curves of LMNO and LMNO-A. (C) The specific capacity and energy density of LMNO and LMNO-A. (D) The electrochemical behavior of LMNO-A at 0.2 C. (E) The cycling performance of LMNO and LMNO-A in the voltage range of 2~4.8 V at 1 C. (F) The Normalized discharge curves at 1 C. The corresponding dQ/dV curve of (G) LMNO and (H) LMNO-A. (I) The cycling performance of LMNO and LMNO-A in the voltage range of 2~4.8 V at 5 C. (J) The discharge curves and corresponding dQ/dV curves of 400 cycles of LMNO-A.
The aforementioned results indicate that the inclusion of LiAl5O8 in the system reduces interfacial side reactions, which improves the ICE and discharge specific capacity of LMNO-A. Meanwhile, the neural-like network structure constructed by LiAl5O8 on the surface extends to the interior of LMNO-A particles. It facilitates rapid diffusion of Li+ inside the electrode material, thereby significantly improving the high-rate performance. Furthermore, the presence of this network structure effectively suppresses the structural evolution of the electrode, thereby inhibiting voltage decay, particularly under high-rate conditions.
The effect of neural-like network structure on the kinetics was analyzed using in situ EIS, Cyclic Voltammetry (CV), and Galvanostatic Intermittent Titration Technique (GITT). The EIS analysis was conducted on the two samples at various charge states within the voltage range of 2~4.8 V, using a rate of 0.2 C. The EIS data is then fitted using an equivalent circuit (refer to Supplementary Figure 8), and the specific values obtained are presented in Supplementary Table 3. The internal resistance of the solution (Rs) is represented by the intercept of the curve with the solid part in the high-frequency region. It is mainly related to the electronic conductivity of the cathode and the ionic conductivity of the electrolyte adsorbed on the electrode, representing the resistance of cathode electrolyte interface (CEI). The charge transfer impedance (Rct) represents the resistance of the charge transfer process in the medium to high frequency region; the diffusion impedance of Li+ (W) in the low frequency region represents the diffusion polarization of Li+ in the electrode; CPE1 indicates the capacitive components associated[18,59]. The results indicate that LMNO-A [Figure 5A] and LMNO [Figure 5B] display a similar trend of change, with minimal variation observed in the Rs throughout the process. As a result, the analysis primarily focuses on examining the changes in the Rct and the W. Notably, the Rct exhibits almost no change from the initial stage of charging up to 3.6 V. This can be attributed to the fact that the voltage required for cation/anion oxidation has not been reached at this stage, and Li+ is not yet being extracted from the cathode. On the contrary, as the charging progresses to approximately 3.8 V, the rapid extraction of Li+ results in a gradual reduction of Rct. As the charging voltage increases to 4.6 V, the oxidation of fewer lattice O atoms results in a smaller range of the Rct variation[25,60]. The Rct steadily rises as Li+ ions are re-embedded into the cathode throughout the discharge process. Clearly, the Rct of LMNO remains high when discharged to 2.0 V, which may be because part of the On- cannot be reduced to O2- or part of the O2- has been oxidized to O2 irreversibly released. In contrast, LMNO-A exhibits significantly smaller Rct throughout the entire process, indicating that the modified LMNO-A possesses a more abundant lithium-ion diffusion pathway and better ionic conductivity. The diffusion impedance of Li+ (DLi+) can be represented by the curve of ω-1/2 and Z′
Figure 5. The in-situ EIS results of (A) LMNO-A and (B) LMNO in the first cycle and second charge. (C) The value of DLi+ and Rct of LMNO-A and LMNO in the whole process. (D)The GITT curves and the Li+ diffusion coefficient of LMNO-A and LMNO. The (E) CV curves and (F) Li+ diffusion coefficient for different scan rates of LMNO-A. (G)The in situ XRD patterns about the (003) peak of LMNO-A and LMNO during the first cycle. (H) The changes of c during the first cycle and (I) the corresponding parameter comparisons.
