Tailoring crystal planes and oxygen vacancies of ceria for enhanced catalytic performance of single-atom Ru in hydrogenative dearomatization of lignin-derived phenols
Abstract
Lignin-based guaiacol and its derivatives can be hydrogenated to synthesize 2-methoxycyclohexanols (2-MCHs), widely used in the pharmaceutical industry, while the efficient catalytic conversion of guaiacols into 2-MCHs is challenging due to the interference of the side reaction of CAr-OCH3 bond cleavage. In this work, highly selective hydrogenation of various guaiacyl lignin-derived phenols to 2-MCHs (yields of 86%-97%) was realized over a single-atom Ru-based catalyst (Ru1/o-CeO2-ov) that preferentially exposes the CeO2(111) plane and has abundant oxygen vacancies. Control experiments and mechanism studies expounded that Ru-O-Ce is the active site for hydrogenation of aromatic ring in guaiacols, and the exposed (111) plane and abundant oxygen vacancies of ceria can reduce the activation energy of aromatic ring hydrogenation, thereby enabling guaiacols to generate the corresponding hydrogenated products with high selectivity. Response surface optimization experiments indicated that temperature and time have relatively more significant effects on the 2-MCH yield. Moreover, the
Keywords
INTRODUCTION
Catalytic upgrading of lignin-based renewable resources into high-value-added chemicals and biofuels will bring enormous social and economic benefits to humankind, which has also been the focus of researchers in recent years[1]. As an essential class of lignin phenols, guaiacol and its derivatives can be converted into catechols[2], phenols[3], cyclohexanols[4,5], ethers[6,7], arenes[8,9], cyclohexanes[10], etc., through selective cleavage of C-O bond and/or hydrogenation of benzene ring[11]. Notably, the aromatic ring hydrogenation of guaiacols can produce 2-methoxycyclohexanols (2-MCHs), which have both hydroxyl (-OH) and ether
Scheme 1. Synthesis methods of 2-MCHs. Classical method (A) and sustainable method in previous work (B) and this work (C).
Typically, the CAr-OCH3 cleavage of guaiacols is directly competitive with the aromatic ring hydrogenation reaction[19,20], which depends on the identity of the active hydrogen (H* and H+), the isomerization equilibrium of ketene and alcohol, and the transition state barrier of the CAr-OCH3 cleavage[21]. In previous studies, the intramolecular hydrogen bonds formed by guaiacol were illustrated to preferentially undergo CAr-OCH3 cleavage over Co-based catalysts[22]. The ring hydrogenation in guaiacol was found to be faster than CAr-OCH3 cleavage from the perspective of transition state energy, but the latter was more likely to occur from a microdynamic analysis, possibly due to the considerable interaction between the benzene ring on guaiacol and the catalyst[23]. In addition, 2-MCHs were inclined to complete the demethoxylation reaction under certain conditions, resulting in the final production of cyclohexanols rather than
Over the past decade, single-atom catalysts (SACs) have shown high catalytic performance in many reactions due to the isolated atoms being more easily affected by metal oxides to change their d-band center position and d-band width[31-33]. However, due to the lack of metal bonds and weak hydrogen spillover performance, it is difficult for SACs to complete the hydrogenation of benzene rings with comparable efficiency to traditionally supported metal NPs[24,34]. SACs have been developed for the upgrading of vanillin, among which Co-based SAC [Co1@NC-(SBA)] can promote the hydrodeoxygenation of vanillin to
Here, a Ru-based SAC (Ru1/o-CeO2-ov) with exposed crystal planes and oxygen vacancies of ceria was precisely constructed, which could completely hydrogenate the aromatic ring while suppressing the
EXPERIMENTAL
Materials
Cerous nitrate hexahydrate (99%), sodium hydroxide (99%), sodium orthophosphate (98%), ruthenium trichloride hydrate (99.95% metals basis), cobalt nitrate hexahydrate (99.99% metals basis), copper(ii) nitrate hydrate (99.99% metals basis), ferric nitrate nonahydrate (99.99% metals basis), nickel(II) chloride hexahydrate (99.9%), phenol (99.5%), guaiacol (98%), 4-methylphenol (99%), 4-methylguaiacol (99%),
Catalyst preparation
Preparation of SACs
The preparation methods of ceria support with different morphologies (x-CeO2) are shown in the Supporting information. A series of SACs were prepared by loading metal ions onto x-CeO2 using the supersaturated impregnation method. In a typical example, 100 mg octahedral ceria (o-CeO2) was dispersed in 20 mL deionized water, and then an aqueous solution containing 2.0 mg hydrated ruthenium trichloride was added dropwise to the dispersion. The resulting mixed turbid liquid was stirred at 25 °C for 6 h, and the Ru1/o-CeO2 catalyst was obtained after washing, centrifugation, and vacuum drying (80 °C). Next,
Preparation of Ru1/x-CeO2-ov
Oxygen vacancies were introduced into Ru-based SACs by a high-temperature reduction method. The typical process for preparing Ru1/x-CeO2-ov was to place 100 mg of Ru1/x-CeO2 in a tube furnace. In a 10% H2/Ar atmosphere, the temperature was increased from 30 °C to 400 °C (rate of 5 °C min-1) and kept constant for 3 h. Then, the temperature was reduced to 100 °C (rate of 5 °C min-1), and the program was stopped. The sample was taken out when it was cooled down to below 30 °C for use.
