Three-dimensional MXene coupled CoFe nanoalloys as sulfur host for long-life room-temperature sodium-sulfur batteries
Abstract
Room-temperature sodium-sulfur (RT Na-S) batteries are potential candidates for next-generation energy storage systems because of low-cost resources, high theoretical capacity, and high energy density. However, their commercialization is hindered by the inherent shuttle effect, insulation of sulfur, and slow catalytic conversion. This study proposes a novel approach involving the design of a C/CoFe alloy catalyst coupled with Ti3C2Tx MXene substrate (C/CoFe-MXene) as a three-dimensional porous conductive sulfur host. Polysulfide adsorption/catalytic experiments and density functional theory calculation confirmed the excellent affinity and strong catalytic conversion ability of the C/CoFe-MXene composite for polysulfides. The heterostructure formed between the CoFe alloy and the MXene substrate promotes Na+ transport and accelerates reaction kinetics of sulfur species. Consequently, the assembled RT Na-S batteries with a C/CoFe-MXene sulfur host (2.0 mg cm-2) deliver a high initial specific capacity of 572 mAh g-1 at 1 C. Even at 5 C, the battery achieves ultralong-term cycling over 5,400 cycles with a capacity retention rate of 61.9%, corresponding to a slow capacity fading rate of 0.0089% per cycle, demonstrating outstanding high-rate tolerance. This work provides new insights into the preparation of three-dimensional porous sulfur cathodes with high specific surface area and excellent catalytic activity using catalysts loaded on MXene substrates in RT Na-S batteries.
Keywords
INTRODUCTION
Lithium-ion batteries nowadays have been widely used in various fields due to the merit of high energy densities. However, lithium resources are relatively scarce in the earth’s crust, showing ever-increasing cost, making it difficult to meet the long-term market requirements[1,2]. Room-temperature sodium-sulfur
The most popular strategy to address the problems is encapsulating sulfur into host materials, forming a composite cathode. For example, nanostructured carbon materials, including carbon nanotubes, carbon nanofibers, hollow carbon nanospheres, etc., were exploited to serve as sulfur hosts to boost the electrical conductivity of the cathode and provide confinement for intermediate reaction products of polysulfides in RT Na-S batteries[6,7]. However, carbon materials only have weak interactions with polysulfides due to their non-polar nature, which contributes to limited suppression of polysulfide shuttling[8]. Therefore, the sulfiphilic transition metal oxides[9], nitride[10], sulfides[11-13] and alloys[14] nanomaterials were introduced into the nanosized porous carbon to further enhance the electrochemical properties of RT Na-S batteries. This is because the newly incorporated components not only exhibit superior chemical adsorption ability to polysulfides but also catalyze the conversion of polysulfides. Therefore, qualified sulfur host candidates for RT Na-S batteries should be equipped with the features below: (a) high specific surface area for loading a desired sulfur amount and relieving the volume change during the charge/discharge process, (b) strong adsorption ability of reaction intermediates to suppress their shuttling, and (c) high electrical conductivity and good catalytic performance for accelerated conversion kinetics at the cathode.
MXenes are a new kind of two-dimensional transition metal carbides/nitrides with the general composition Mn+1XnTx, where M represents transition metal elements (Ti, V, Mo Cr, V, etc.), X is carbon and/or nitrogen, and T refers to surface functional groups (-O, -F and -OH). They are equipped with polar functional groups, metallic conductivity and catalytic properties for polysulfides, which makes them potential sulfur hosts for RT Na-S batteries[15]. Multi/few-layer Ti3C2Tx MXene and MXene-based composites with heterostructure have been studied as sulfur hosts in RT Na-S batteries and displayed excellent electrochemical performance[16,17]. Nevertheless, restacking and agglomeration of MXene sheets impede the availability of surface-active sites for anchoring and catalyzing sodium polysulfides (NaPSs)[18-20].