Moreover, the GITT results obtained for LMNO and LMNO-A at a rate of 0.1 C [Figure 5D and
Superior initial capacity rendering and lower voltage decay during cycling are associated with a stable crystal structure. In order to explore the structural evolution during the initial charging/discharging process, in-situ XRD was conducted to explore the variation of the (003) and (101) peaks of LMNO-A and LMNO during initial charging/discharging [Figure 5G, Supplementary Figure 10A and B]. The results show that the two samples exhibit the same trend. During charging, Li+ is consistently removed from the cathode. This can be attributed to the increased electrostatic repulsion between the neighboring oxygen layers, which leads to an expansion of the layer spacing[28]. Consequently, this expansion causes a lower-angle shift of the (003) peak during the discharging process, and the Li+ is embedded back into the cathode, causing a higher-angle shift of the (003) peak as well as the (101) peak. The shifts in the positions of the (003) and (101) peaks of LMNO-A during this process are significantly smaller, which indicates better structural reversibility. However, the LMNO peak intensity is significantly lower and the full width at half maximum (FWHM) is larger at the high voltage range, which signaled a more severe decline of the layer structure[61]. We conduct a refined analysis of the in-situ XRD results, as shown in Figure 5H and I, Supplementary Figure 10C and D. Following the initial charging/discharging process, the changes observed in the c-axis and a-axis of LMNO-A are only 1.24 × 10-3 and 1.45 × 10-3 Å, respectively. In comparison, the changes in the c-axis and a-axis of LMNO are 1.42 × 10-2 and 1.77 × 10-3 Å, respectively. Furthermore, the maximum changes observed in the c-axis and a-axis of LMNO-A are merely 7.01 × 10-2 and 4.97 × 10-3 Å, while for LMNO, they are 9.8 × 10-2 and 5.07 × 10-3 Å. The above results suggest that the neural-like network structure facilitated by LiAl5O8 not only improves the Li+ diffusion rate but also mitigates the structural evolution of LMNO-A to some extent during the charging/discharging process. Additionally, the neural-like network structure exhibits better preservation of the layer structure, particularly at high voltages. This enhances the structural stability of the electrode during the cycling process.
To further explore the advantages of the modified material LMNO-A, we systematically compare the material characteristics after cycling. After 400 cycles at 5 C, the SEM images of the LMNO cathode reveal that most secondary particles have developed significant cracks [Figure 6A]. These cracks primarily result from stress accumulation caused by volume changes during cycling. Such structural degradation exacerbates side reactions between the cathode and the electrolyte, further accelerating material corrosion and fracture, which in turn leads to rapid capacity and voltage decay. In contrast, thanks to the presence of the LiAl5O8 neural-like network, the secondary particles of LMNO-A maintain high structural integrity under the same cycling conditions [Figure 6B]. LiAl5O8 fills the gaps between primary particles, effectively suppressing crack propagation and electrolyte corrosion, thereby endowing the material with excellent structural stability. The TEM images [Supplementary Figure 11] show that the thickness of the CEI layer on the surface of the pristine LMNO material is approximately 8 nm. This indicates that severe interfacial side reactions occur between the LMNO electrode and the electrolyte during high-rate cycling, leading to continuous thickening of the CEI layer. In contrast, for the LMNO-A material, the surface-modified LiAl5O8 layer acts as a protective barrier, effectively suppressing interfacial side reactions. As a result, the thickness of the CEI layer on LMNO-A is only 2 nm. This result demonstrates the significant role of the LiAl5O8 modification in stabilizing the interface structure and enhancing the cycling performance of the material. Moreover, the XRD tests conducted on the electrode after cycling reveal notable differences between LMNO-A and LMNO. Specifically, the intensity of the (003), (101), and (104) peaks in LMNO-A is significantly higher compared to LMNO [Figure 6C]. Additionally, the enlarged (108/110) diffraction peaks indicate a more pronounced peak splitting, reflecting better preservation of the layer structure in LMNO-A[31]. More importantly, upon disassembling the cycled battery, a notable observation is the reduced presence of black material surrounding the LMNO-A electrode. XPS tests were performed on the cycled electrodes, revealing distinct Mn characteristic signals in the Mn 2p spectra of LMNO-A. In contrast, the Mn 2p signal intensity of LMNO is significantly weaker [Supplementary Figure 12A]. To further quantify this effect, inductively coupled plasma optical emission spectroscopy (ICP-OES) was employed to measure the dissolution of Mn ions into the electrolyte for both cycled LMNO-A and LMNO electrodes. The ICP-OES results
Figure 6. The SEM images of (A) LMNO and (B) LMNO-A after 400 cycles at 5 C. (C) The XRD patterns of LMNO and LMNO-A after cycles. The XPS spectra of (D) F 1s and (E) O 1s of LMNO and LMNO-A after cycles. The (F) EIS results of LMNO and LMNO-A after cycles at different charge states, (G) the value of Rct and (H) σ.