Catalyst characterization
The X-ray diffraction (XRD) patterns and Raman spectra of the samples were obtained using Bruker’s D8 ADVANCE X-ray powder diffractometer and HORIBA’s LabRam HR Evolution, respectively. The elements of the samples were quantitatively analyzed using a PE Optima 8000 inductively coupled plasma optical emission spectrometer (ICP-OES) from PerkinElmer, USA. X-ray photoelectron spectroscopy (XPS) of all samples was measured by ESCALAB 250XI instrument from Thermo. The microstructure, elemental composition, and distribution of the samples were analyzed using a scanning transmission electron microscope (STEM) combined with an energy-dispersive X-ray spectrometer (EDS). Atomic mapping of the sample was collected using an aberration-corrected high-angle annular dark field STEM (AC HAADF STEM, JEOL ARM 200F) equipped with a Cs corrector (JEM-2200FS) and a high-resolution spectrometer with a large solid angle of 0.97 sr (JED-2300T). The acceleration voltage was 200 kV, the STEM probe current was 17 pA, and the probe size was 0.133 nm. Surface area and pore size distribution were determined using a Micromeritics ASAP2460 apparatus. The hydrogen temperature-programmed reduction (H2-TPR) curves were obtained on an AutoChem II 2920 instrument. The characteristics of oxygen vacancies in the samples were analyzed using a Bruker ELEXSYS E500 electron paramagnetic resonance (EPR) spectrometer.
Catalyst activity evaluation and first-principles calculations
Model molecule experiments
The hydrogenation reactions of lignin-derived phenolic model molecules were carried out in a high-pressure reactor (25 mL) equipped with a magnetic stirrer. In a typical example, 1 mmol of guaiacol, 3 mL of n-octane (solvent), 0.3 mol% Ru1/o-CeO2-ov catalyst, and 20 mg of n-hexadecane as an internal standard were added to the autoclave. The products were qualitatively analyzed using an Agilent 5,977 A gas chromatograph-mass spectrometer (GC-MS), and quantitatively analyzed using an Agilent 7,890 A gas chromatograph (GC) equipped with a hydrogen flame ionization detector and an HP-5 capillary column (30 m × 0.25 mm × 0.25 µm). The catalyst activity evaluation indexes include guaiacol conversion (Conv.), 2-MCH selectivity (Sel.), and 2-MCH yield, which were calculated according to
Where n1 is the molar amount of the initial substrate, n2 is the molar amount of the remaining substrate, and n3 is the molar amount of 2-MCHs generated. In addition, to compare the activity of catalysts with those in the literature, the turnover frequency (TOF) of this work was calculated according to
Real lignin oil experiment
The hydrogenation of real lignin oil was also carried out in a high-pressure reactor equipped with a magnetic stirrer. The specific operation steps were to add 150 mg of lignin oil, 3 mL of n-octane, and
Theoretical calculations
Density functional theory (DFT) calculations were performed in this work using the Vienna ab initio simulation package (VASP) software. The calculation details are given in the Supplementary Materials.