Herein, we prepared three-dimensional porous C/CoFe-MXene composites via a combination of electrostatic self-assembly, co-precipitation and pyrolysis. The introduced CoFe alloy not only inhibits MXene restacking but also serves as new active sites to boost the chemical adsorption and catalytic conversion of polysulfides. By virtue of the synergistic effect of the components, the RT Na-S batteries with the C/CoFe-MXene cathode under 2.0 mg cm-2 of sulfur loading display a great specific capacity of
EXPERIMENTAL
Material synthesis
Synthesis of few-layer MXene dispersions and powder
First, 1.6 g lithium fluoride was added to hydrochloric acid solution (20 mL, 9 M) on a Teflon bottle. After stirring thoroughly, Ti3AlC2 powder (1 g) was added to the above mixture solution slowly and further stirred at 40 °C for 32 h. The resultant product was washed repetitively via 3,500 rpm centrifugation procedures until the pH of the supernatant reached 6. We then added an appropriate amount of deionized (DI) water, transferred the obtained clay-like deposits to a gas washing bottle and treated it by low-temperature ultrasonication for 1 h under argon atmosphere to delaminate MXene. Finally, a few-layer MXene dispersion was obtained by centrifugation (3,500 rpm, 30 min). In our experiment, around 16 mg mL-1 MXene solution was eventually obtained. A portion of the dispersion was freeze-dried to obtain the
Synthesis of C/CoFe-MXene and C/CoFe
Typically, 0.3 mmol of Co(NO3)2·6H2O and 0.45 mmol of Na3C6H5O7·2H2O were dissolved into 20 mL of DI water. Subsequently, the few-layer MXene dispersion (80 mg, 20 mL) was added to the above mixture solution and stirred for 10 min to obtain solution A. Additionally, 0.2 mmol of K3Fe(CN)6 was added into
Synthesis of S composites
The sulfur composites were prepared through a typical melt diffusion strategy. The as-prepared samples were first mixed with a mass ratio of 7:3 (S:C/CoFe-MXene) by hand milling. The resultant mixture was heated at 155 °C for 12 h in a tube furnace and further heated to 200 °C for 20 min in argon atmosphere to obtain the C/CoFe-MXene/S composite. The C/CoFe/S and MXene/S were prepared under the same conditions.
Material characterization
Structure investigation
X-ray diffraction (XRD) measurements were performed on a diffractometer (Rigaku SmartLab) with Cu Kα source (λ = 0.15406 nm). X-ray photoelectron spectroscopy (XPS, Thermo Fisher ESCALAB 250Xi) was operated to obtain the chemical state of the samples. Field emission scanning electron microscopy (SEM, JEOL Model JSM-6490) and transmission electron microscopy (TEM, JEOL JEM-2100F) with energy-dispersive X-ray spectroscopy (EDS) analysis were used to study the morphology, structure and element distribution. Raman spectra were collected by a WITEC confocal Raman system (Alpha300 R). A Micromeritics ASAP2020 analyzer was used to investigate the surface area and pore structure. Thermogravimetric analysis (TGA) was carried out using a thermogravimetric analyzer [TGA/differential scanning calorimetry (DSC)
Electrochemical measurements
S composites, super P, and sodium carboxymethylcellulose (mass ratio = 7:2:1 in DI water) were mixed into a uniform slurry and then coated onto an Al foil. The electrodes were cut into disks with a diameter of
Na2S6 solution adsorption experiment
The Na2S6 solution was prepared by sulfur and anhydrous Na2S powder with the molar ratio of 5:1 in a mixed solvent of EC: PC (v/v 1:1). Then, the suspension was stirred vigorously for 24 h at 80 °C in the argon-filled glove box. Finally, a 0.2 M brown Na2S6 solution was obtained. The different samples with the same mass were added into the as-prepared low concentration Na2S6 solution. The colors of the solutions after standing overnight were recorded by a digital camera and were further measured by ultraviolet-visible (UV-Vis) absorption spectra.
Symmetrical cell measurements
The two identical electrodes with the active sample were used as working and counter electrodes to construct a CR2032 coin cell with a GF/D glass fiber as the separator, and as-prepared Na2S6 solution as electrolyte. The CV tests of symmetrical cells were performed at the scan rates from1 to 10 mV s-1 between
Linear sweep voltammetry measurement of Na2S oxidation
A uniform ink was obtained by ultrasonically mixing 5 mg electrocatalyst, 700 μL anhydrous ethanol, 300 μL DI water and 50 μL Nafion. Then, 10 μL ink was dropped onto the glass carbon with an area of 0.196 cm-2 and dried to form a working electrode. A platinum wire and a saturated Ag/AgCl electrode were used as the counter electrode and reference electrode, respectively. The electrolyte was obtained by dissolving
RESULTS AND DISCUSSION
Figure 1A schematically depicts the fabrication procedure of the C/CoFe-MXene. First, few-layer MXene was obtained by selective etching of layered Ti3AlC2 MAX [Supplementary Figure 1A] and a further delamination[21,22]. A drop of Ti3C2Tx aqueous solution was coated onto a copper film to observe the morphology of MXene sheets. The diameter of most of the 2D sheets is of a few micrometers
Figure 1. (A) Schematic illustration for the synthesis of C/CoFe-MXene. SEM images of (B) CoFePBA-MXene and (c) C/CoFe-MXene; Inset in (C) is the magnified image; (D) TEM image; (E) HRTEM image; (F) HAADF-STEM image and (G) corresponding EELS elemental mappings of C/CoFe-MXene. SEM: Scanning electron microscopy; TEM: transmission electron microscopy; HAADF-STEM: high-angle annular dark field scanning transmission electron microscopy; HRTEM: high resolution transmission electron microscopy; EELS: electron energy-loss spectroscopy.