CONCLUSIONS
In conclusion, the neural-like network structure strategy has successfully improved the electrochemical performance of Co-free Li-rich Mn-based cathodes. Different from other modification methods, the incorporation of LiAl5O8 not only covers the surface of LMNO-A particles but also gradually extends to the interior grain boundaries, forming a comprehensive neural-like network structure throughout the cathode. On the one hand, abundant 3D Li+ diffusion channels are provided at the internal interfaces and surfaces from the kinetic point of view, which significantly improves the high-rate performance of the electrode materials. On the other hand, less interfacial side reactions and high lattice oxygen stability, suppress the structural evolution, significantly reduce voltage decay, and improve the structural stability of electrodes. Therefore, the designed LMNO-A cathode has excellent capacity retention/voltage retention (91.68%/90.90%) at 5 C. Currently, all-solid-state batteries require cathodes that match safer and better interfacial contact, and this strategy improves the ionic conductivity of Li-rich cathodes, providing a new way to solve the interfacial contact problem and offering new possibilities for Li-rich commercialization.
DECLARATIONS
Authors’ contributions
Methodology and formal analysis: Cheng, Y.; Yang, C.; Wang, Y.; Tong, Q.; Ge, Y.; Zhu, J.
Writing - original draft: Yang, C.; Wang, Y.
Data analysis and technical support: Yang, C.; Wang, Y.; Tong, Q.; Ge, Y.; Zhu, J.; Jiang, Y.
Data acquisition: Yang, C.; Wang, Y.; Tong, Q.; Jiang, Y.
Supervision, writing - review and editing: Cheng, Y.; Ge, Y.; Sun, G.; Tian, B.; Wang, Z.; Yu, Z.
Resources, Project administration, Funding acquisition: Cheng, Y.; Sun, G.; Wang, Z.; Yu, Z.
Investigation: Cheng, Y.; Yang, C.; Wang, Y.; Zhu, J.; Jiang, Y.; Tian, B.; Wang, Z.; Yu, Z.
Availability of data and materials
The data supporting our findings can be found in the Supplementary Material.
Financial support and sponsorship
This study was financially supported by the Guangxi Natural Science Foundation (Grant Nos. 2024GXNSFFA010002, 2023GXNSFAA026358 and 2021AB17045), Guangxi Key Laboratory of Manufacturing Systems Foundation (Grant No. 23354S008), Engineering Research Center Foundation of Electronic Information Materials and Devices (Grant No. EIMD-AA202005), the National Key Research and Development Program of China (2023YFB2406100), the National Natural Science Foundation of China (Grant Nos. 22075062 and U23A20573), Heilongjiang Touyan Team (Grant No. HITTY-20190033), and the Fundamental Research Funds for the Central Universities (Grant No. FRFCU5710051922).
Conflicts of interest
All authors declared that there are no conflicts of interest.