RESULTS AND DISCUSSION
Catalyst preparation and characterization
The preparation process of octahedral (o-CeO2), cubic (c-CeO2), and rod-shaped (r-CeO2) ceria is shown in Figure 1A. XRD patterns confirmed that the characteristic diffraction peaks of the three carriers all belong to CeO2 crystals with face-centered cubic structure (PDF# 43-1002) [Supplementary Figure 1]. Transmission electron microscopy (TEM) images showed that the supports o-CeO2, c-CeO2, and r-CeO2 corresponded to octahedral, cubic, and rod-shaped crystal morphologies, respectively [Supplementary Figures 2-4]. Each face on o-CeO2 and c-CeO2 had the same crystal face cluster, corresponding to {111} and {100}, respectively, which indicated that o-CeO2 and c-CeO2 were prone to expose the (111) and (100) planes[39]. The supersaturated impregnation and hydrogen reduction method was adopted to prepare a series of Ru-based SACs (Ru1/x-CeO2-ov) based on three CeO2 supports with different morphologies [Figure 1A]. The catalysts introduced with single-atom Ru still maintained the crystalline structure of ceria [Supplementary Figure 5]. The EDS images displayed that the metal Ru on the catalysts Ru1/x-CeO2-ov was evenly dispersed on the carrier [Figure 1B-D]. ICP-OES results showed that the mass fraction of Ru in the above four catalysts was between 0.287%-0.541%, and the lower loading amount of Ru could be better dispersed on the surface of the ceria [Table 1]. P and Cl element signals were not detected by ICP, indicating that these elements could be removed by water washing and hydrogen pre-reduction. Similarly, the characteristic peaks of P and Cl were not observed in the EDS spectra of Ru1/x-CeO2-ov [Supplementary Figures 6-8]. In addition, since the EDS energy spectrum could only observe the element content near the surface of the catalyst, the Ru content tested by EDS was much higher than the result of ICP-OES [Table 1], which confirmed that Ru was more loaded on the surface of the ceria support. High-resolution TEM images could further observe that the catalysts prepared with three morphologies of x-CeO2 carriers all had visible lattice patterns [Figure 1E-G]. Fast Fourier transform (FFT) of the lattice patterns further confirmed that Ru1/o-CeO2-ov and
Figure 1. (A) Schematic diagram of the preparation process of Ru-based catalysts supported on ceria of different morphologies; (B-D) HAADF STEM images and EDS elemental mappings of Ru1/o-CeO2-ov, Ru1/c-CeO2-ov, and Ru1/r-CeO2-ov; (E-G) High-resolution TEM images of Ru1/x-CeO2-ov (inset is FFT images); (H) AC HAADF STEM EDS mapping of the Ru1/o-CeO2-ov catalyst. AC HAADF STEM: Aberration-corrected high-angle annular dark field scanning transmission electron microscope; EDS: dispersive X-ray spectrometer; TEM: transmission electron microscopy.
Catalyst characterization results
Catalyst | Ru content (wt%) | SBET (m2 g-1)a | FWHM (°) | Hydrogen consumption (μmol g-1)b | ||
ICP-OES | EDS | α | β | |||
Ru1/o-CeO2-ov | 0.287 | 1.26 | 7.22 | 0.21 | 63.7 | 75.6 |
Ru1/c-CeO2-ov | 0.452 | 1.35 | 5.35 | 0.15 | 52.6 | 103.6 |
Ru1/r-CeO2-ov | 0.541 | 1.88 | 61.34 | 0.61 | 86.4 | 246.1 |
The oxygen vacancies in ceria can regulate the electronic structure of active metals, change the charge transfer ability of the material, and thus improve the catalyst performance[42]. H2-TPR results showed that the Ru1/x-CeO2-ov sample had two obvious reduction peaks, in which the first peak (α, ca. 100 °C) can be attributed to the reduction of Ru on the Ru-O-Ce site, while the second peak (β, 150-300 °C) may come from the reduction caused by the embedding of Ru atoms into the CeO2 lattice [Figure 2A]. Notably, the H2 consumption of the α and β peaks was much greater than that required for the reduction of Ru-O-Ce due to the lower Ru loading [Table 1]. A generally accepted explanation is that introducing Ru causes strong metal-support interactions, which leads to the transfer of electrons from Ru to ceria, thereby promoting the continuous reverse spillover of oxygen in ceria to Ru[43]. In a hydrogen atmosphere, oxygen atoms diffuse from the CeO2 bulk to the surface, and the effect of Ru metal accelerates the oxygen spillover process. The water generated by the combination of oxygen and hydrogen will escape from the catalyst surface after exceeding 300 °C, resulting in the appearance of oxygen vacancies[44]. The higher oxygen mobility caused by the Ru-O-Ce bond could dynamically form more oxygen vacancies and efficiently complete the catalytic reaction.