Phase changes of the samples and interlayer spacing of MXene were characterized by XRD analysis. No
Figure 2. XRD patterns of (A) C/CoFe-MXene, C/CoFe and MXene; (B) TGA curves of C/CoFe-MXene/S, C/CoFe/S and MXene/S; High-resolution (C) C 1s and (D) O 1s XPS spectra. XRD: X-ray diffraction; TGA: thermogravimetric analysis; XPS: X-ray photoelectron spectroscopy.
XPS measurement was conducted to further characterize the chemical states and bonds of MXene, C/CoFe and C/CoFe-MXene. In Figure 2C, the C 1s XPS spectrum of C/CoFe-MXene shows some typical predominant peaks at 281.5 eV, 284.8 eV, 285.4 eV, 286.5 eV and 288.6 eV, which originate from C-Ti-Tx, C-C, C-N, C-O and C=O, respectively[25]. The O 1s XPS spectrum [Figure 2D] shows five predominant peaks at 529.8 eV, 530.7 eV, 531.4 eV, 532.1 eV and 533.0 eV, which correspond to Ti-O, C-Ti-O, Ti-O-M (M=Co, Fe), C-Ti-OH and H2O, respectively[26]. Compared with pristine MXene, the emergence of Ti-O-M (M=Co, Fe) peak in C/CoFe-MXene means that the Co and Fe in the CoFe alloy are bonded to the oxygen terminals on the surface of the MXene to form a heterostructure[27,28]. To further verify the coupling effect between CoFe alloy and MXene, we performed high-resolution XPS tests [Supplementary Figure 8]. In the Co 2p XPS spectra of C/CoFe-MXene, the two peaks at 778.4 eV and 795.3 eV can be ascribed to
The adsorption effect of C/CoFe-MXene toward NaPSs can also be evaluated by NaPS adsorption experiment and related UV-vis absorption spectra. As shown in Figure 3A, the color of NaPS solution adsorbed by C/CoFe-MXene became transparent, while brown color was retained in the other two samples, which demonstrates the strongest NaPS adsorption capability of C/CoFe-MXene. The result of the UV-vis spectrum also shows that the peak intensity of NaPSs is lower than that of other two samples and the blank, double confirming the best NaPS adsorption capability in C/CoFe-MXene. The high-resolution XPS spectrum is applied to reveal the interaction of C/CoFe-MXene with the polysulfide. As shown in Figure 3B and C, and Supplementary Figure 9, after adsorption of Na2S6, the Co2+/Co3+ and Fe2+/Fe3+ peaks shift slightly to lower binding energies, which means that the Na2S6 species transfer electrons to Co and Fe atoms. This can also be considered to the formation of Co-S/Fe-S bonds due to the Lewis acid-based role[37]. In Ti 2p XPS spectrum of C/CoFe-MXene [Supplementary Figure 10], the increased intensity of the Ti2+ and Ti3+ may be related to the strong interaction of Ti atoms with polysulfides[38]. Furthermore, in the S 2p XPS spectrum [Figure 3D], the terminal sulfur (ST-1) and bridging sulfur (SB0) atoms were located at
Figure 3. (A) UV-vis spectra after adsorption between NaPSs with different samples and digital photos (inset); High-resolution (B) Co 2p; (C) Fe 2p XPS spectra of C/CoFe-MXene before and after adsorption; High-resolution (D) S 2p XPS spectra of C/CoFe-MXene, C/CoFe and MXene after adsorption; (E) CV curves of symmetric batteries with different samples at 10 mV s-1; (F) LSV curves of Na2S oxidation and corresponding Tafel plots (inset). UV-vis: Ultraviolet-visible; XPS: X-ray photoelectron spectroscopy; CV: cyclic voltammetry; LSV: linear sweep voltammetry; NaPSs: sodium polysulfides.