Ethical approval and consent to participate
Not applicable.
Consent for publication
Not applicable.
Copyright
© The Author(s) 2025.
Supplementary Materials
REFERENCES
2. He, W.; Zhuang, Y.; Mei, J.; et al. In situ induced lattice-matched interfacial oxygen-passivation-layer endowing Li-rich and Mn-based cathodes with ultralong life. Small 2022, 18, e2200942.
3. Yang, X.; Wang, S.; Han, D.; et al. Structural origin of suppressed voltage decay in single-crystalline Li-rich layered
4. Yu, Z.; Lu, Q.; Wang, Y.; et al. Self-compacting engineering to achieve high-performance lithium-rich layered oxides cathode materials. Appl. Surf. Sci. 2023, 619, 156683.
5. Yu, Z.; Yu, K.; Ji, F.; et al. Enhancing the cycling stability of a hollow architecture Li-rich cathode via Ce-integrated surface/interface/doping engineering. Inorg. Chem. Front. 2023, 10, 682-91.
6. Wang, T.; Zeng, W.; Zhu, J.; et al. SeO2-infused grain boundaries effectively improve rate and stability performance of Li-rich manganese-based layered cathode materials. Nano. Energy. 2023, 113, 108577.
7. Xu, Z.; Guo, X.; Wang, J.; et al. Restraining the octahedron collapse in lithium and manganese rich NCM cathode toward suppressing structure transformation. Adv. Energy. Mater. 2022, 12, 2201323.
8. Liang, C.; Cheng, Y.; Lv, C.; et al. Surface oxygen-locked LiNi0.6Mn0.4O2: towards stable cycling at 4.7 V. Energy. Storage. Mater. 2025, 75, 104087.
9. Assat, G.; Foix, D.; Delacourt, C.; Iadecola, A.; Dedryvère, R.; Tarascon, J. M. Fundamental interplay between anionic/cationic redox governing the kinetics and thermodynamics of lithium-rich cathodes. Nat. Commun. 2017, 8, 2219.
10. Sun, Z.; Xu, L.; Dong, C.; et al. A facile gaseous sulfur treatment strategy for Li-rich and Ni-rich cathode materials with high cycling and rate performance. Nano. Energy. 2019, 63, 103887.
11. Hao, Y.; Li, X.; Liu, W.; et al. Interfacial Mn vacancy for Li-rich Mn-based oxide cathodes. ACS. Appl. Mater. Interfaces. 2022, 14, 22161-9.
12. He, J.; Ma, H.; Zhang, H.; et al. Promoting the electrochemical performance of Li-rich layered Li1.2(Ni1/6Co1/6Mn4/6)0.8O2 with the in situ transformed allogenic spinel phase. ACS. Sustain. Chem. Eng. 2020, 8, 2215-25.
13. Yang, C.; Wang, H.; Wei, Z.; et al. One-step simultaneous construction of oxygen vacancies and Mo-O bonds to enhance the cyclic stability of lithium-rich manganese-based layered oxides. J. Alloys. Compd. 2025, 1010, 178267.
14. Wang, K.; Qiu, J.; Hou, F.; et al. Unraveling the role of surficial oxygen vacancies in stabilizing Li-rich layered oxides. Adv. Energy. Mater. 2023, 13, 2301216.
15. Guo, W.; Zhang, Y.; Lin, L.; et al. Enhancing cycling stability in Li-rich Mn-based cathode materials by solid-liquid-gas integrated interface engineering. Nano. Energy. 2022, 97, 107201.
16. Yang, Y.; Gao, C.; Luo, T.; et al. Unlocking the potential of Li-rich Mn-based oxides for high-rate rechargeable lithium-ion batteries. Adv. Mater. 2023, 35, e2307138.
17. Li, X.; Yu, F.; Ke, W.; et al. Modulating local electronic structure enhances superior electrochemical activity in Li-rich oxide cathodes. J. Mater. Chem. A. 2023, 11, 2252-61.