Figure 2. (A) H2-TPR curves of Ru1/o-CeO2-ov, Ru1/c-CeO2-ov, and Ru1/r-CeO2-ov. EPR (B) and O 1s XPS (C) spectra of x-CeO2 and
From the EPR characterization results of x-CeO2 and Ru1/x-CeO2-ov samples, it can be seen that all samples exhibited an EPR signal with a g value of about 2.003 [Figure 2B]. Considering the elemental composition of the catalyst, it is suggested that the signal with a g value of 2.003 represents the presence of oxygen vacancies. Compared with the x-CeO2 carriers, the oxygen vacancy signal intensity of the Ru1/x-CeO2-ov series samples increased, especially the signal intensity of Ru1/o-CeO2-ov and Ru1/c-CeO2-ov samples increased by more than twice. It is worth noting that due to the low crystallinity of r-CeO2, it contains more oxygen vacancies, which only increases the oxygen vacancy concentration of the catalyst (Ru1/r-CeO2-ov) by 60% after introducing Ru. Consistent with the EPR detection results, the O 1s XPS spectra of x-CeO2 and Ru1/x-CeO2-ov could more intuitively show that introducing Ru atoms increases the oxygen vacancy concentration of ceria [Figure 2C]. After deconvolution of the O 1s XPS spectrum, two characteristic peaks at ca. 531.8 eV and ca. 529.5 eV could be decomposed [Figure 2D and E]. The former corresponds to
Catalyst performance evaluation
Initially, guaiacol (1a) was used as a model compound to undergo hydrogenative dearomatization to synthesize 2-MCH (2a) over different catalysts at 100 °C and 3 MPa H2 for 3 h [Table 2]. It was observed that none of the three ceria supports (x-CeO2) performed aromatic ring hydrogenation (entries 1-3 in Table 2). It is worth noting that the Ru-based SACs (Ru1/x-CeO2) obtained based on ceria supports
Catalytic hydrogenative dearomatization of 1a to 2a with different catalysts
![]() | ||||
Entry | Catalyst | Conv. of 1a (%) | Sel. of 2aa (%) | Yield of 2a (%) |
1b | o-CeO2 | - | - | - |
2b | c-CeO2 | - | - | - |
3b | r-CeO2 | - | - | - |
4 | Ru1/o-CeO2 | 30.7 | 94.8 | 29.1 |
5 | Ru1/c-CeO2 | 24.2 | 85.5 | 20.7 |
6 | Ru1/r-CeO2 | 28.1 | 80.9 | 22.7 |
7 | Co1/o-CeO2 | - | - | - |
8 | Ni1/o-CeO2 | - | - | - |
9 | Cu1/o-CeO2 | - | - | - |
10 | Fe1/o-CeO2 | - | - | - |
11 | Ru1/o-CeO2-ov | 99.9 | 96.3 | 96.3 |
12 | Ru1/c-CeO2-ov | 80.7 | 84.9 | 68.5 |
13 | Ru1/r-CeO2-ov | 92.1 | 80.2 | 73.9 |
The reaction network of 1a was investigated by adjusting the reaction conditions and controlling the experiments using Ru1/o-CeO2-ov catalyst. First, when the reaction temperature reached 100 °C, 1a could be almost wholly converted, indicating that the reaction was in the kinetic control range below 100 °C [Figure 3A]. As an intermediate, 2a would further undergo demethoxylation reaction to give 3a (Route 1), and the 1a conversion was accelerated with increasing temperature due to kinetics. Under a hydrogen pressure of 1 MPa, the conversion rate of 1a was only 70.7%, while 1a could be completely converted when the hydrogen pressure reached 3 MPa [Figure 3B]. A credible reason is that the increase in hydrogen pressure can increase the hydrogen coverage of the catalyst surface, thereby promoting the hydrogenation of 1a. It is worth noting that after the hydrogen pressure exceeded 3 MPa, further increasing the pressure would lead to a decrease in the yield of 2a and an increase in the yield of 3a, illustrating the conversion of 2a to 3a. Next, the effect of time on the product yield showed that even if 1a had been completely reacted, 3a would continue to increase with the extension of reaction time, further verifying that 2a was an intermediate product of the reaction [Figure 3C]. Although 2a could undergo a C-O bond cleavage reaction, the presence of phenol intermediate was not detected using gas chromatography, resulting in the inability to determine whether 1a undergoes a CAr-OCH3 bond cleavage reaction to generate 3a via Route 2 under experimental conditions. In addition, the extrapolated initial reaction rate (rI) could better evaluate the difficulty of each step in the reaction network of 1a, and given that the conversion of 2a to 3a was a slow process, the average reaction rate (rA) could be used instead of rI. It can be seen that the rI of 1a was