CV measurements were employed to examine the electrochemical behaviors of the cell with
Figure 4. (A) The first five CV curves of the cell with C/CoFe-MXene electrode; (B) Rate capability of different electrodes and (C) galvanostatic charge/discharge profiles of the cell with C/CoFe-MXene electrode at different rates; (D) Cycling performance of the cell with different electrodes under 1 C; (E) Galvanostatic charge/discharge profiles of the cell at the 1st and the 700th cycle under 1 C; (F) Nyquist plots after cycling at 1 C; (G) Ultralong-term cycling performance of C/CoFe-MXene electrode at 5 C; (H) Cycling performance of C/CoFe-MXene/S with high sulfur loading at 0.5 C and inset digital photo of a light-emitting diode (LED) powered by the cell. CV: Cyclic voltammetry.
Na+ diffusion rate plays a crucial role for the redox kinetics of NaPSs, which has been investigated by CV measurements and GITT of RT Na-S batteries using C/CoFe-MXene, C/CoFe and MXene electrodes. As shown in Figure 5A-C, the CV curves with different scan rates from 0.1 mV s-1 to 1.0 mV s-1 indicate that the C/CoFe-MXene electrode exhibits less potential polarization compared to other electrodes with the increased scan rates, suggesting superior redox reversibility of C/CoFe-MXene. The relationship between the peak current (i) and scan rate (v) and its equivalent form are given by:
Figure 5. CV curves at different scan rates and corresponding linear fits of the peak current densities (inset) of the cell with (A)
Where b is a constant. A b value of 0.5 suggests that the electrochemical process is limited by electrolyte ion diffusion, while b=1 represents a non-diffusion controlled capacitive process where surface capacitance is dominant in the charge storage mechanism[44]. According to the fitted plots in Figure 5A inset, the calculated b values are 0.764 and 0.623 for the cathodic peak/ anodic peak, confirming a hybrid surface-controlled and diffusion-controlled charge storage feature of C/CoFe-MXene electrode. On the other hand, GITT curves of C/CoFe-MXene, C/CoFe and MXene reveal superior performance of heterostructures constructed by CoFe alloy and MXene on the charge transfer properties during the sodiation and desodiation processes [Figure 5D]. The sodium-ion diffusion coefficient DNa+ (cm2 s-1) was determined through GITT results. The value of DNa+ was calculated using the Fick’s second law:
where MB represents the molar mass of active material, S means the electrode area, VM equals the molar volume of the active materials, mB describes the mass of active material, and τ denotes the current time[45]. To be more specific, the C/CoFe-MXene has higher Na+ diffusion coefficient than the C/CoFe and MXene during the whole voltage range [Figure 5E], suggesting the heterostructure of C/CoFe-MXene is more beneficial to Na+ diffusion. This also shows that low resistance and fast reaction kinetics are maintained throughout the charge-discharge process, which is also demonstrated by the in-situ EIS [Figure 5F and Supplementary Figure 16]. Specifically, after discharge to 1.0 V at the first discharge stage, there is a slight increase in resistance because the dissolved polysulfide in the electrolyte increases the concentration of the electrolysis[46]. When further discharged to 0.5 V, the resistance increases further, because the polysulfide is converted into solid sodium sulfide and deposited on the cathode surface. That is why there are two semicircles in the middle and low frequency ranges and the internal resistance of the battery reaches the maximum in this state. During the charging process, the resistance decreases first and then increases as sodium sulfide converts to polysulfide and then to solid sulfur. Similarly, the change in resistance during the second cycle of discharge is almost the same as during the first cycle of discharge, which further proves the reversible redox reaction of RT Na-S batteries in this system[47]. Additionally, compared to other samples,
Density functional theory (DFT) calculations were carried out to elucidate the strong interaction between CoFe alloy and MXene, investigate the adsorption behavior of NaPSs on different substrates and reveal their electrocatalytic functionalities during sulfur conversion. Supplementary Figure 17 displayed the simulated clean surface of MXene, CoFe and CoFe-MXene. The reduced conversion process of NaPSs is divided into six steps[48,49], and their corresponding structures in the side view are shown in Figure 6A and Supplementary Figure 18. By comparing with the binding energy of NaPSs on different substrates [Figure 6B and Supplementary Table 2], the CoFe-MXene is found to possess superior anchoring ability for polysulfides. From the calculated differential charge