18. Kim, S. Y.; Park, C. S.; Hosseini, S.; Lampert, J.; Kim, Y. J.; Nazar, L. F. Inhibiting oxygen release from Li-rich, Mn-rich layered oxides at the surface with a solution processable oxygen scavenger polymer. Adv. Energy. Mater. 2021, 11, 2100552.
19. Wen, X.; Yin, C.; Qiu, B.; et al. Controls of oxygen-partial pressure to accelerate the electrochemical activation in Co-free Li-rich layered oxide cathodes. J. Power. Sources. 2022, 523, 231022.
20. Jiang, W.; Zhang, C.; Feng, Y.; et al. Achieving high structure and voltage stability in cobalt-free Li-rich layered oxide cathodes via selective dual-cation doping. Energy. Storage. Mater. 2020, 32, 37-45.
21. Feng, Z.; Song, H.; Li, Y.; Lyu, Y.; Xiao, D.; Guo, B. Adjusting oxygen redox reaction and structural stability of Li- and Mn-rich cathodes by Zr-Ti dual-doping. ACS. Appl. Mater. Interfaces. 2022, 14, 5308-17.
22. Su, Y.; Zhao, J.; Dong, J.; et al. Atomic pins bridging integrated surface to assist high-rate stability for Co-free Li-rich cathode. Chem. Eng. J. 2023, 475, 145991.
23. Li, S.; Yang, L.; Liu, Z.; et al. Surface Al-doping for compromise between facilitating oxygen redox and enhancing structural stability of Li-rich layered oxide. Energy. Storage. Mater. 2023, 55, 356-63.
24. Zhang, K.; Sheng, H.; Wu, X.; et al. Improving electrochemical properties by sodium doping for lithium-rich layered oxides. ACS. Appl. Energy. Mater. 2020, 3, 8953-9.
25. Cheng, W.; Ding, J.; Liu, Z.; et al. Zn/Ti dual concentration-gradients surface doping to improve the stability and kinetics for Li-rich layered oxides cathode. Chem. Eng. J. 2023, 451, 138678.
26. Wang, E.; Xiao, D.; Wu, T.; et al. Al/Ti synergistic doping enhanced cycle stability of Li-rich layered oxides. Adv. Funct. Mater. 2022, 32, 2201744.
27. Luo, D.; Ding, X.; Fan, J.; et al. Accurate control of initial coulombic efficiency for lithium-rich manganese-based layered oxides by surface multicomponent integration. Angew. Chem. Int. Ed. 2020, 59, 23061-6.
28. Tan, Z.; Li, Y.; Xi, X.; et al. A novelty strategy induced pinning effect and defect structure in Ni-rich layered cathodes towards boosting its electrochemical performance. J. Energy. Chem. 2022, 72, 570-80.
29. Marie, J. J.; House, R. A.; Rees, G. J.; et al. Trapped O2 and the origin of voltage fade in layered Li-rich cathodes. Nat. Mater. 2024, 23, 818-25.
30. Yan, C.; Shao, Q.; Yao, Z.; et al. Multifunctional surface construction for long-term cycling stability of Li-rich Mn-based layered oxide cathode for Li-ion batteries. Small 2022, 18, e2107910.
31. Zhang, G.; Chen, M.; Li, C.; et al. Surface spinel and interface oxygen vacancies enhanced lithium-rich layered oxides with excellent electrochemical performances. Chem. Eng. J. 2022, 443, 136434.
32. Liu, Y.; Zhu, H.; Zhu, H.; et al. Modulating the surface ligand orientation for stabilized anionic redox in Li-rich oxide cathodes. Adv. Energy. Mater. 2021, 11, 2003479.
33. Zheng, H.; Zhang, C.; Zhang, Y.; et al. Manipulating the local electronic structure in Li-Rich layered cathode towards superior electrochemical performance. Adv. Funct. Mater. 2021, 31, 2100783.