Figure 3. Reaction network analysis of 1a. Effects of temperature (A); hydrogen pressure (B); and time (C) on the 1a conversion and product yield; (D) Proposed 1a reaction network, where Route 1 is that 1a undergoes aromatic ring hydrogenation to generate 2a followed by C-O bond cleavage, while Route 2 involves the first C-O bond cleavage and then the dearomatization process. Reaction conditions: 1 mmol 1a, Ru1/o-CeO2-ov of 0.3 mol% Ru, 3 mL n-octane.
Condition optimization and catalyst stability
According to the results of the previous single-factor investigation experiment [Supplementary Figure 14], a three-factor, three-level response surface experiment model was set up [Supplementary Table 1]. Design-Expert 13 was used to analyze the obtained data [Supplementary Table 2]. It could be seen that the P value of the model was less than 0.0001, R2 = 0.988, and the adjusted R2 = 0.974, all of which were above 90%, indicating that the model’s fit had a minor error with the actual situation and could better use the response value to analyze each factor. From the response surface of temperature-time (AB) parameters to predict the yield of 2a [Figure 4A], it could be seen that the yield of 2a also increased with the temperature due to the influence of kinetics. However, the high reaction temperature (> 100 °C) decreased the yield of 2a, mainly due to the accelerated demethoxylation reaction of 2a at a high temperature. Similarly, due to the continuous conversion of 2a to 3a, the effect of reaction time on the yield of 2a also showed a volcano-shaped curve trend. The response surface of temperature-pressure (AC) parameters and time-pressure (BC) parameters for predicting the yield of 2a found that a too-high pressure did not increase the yield of 2a
Figure 4. Data from response surface optimization and catalyst stability. The 3D surfaces show the effects of temperature-time (A); temperature-pressure (B), and time-pressure (C) parameters on the yield of 2a; The 2D contours show the effects of temperature-time (D); temperature-pressure (E); and time-pressure (F) parameters on the yield of 2a; (G) Heterogeneous catalytic behavior of
After 2 h of reaction, Ru1/o-CeO2-ov was removed from the reaction system to verify the catalyst’s heterogeneous behavior [Figure 4G]. ICP-OES analysis of the liquid mixture after the reaction revealed no Ru signal, which means that the metallic Ru on the catalyst was not leached into the reaction solution
Mechanism study
To explore the effect of the crystal plane structure of ceria on the hydrogenation of aromatic rings, models of single-atom Ru loaded on the (111), (100), and (110) planes of CeO2 containing oxygen vacancies were established and optimized, corresponding to Ru1/o-CeO2-ov, Ru1/c-CeO2-ov, and Ru1/r-CeO2-ov, respectively [Figure 5A]. It was generally believed that the opening of the π bond of the aromatic ring, that was, the addition of the first hydrogen atom, was the rate-determining step of hydrogenation saturation, which means that the first step in the entire saturation process requires the highest energy[45,46]. Therefore, we calculated the energetics of the first-step hydrogenation of the aromatic ring in 1a to compare the hydrogenation performance of catalysts with different preferentially exposed crystal planes. As shown in Figure 5B-D, the Ru1/o-CeO2-ov catalyst represented by the CeO2(111) plane only needs to overcome an energy barrier of 0.22 eV to complete the hydrogenation of the aromatic ring. However, the catalysts represented by the (100) and (110) planes need to overcome 0.34 eV and 0.30 eV, respectively, indicating that guaiacol was more likely to complete the aromatic ring hydrogenation reaction over the Ru1/o-CeO2-ov catalyst from a kinetic point of view. The above conclusions explain well that the Ru1/o-CeO2-ov catalyst activity prepared with octahedral ceria in the experiment is better than that of cubic and rod-shaped ceria.