density [Figure 6C], the electrons are transferred from CoFe alloy to MXene in heterointerface, suggesting a self-built internal electric field that could accelerate the electron/ion transport during the charge/discharge process[50,51]. These results are consistent with the analysis of the XPS. The density of states (DOS) of the CoFe-MXene, CoFe and MXene [Figure 6D] reveals that CoFe-MXene composite possesses increased DOS at Fermi level compared with CoFe and MXene, confirming its higher metallic conductivity. This also indicated the strong coupling effect between CoFe and MXene. The optimized adsorption configurations in the top view, showing the interactions between NaPS species and CoFe-MXene, CoFe, and MXene, are displayed in Figure 6E and Supplementary Figure 19. Furthermore, Gibbs free energies (ΔG) are estimated for the conversion of NaPS species on three substrates. As shown in Figure 6E, the transformation from S8 to Na2S8 is spontaneous, while every conversion step from Na2S8 to Na2S faces an energy uphill. In particular, the conversion from Na2S4 to Na2S2 exhibits an extraordinarily high-energy barrier, which can be regarded as the rate-limiting step during the discharge process[52]. The largest ΔG values for CoFe-MXene, CoFe and MXene surfaces are 0.89 eV, 1.10 eV and 1.38 eV, respectively. Obviously, the CoFe-MXene exhibits the lowest ΔG barrier, which proves that the reduction process of sulfur could be boosted on CoFe-MXene surface from the perspective of thermodynamics. These results demonstrate that heterostructure from the CoFe-MXene nanocomposite exerts a synergistic catalytic effect that promotes the whole conversion and shows better reaction kinetics.
Figure 6. (A) Optimized adsorption conformations of Na2S6; (B) Binding energy of NaPSs species; (C) Differential charge density distribution at interface of CoFe-MXene, yellow and blue regions indicate electron accumulation and depletion, respectively. (D) Density of states; (E) Gibbs free energy profiles of NaPSs species on the CoFe-MXene, CoFe and MXene. NaPSs: Sodium polysulfides.
CONCLUSIONS
In summary, C/CoFe alloys embedded on MXene substrate catalysts were designed via an in situ growth strategy as a sulfur host for RT Na-S batteries. Specifically, compared to the performance of C/CoFe and MXene, the C/CoFe-MXene delivered an impressive specific capacity and an ultralow capacity decay of 0.0089% per cycle at 5 C, demonstrating high tolerance at high current densities. Through comprehensive systemic characterizations on structural features, chemical interactions, and electrochemical behavior, the structure-property relationship in the dynamic electrode reaction process was revealed. Structurally, the introduction of C/CoFe alloys facilitates the assembly of three-dimensional structures with MXene, allowing MXene to expose more active surface-supported nanoalloys to form heterostructures. The C/CoFe alloy and MXene are connected by bonding, enhancing the stability of the catalyst under long-term cycles and high current density, preventing detachment and failure. Functionally, C/CoFe alloys act as potent catalysts for the adsorption and conversion of polysulfides. The heterogeneous interface between the C/CoFe alloy and MXene facilitates rapid ion transport, accelerates reaction kinetics, and improves rate performance. This work provides new insights into understanding the catalytic role of MXene-based supported nanoalloy catalysts during kinetic conversion.
DECLARATIONS
Authors’ contributions
Methodology, characterization, data analysis, writing-original draft: Yu, X.
Characterization: Liang, K.; Liu, S.; Li, L.; Zhu, Y.
Writing-review and editing: Li, H.
Conceptualization, supervision, writing-review and editing, funding resources: Huang, H.
All authors have given approval to the final version of the manuscript.
Availability of data and materials
All data are available in the manuscript and the Supplementary Materials.
Financial support and sponsorship
This work was supported by the Science and Technology Program of Guangdong Province of China (2021B1515130010). Yu, X. thanks the financial support from the Research Institute for Smart Energy, the Hong Kong Polytechnic University.
Conflicts of interest
All authors declared that there are no conflicts of interest.
Ethical approval and consent to participate
Not applicable.
Consent for publication
Not applicable.
Copyright
© The Author(s) 2025.
Supplementary Materials
REFERENCES
1. Sun, Y.; Liu, N.; Cui, Y. Promises and challenges of nanomaterials for lithium-based rechargeable batteries. Nat. Energy. 2016, 1, 1671.
2. Pan, H.; Hu, Y.; Chen, L. Room-temperature stationary sodium-ion batteries for large-scale electric energy storage. Energy. Environ. Sci. 2013, 6, 2338-60.