34. Mo, S.; Zhang, B.; Zhang, K.; Li, S.; Pan, F. LiAl5O8 as a potential coating material in lithium-ion batteries: a first principles study. Phys. Chem. Chem. Phys. 2019, 21, 13758-65.
35. Wu, Y.; Lei, D.; Wang, C. The formation of LiAl5O8 nanowires from bulk Li-Al alloy enables dendrite-free Li metal batteries. Mater. Today. Phys. 2021, 18, 100395.
36. Aykol, M.; Kim, S.; Hegde, V. I.; et al. High-throughput computational design of cathode coatings for Li-ion batteries. Nat. Commun. 2016, 7, 13779.
37. Li, Z.; Li, Y.; Zhang, M.; et al. Modifying Li@Mn6 Superstructure units by Al substitution to enhance the long-cycle performance of Co-free Li-rich cathode. Adv. Energy. Mater. 2021, 11, 2101962.
38. Zhang, C.; Wei, B.; Wang, M.; et al. Regulating oxygen covalent electron localization to enhance anionic redox reversibility of lithium-rich layered oxide cathodes. Energy. Storage. Mater. 2022, 46, 512-22.
39. Li, Z.; Tian, F.; Li, Y.; Lei, D.; Wang, C. Zero-strain insertion anode material of lithium-ion batteries. Small 2022, 18, e2204875.
40. Zhang, Y.; Zheng, S.; Meng, C.; et al. A near-surface structure reconfiguration strategy to regulate Mn3+/Mn4+and O2-/O2n- redox for stabilizing lithium-rich oxide cathode. Adv. Funct. Mater. 2023, 33, 2300987.
41. Liu, P.; Zhang, H.; He, W.; et al. Lithium deficiencies engineering in Li-rich layered oxide Li1.098Mn0.533Ni0.113Co0.138O2 for high-stability cathode. J. Am. Chem. Soc. 2019, 141, 10876-82.
42. Rosina, K. J.; Jiang, M.; Zeng, D.; Salager, E.; Best, A. S.; Grey, C. P. Structure of aluminum fluoride coated Li[Li1/9Ni1/3Mn5/9]O2 cathodes for secondary lithium-ion batteries. J. Mater. Chem. 2012, 22, 20602.
43. Yu, R.; Banis, M. N.; Wang, C.; et al. Tailoring bulk Li+ ion diffusion kinetics and surface lattice oxygen activity for high-performance lithium-rich manganese-based layered oxides. Energy. Storage. Mater. 2021, 37, 509-20.
44. Singh, V.; Chakradhar, R.; Rao, J.; Kwak, H. Characterization, EPR and photoluminescence studies of LiAl5O8:Cr phosphors. Solid. State. Sci. 2009, 11, 870-4.
45. Singh, V.; Chakradhar, R.; Rao, J.; Kim, D. EPR and luminescence properties of combustion synthesized LiAl5O8:Mn phosphors. Mater. Chem. Phys. 2008, 110, 43-51.
46. Li, S.; Li, H.; Zhang, H.; Zhang, S.; Lai, Y.; Zhang, Z. Constructing stable surface structures enabling fast charging for Li-rich layered oxide cathodes. Chem. Eng. J. 2022, 427, 132036.
47. Li, Z.; Li, H.; Cao, S.; et al. Reversible anionic redox and spinel-layered coherent structure enable high-capacity and long-term cycling of Li-rich cathode. Chem. Eng. J. 2023, 452, 139041.
48. Cao, J.; Huang, H.; Qu, Y.; Tang, W.; Yang, Z.; Zhang, W. Construction of a hetero-epitaxial nanostructure at the interface of Li-rich cathode materials to boost their rate capability and cycling performances. Nanoscale 2021, 13, 20488-97.