Figure 5. Mechanism study. (A) Models of single-atom Ru supported on different crystal planes of ceria with and without oxygen vacancies. Transition state energy barriers of 1a hydrogenation over single-atom Ru catalysts containing oxygen vacancies on the (111) (B), (100) (C), and (110) (D) planes; (E) Transition state energy barriers of 1a hydrogenation on a single-atom Ru catalyst without oxygen vacancies on the (111) plane; (F) Transition state energy barriers of CAr-OCH3 bond cleavage of 1a over Ru1/o-CeO2-ov catalyst.
To investigate the effect of ceria oxygen vacancies on the hydrogenation of 1a and the superior selectivity of Ru1/o-CeO2-ov catalyst toward 2a, the energy of aromatic ring hydrogenation on the catalyst corresponding to intact CeO2(111) plane was calculated [Figure 5E]. It could be seen that the transition state energy
Substrate range
Thanks to the 100% utilization of single-atom Ru metal and the Ru-O-Ce active sites rich in oxygen vacancies, Ru1/o-CeO2-ov had a relatively high TOF = 107 h-1 compared with several metal catalysts reported in the literature [Figure 6A][25,27,28,30,47,48]. Subsequently, the aromatic ring hydrogenation performance of a series of lignin-derived phenols was evaluated using Ru1/o-CeO2-ov catalyst [Figure 6B]. The yields of the corresponding hydrogenated products of the guaiacol derivatives (1a-1d) obtained after the reaction could reach a good level (88%-96%). Surprisingly, since phenol derivatives were not affected by methoxy groups, the yields of the corresponding hydrogenated products could reach over 96% (2e-2h). The aromatic ring could also reach complete saturation when using syringyl 2,6-dimethoxyphenol (1i) as a substrate. Unfortunately, because higher temperatures were required to activate the aromatic ring in 1i, more than 60% of the product was a mixture of 2a (46% yield) and 2e (15% yield), and the corresponding hydrogenation product 2i was only 31%. Finally, the aromatic ring hydrogenation performance of
Figure 6. (A) TOF comparison of 1a hydrogenation reaction on Ru1/o-CeO2-ov and literature catalysts; (B) Hydrogenation performance of different lignin-derived phenols catalyzed by Ru1/o-CeO2-ov (a 150 °C; b 160 °C, 4 h; c 4 h; d 200 °C, 3 MPa H2, 4 h); 2D HSQC NMR spectra of authentic lignin oil in the aromatic region (C); methoxy region (D), and aliphatic region (E) before and after the reaction. 2D HSQC NMR: Two-dimensional heteronuclear single quantum coherence nuclear magnetic resonance.
CONCLUSIONS
In summary, we developed a versatile catalytic strategy to rapidly hydrogenate the aromatic ring of guaiacols while suppressing C-O bond cleavage by tuning the crystal plane of the ceria and the oxygen vacancies of the Ru1/o-CeO2-ov catalyst. The Ru1/o-CeO2-ov catalyst had excellent aromatic ring hydrogenation ability due to the formation of Ru-O-Ce active sites. DFT calculations elaborated that the oxygen vacancies and preferentially exposed (111) plane of ceria could reduce the transition state energy barrier of aromatic ring hydrogenation, which was only 40% of the CAr-OCH3 bond cleavage energy. Response surface experiment optimization revealed that temperature and time were more sensitive to the selectivity of the target product than hydrogen pressure, and the yield of 2-MCH could exceed 97% under the optimal process conditions. The Ru1/o-CeO2-ov catalyst was able to completely hydrogenate almost all lignin-derived phenols, including real lignin oil, which provides a new application direction for CeO2 crystal surface regulation, namely, the transformation of lignin-derived phenols into value-added chemicals.
DECLARATIONS
Authors’ contributions
Experimentation: Wang, B.
Writing - review and editing: Wang, B.; Yan, X.; Li, H.
Investigation: Wang, B.; Yan, X.; Zhou, M.
Methodology: Wang, B.; Yan, X.; Zhou, M.; Li, H.