3. Zhao, L.; Tao, Y.; Zhang, Y.; et al. A critical review on room-temperature sodium-sulfur batteries: from research advances to practical perspectives. Adv. Mater. 2024, 36, 2402337.
4. Jin, F.; Wang, B.; Wang, J.; et al. Boosting electrochemical kinetics of S cathodes for room temperature Na/S batteries. Matter 2021, 4, 1768-800.
5. Zhang, S.; Yao, Y.; Yu, Y. Frontiers for room-temperature sodium-sulfur batteries. ACS. Energy. Lett. 2021, 6, 529-36.
7. Yang, J.; Han, H.; Repich, H.; et al. Recent progress on the design of hollow carbon spheres to host sulfur in room-temperature sodium-sulfur batteries. New. Carbon. Mater. 2020, 35, 630-45.
8. Yang, X.; Luo, J.; Sun, X. Towards high-performance solid-state Li-S batteries: from fundamental understanding to engineering design. Chem. Soc. Rev. 2020, 49, 2140-95.
9. Xiao, Y.; Zheng, Y.; Yao, G.; et al. Defect engineering of a TiO2 anatase/rutile homojunction accelerating sulfur redox kinetics for high-performance Na-S batteries. Dalton. Trans. 2024, 53, 8168-76.
10. Ye, X.; Ruan, J.; Pang, Y.; et al. Enabling a stable room-temperature sodium-sulfur battery cathode by building heterostructures in multichannel carbon fibers. ACS. Nano. 2021, 15, 5639-48.
11. Asif, M.; Ali, Z.; Qiu, H.; Rashad, M.; Hou, Y. Confined polysulfide shuttle by nickel disulfide nanoparticles encapsulated in graphene nanoshells synthesized by cooking oil. ACS. Appl. Energy. Mater. 2020, 3, 3541-52.
12. Sadaqat, A.; Ali, G.; ul, H. M.; Liaqat, A.; Khalid, S.; Farooq, K. M. Binary Co3S4/SnS chalcogenide composites anchored on graphene oxide and carbon nanotubes as anodes for Na-ion batteries. Appl. Surf. Sci. 2024, 665, 160323.
13. Guo, Q.; Ma, Y.; Chen, T.; et al. Cobalt sulfide quantum dot embedded N/S-doped carbon nanosheets with superior reversibility and rate capability for sodium-ion batteries. ACS. Nano. 2017, 11, 12658-67.
14. Wang, L.; Wang, H.; Zhang, S.; et al. Manipulating the electronic structure of nickel via alloying with iron: toward high-kinetics sulfur cathode for Na-S batteries. ACS. Nano. 2021, 15, 15218-28.
15. Dai, X.; Wang, Z.; Wang, X.; et al. MXene-based sodium-sulfur batteries: synthesis, applications and perspectives. Rare. Met. 2025, 44, 1522-55.
16. Huo, X.; Liu, Y.; Li, R.; Li, J. Two-dimensional Ti3C2Tx@S as cathode for room temperature sodium-sulfur batteries. Ionics 2019, 25, 5373-82.
17. Reddy, B.; Cho, G.; Reddy, N.; et al. Layered-like structure of TiO2-Ti3C2 Mxene as an efficient sulfur host for room-temperature sodium-sulfur batteries. J. Alloys. Compd. 2021, 883, 160910.
18. Zhang, Y.; Ma, C.; He, W.; et al. MXene and MXene-based materials for lithium-sulfur batteries. Prog. Nat. Sci. Mater. Int. 2021, 31, 501-13.
19. Zhang, S.; Zhong, N.; Zhou, X.; et al. Comprehensive design of the high-sulfur-loading Li-S battery based on MXene nanosheets. Nano-Micro. Lett. 2020, 12, 112.
20. Zhao, W.; Lei, Y.; Zhu, Y.; et al. Hierarchically structured Ti3C2T MXene paper for Li-S batteries with high volumetric capacity. Nano. Energy. 2021, 86, 106120.
21. Li, H.; Zhou, H.; Zhuang, L.; Liu, T.; Han, W.; Huang, H. Enhanced ion diffusion in flexible Ti3C2TX MXene film for high-performance supercapacitors. Adv. Energy. Sustain. Res. 2022, 3, 2100216.
22. Yu, X.; Yang, Y.; Si, L.; Cai, J.; Lu, X.; Sun, Z. V4C3TX MXene: first-principles computational and separator modification study on immobilization and catalytic conversion of polysulfide in Li-S batteries. J. Colloid. Interface. Sci. 2022, 627, 992-1002.