49. Mohapatra, M.; Seshadri, M.; Naik, Y. P.; Meena, G.; Kadam, R. M.; Singh, V. Radiative properties of ‘intense’ red emitting LiAl5O8:Eu phosphors. J. Mater. Sci. Mater. Electron. 2018, 29, 7778-84.
50. Yu, H.; Ibrahim, K. B.; Chi, P.; et al. Modulating the voltage decay and cationic redox kinetics of Li-rich cathodes via controlling the local electronic structure. Adv. Funct. Mater. 2022, 32, 2112394.
51. Xu, G.; Ke, W.; Yu, F.; et al. Modulation of lattice oxygen boosts the electrochemical activity and stability of Co-free Li-rich cathodes. J. Energy. Chem. 2022, 75, 117-26.
52. Zhang, X.; Belharouak, I.; Li, L.; et al. Structural and electrochemical study of Al2O3 and TiO2 coated Li1.2Ni0.13Mn0.54Co0.13O2 cathode material using ALD. Adv. Energy. Mater. 2013, 3, 1299-307.
53. Lu, Q.; Wang, Y.; Yu, K.; Zhao, G.; Cheng, Y.; Yu, Z. One-step constructed oxygen vacancies and Fe-doping to improve the electrochemical performance of Li-rich Mn-based cathode. J. Alloys. Compd. 2023, 937, 168426.
54. Liu, Y.; Chen, Y.; Wang, J.; et al. Hierarchical yolk-shell structured Li-rich cathode boosting cycling and voltage stabled LIBs. Nano. Res. 2022, 15, 3178-86.
55. Zhang, P.; Zhai, X.; Huang, H.; et al. Synergistic Na+ and F- co-doping modification strategy to improve the electrochemical performance of Li-rich Li1·20Mn0·54Ni0·13Co0·13O2 cathode. Ceram. Int. 2020, 46, 24723-36.
56. Liu, Y.; Yang, Z.; Zhong, J.; et al. Surface-functionalized coating for lithium-rich cathode material to achieve ultra-high rate and excellent cycle performance. ACS. Nano. 2019, 13, 11891-900.
57. Liu, J.; Wu, Z.; Yu, M.; et al. Building homogenous Li2TiO3 coating layer on primary particles to stabilize Li-rich Mn-based cathode materials. Small 2022, 18, e2106337.
58. Zhang, B.; Zhang, Y.; Wang, X.; et al. Role of substitution elements in enhancing the structural stability of Li-rich layered cathodes. J. Am. Chem. Soc. 2023, 145, 8700-13.
59. Sun, G.; Yu, F.; Zhao, C.; et al. Decoupling the voltage hysteresis of Li-rich cathodes: electrochemical monitoring, modulation anionic redox chemistry and theoretical verifying. Adv. Funct. Mater. 2021, 31, 2002643.
60. Charbonneau, V.; Lasia, A.; Brisard, G. Impedance studies of Li+ diffusion in nickel manganese cobalt oxide (NMC) during charge/discharge cycles. J. Electroanal. Chem. 2020, 875, 113944.
Cite This Article

How to Cite
Download Citation
Export Citation File:
Type of Import
Tips on Downloading Citation
Citation Manager File Format
Type of Import
Direct Import: When the Direct Import option is selected (the default state), a dialogue box will give you the option to Save or Open the downloaded citation data. Choosing Open will either launch your citation manager or give you a choice of applications with which to use the metadata. The Save option saves the file locally for later use.
Indirect Import: When the Indirect Import option is selected, the metadata is displayed and may be copied and pasted as needed.
About This Article
Copyright
Data & Comments
Data
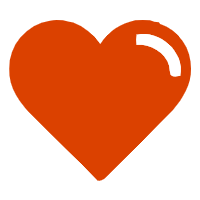
Comments
Comments must be written in English. Spam, offensive content, impersonation, and private information will not be permitted. If any comment is reported and identified as inappropriate content by OAE staff, the comment will be removed without notice. If you have any queries or need any help, please contact us at [email protected].