23. Ghidiu, M.; Barsoum, M. W. The {110} reflection in X-ray diffraction of MX ene films: misinterpretation and measurement via non-standard orientation. J. Am. Ceram. Soc. 2017, 100, 5395-9.
24. Liu, W.; Zheng, Y.; Zhang, Z.; et al. Ultrahigh gravimetric and volumetric capacitance in Ti3C2Tx MXene negative electrode enabled by surface modification and in-situ intercalation. J. Power. Sources. 2022, 521, 230965.
25. Feng, J.; Liu, W.; Shi, C.; et al. Enabling fast diffusion/conversion kinetics by thiourea-induced wrinkled N, S co-doped functional MXene for lithium-sulfur battery. Energy. Storage. Mater. 2024, 67, 103328.
26. Wang, Z.; Jiang, H.; Wei, C.; et al. Ultrasmall CoFe bimetallic alloy anchored on fluoride-free MXene by one-pot etching strategy for the barrier-adsorption-catalyst functions of polysulfides in lithium-sulfur batteries. Adv. Funct. Mater. 2024, 34, 2315178.
27. Hui, X.; Zhao, R.; Zhang, P.; Li, C.; Wang, C.; Yin, L. Low-temperature reduction strategy synthesized Si/Ti3C2 MXene composite anodes for high-performance Li-ion batteries. Adv. Energy. Mater. 2019, 9, 1901065.
28. Huang, P.; Zhang, S.; Ying, H.; et al. Fabrication of Fe nanocomplex pillared few-layered Ti3C2Tx MXene with enhanced rate performance for lithium-ion batteries. Nano. Res. 2021, 14, 1218-27.
29. Mou, J.; Li, Y.; Liu, T.; et al. Metal-organic frameworks-derived nitrogen-doped porous carbon nanocubes with embedded Co nanoparticles as efficient sulfur immobilizers for room temperature sodium-sulfur batteries. Small. Methods. 2021, 5, 2100455.
30. Qi, W.; Wu, W.; Cao, B.; Zhang, Y.; Wu, Y. Fabrication of CoFe/N-doped mesoporous carbon hybrids from Prussian blue analogous as high performance cathodes for lithium-sulfur batteries. Int. J. Hydrogen. Energy. 2019, 44, 20257-66.
31. Wang, Y.; Zhu, L.; Wang, J.; Zhang, Z.; Yu, J.; Yang, Z. Enhanced chemisorption and catalytic conversion of polysulfides via CoFe@NC nanocubes modified separator for superior Li-S batteries. Chem. Eng. J. 2022, 433, 133792.
32. Lu, C.; Li, A.; Zhai, T.; et al. Interface design based on Ti3C2 MXene atomic layers of advanced battery-type material for supercapacitors. Energy. Storage. Mater. 2020, 26, 472-82.
33. Zhang, G.; Yang, H.; Zhou, H.; et al. MXene-mediated interfacial growth of 2D-2D heterostructured nanomaterials as cathodes for zn-based aqueous batteries. Angew. Chem. Int. Ed. 2024, 63, e202401903.
34. Yu, M.; Zhou, S.; Wang, Z.; Zhao, J.; Qiu, J. Boosting electrocatalytic oxygen evolution by synergistically coupling layered double hydroxide with MXene. Nano. Energy. 2018, 44, 181-90.
35. Li, H.; Fan, K.; Xiong, P.; et al. Selective grafting of phosphorus onto Ti3C2Tx MXene enables a two-proton process and enhanced charge storage. J. Mater. Chem. A. 2024, 12, 3449-59.
36. He, Y.; Zhao, Y.; Zhang, Y.; et al. Building flexibly porous conductive skeleton inlaid with surface oxygen-dominated MXene as an amphiphilic nanoreactor for stable Li-S pouch batteries. Energy. Storage. Mater. 2022, 47, 434-44.
37. Zhang, G.; Chen, X.; Yu, X.; et al. Crystalline-amorphous heterostructure on the phosphatized P-CoS2/CNT for augmenting the catalytic conversion kinetics of Li-S batteries. Chem. Eng. J. 2024, 488, 150696.
38. Yao, Y.; Wang, S.; Jia, X.; et al. Freestanding sandwich-like hierarchically TiS2-TiO2/Mxene bi-functional interlayer for stable Li-S batteries. Carbon 2022, 188, 533-42.
39. Park, S. J.; Choi, Y. J.; Kim, H.; et al. Stable immobilization of lithium polysulfides using three-dimensional ordered mesoporous Mn2O3 as the host material in lithium-sulfur batteries. Carbon. Energy. 2024, 6, e487.
40. Ou, L.; Mou, J.; Peng, J.; Zhang, Y.; Chen, Y.; Huang, J. Heterostructured Co/CeO2-decorating N-doped porous carbon nanocubes as efficient sulfur hosts with enhanced rate capability and cycling durability toward room-temperature Na-S batteries. ACS. Appl. Mater. Interfaces. 2024, 16, 3302-10.
41. Mou, J.; Li, Y.; Ou, L.; Huang, J. A highly-efficient electrocatalyst for room temperature sodium-sulfur batteries: assembled nitrogen-doped hollow porous carbon spheres decorated with ultrafine α-MoC1-x nanoparticles. Energy. Storage. Mater. 2022, 52, 111-9.
42. Liu, H.; Lai, W. H.; Yang, Q.; et al. Understanding Sulfur redox mechanisms in different electrolytes for room-temperature Na-S batteries. Nano-Micro. Lett. 2021, 13, 121.
43. Tang, K.; Peng, X.; Zhang, Z.; et al. A highly dispersed cobalt electrocatalyst with electron-deficient centers induced by boron toward enhanced adsorption and electrocatalysis for room-temperature sodium-sulfur batteries. Small 2024, 20, e2311151.
44. Zhou, X.; Yu, Z.; Yao, Y.; et al. A high-efficiency Mo2C electrocatalyst promoting the polysulfide redox kinetics for Na-S batteries. Adv. Mater. 2022, 34, 2200479.
45. Jiang, Y.; Yu, Z.; Zhou, X.; et al. Single-atom vanadium catalyst boosting reaction kinetics of polysulfides in Na-S batteries. Adv. Mater. 2023, 35, 2208873.
46. Zhao, Z.; Yi, Z.; Li, H.; et al. Synergetic effect of spatially separated dual co-catalyst for accelerating multiple conversion reaction in advanced lithium sulfur batteries. Nano. Energy. 2021, 81, 105621.
47. Wang, Y.; Wang, Y.; Xu, C.; et al. Phosphor-doped carbon network electrocatalyst enables accelerated redox kinetics of polysulfides for sodium-sulfur batteries. ACS. Nano. 2024, 18, 3839-49.
48. Jayan, R.; Islam, M. M. Mechanistic insights into interactions of polysulfides at VS2 interfaces in Na-S batteries: a DFT study. ACS. Appl. Mater. Interfaces. 2021, 13, 35848-55.
49. Fang, D.; Ghosh, T.; Huang, S.; et al. Core-shell tandem catalysis coupled with interface engineering for high-performance room-temperature Na-S batteries. Small 2023, 19, 2302461.
50. Yu, X.; Wang, N.; Sun, Z.; Shao, L.; Shi, X.; Cai, J. Separator modified by a caterpillar-like composite with interconnected N-doped carbon nanotubes decorated Co-MnO heterointerface enabling robust polysulfide adsorption and catalytic conversion for Li-S batteries. Electrochim. Acta. 2023, 457, 142497.
51. Li, T.; Liang, L.; Chen, Z.; Zhu, J.; Shen, P. Hollow Ti3C2T MXene@CoSe2/N-doped carbon heterostructured composites for multiphase electrocatalysis process in lithium-sulfur batteries. Chem. Eng. J. 2023, 474, 145970.
Cite This Article

How to Cite
Download Citation
Export Citation File:
Type of Import
Tips on Downloading Citation
Citation Manager File Format
Type of Import
Direct Import: When the Direct Import option is selected (the default state), a dialogue box will give you the option to Save or Open the downloaded citation data. Choosing Open will either launch your citation manager or give you a choice of applications with which to use the metadata. The Save option saves the file locally for later use.
Indirect Import: When the Indirect Import option is selected, the metadata is displayed and may be copied and pasted as needed.
About This Article
Copyright
Data & Comments
Data
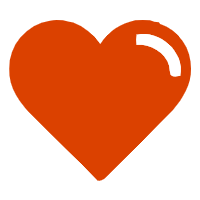
Comments
Comments must be written in English. Spam, offensive content, impersonation, and private information will not be permitted. If any comment is reported and identified as inappropriate content by OAE staff, the comment will be removed without notice. If you have any queries or need any help, please contact us at [email protected].