Formal analysis: Wang, B.; Yan, X.
Supervision, conceptualization: Li, H.
Availability of data and materials
The related data, deposited in the individual repositories of the participating institutions, will be provided upon request.
Financial support and sponsorship
The authors acknowledge the financial support from the National Natural Science Foundation of China (52466011, 22368014, 22478087), Marine Science First-Class Subject, Beibu Gulf University, Natural Science Foundation of Guangxi Zhuang Autonomous Region (2024GXNSFAA010382, 2025GXNSFAA069590), and Guizhou Provincial S&T Project (GCC [2023]011, ZK [2022]011).
Conflicts of interest
Li, H. is the Guest Editor of the Special Issue of “Biofuels and Carbon Resource Utilization” of the journal of Energy Materials and was not involved in any steps of editorial processing, notably including reviewer selection, manuscript handling, or decision making. The other authors declare that there are no conflicts of interest.
Ethical approval and consent to participate
Not applicable.
Consent for publication
Not applicable.
Copyright
© The Author(s) 2025.
Supplementary Materials
REFERENCES
1. Ashokkumar, V.; Venkatkarthick, R.; Jayashree, S.; et al. Recent advances in lignocellulosic biomass for biofuels and value-added bioproducts - a critical review. Bioresour. Technol. 2022, 344, 126195.
2. Pompei, S.; Grimm, C.; Schiller, C.; Schober, L.; Kroutil, W. Thiols act as methyl traps in the biocatalytic demethylation of guaiacol derivatives. Angew. Chem. Int. Ed. 2021, 60, 16906-10.
3. Smet G, Bai X, Maes BUW. Selective C(aryl)-O bond cleavage in biorenewable phenolics. Chem. Soc. Rev. 2024, 53, 5489-551.
4. Zhao, Y.; Zhan, J.; Hu, R.; et al. Water-assisted catalytic transfer hydrogenation of guaiacol to cyclohexanol over Ru/NiAl2O4. Chem. Eng. J. 2024, 485, 149934.
5. Wang, B.; Huang, J.; Wu, H.; Yan, X.; Liao, Y.; Li, H. Synergy of heterogeneous Co/Ni dual atoms enabling selective C-O bond scission of lignin coupling with in-situ N-functionalization. J. Energy. Chem. 2024, 92, 16-25.
6. Smet G, Bai X, Mensch C, Sergeyev S, Evano G, Maes BUW. Selective nickel-catalyzed hydrodeacetoxylation of aryl acetates. Angew. Chem. Int. Ed. 2022, 61, e202201751.
7. Wu, R.; Meng, Q.; Yan, J.; et al. Intermetallic synergy in platinum-cobalt electrocatalysts for selective C-O bond cleavage. Nat. Catal. 2024, 7, 702-18.
8. Lang, M.; Li, H. Toward value-added arenes from lignin-derived phenolic compounds via catalytic hydrodeoxygenation. ACS. Sustainable. Chem. Eng. 2022, 10, 13208-43.
9. Zhang, J.; Sun, J.; Wang, Y. Recent advances in the selective catalytic hydrodeoxygenation of lignin-derived oxygenates to arenes. Green. Chem. 2020, 22, 1072-98.
10. Lang, M.; Li, H. Heterogeneous metal-based catalysts for cyclohexane synthesis from hydrodeoxygenation of lignin-derived phenolics. Fuel 2023, 344, 128084.
11. Wong, S. S.; Shu, R.; Zhang, J.; Liu, H.; Yan, N. Downstream processing of lignin derived feedstock into end products. Chem. Soc. Rev. 2020, 49, 5510-60.
12. Winterton, N. The green solvent: a critical perspective. Clean. Technol. Environ. Policy. 2021, 23, 2499-522.
13. Marco-Contelles, J.; Molina, M. T.; Anjum, S. Naturally occurring cyclohexane epoxides: sources, biological activities, and synthesis. Chem. Rev. 2004, 104, 2857-900.
14. Liu, L.; Liu, L.; Zhang, K.; et al. Selective conversion of cyclohexene to 2-methoxycyclohexanol over molybdenum oxide on beta zeolite. Ind. Eng. Chem. Res. 2023, 62, 16207-14.
15. Feng, J.; Shang, Y.; Zhang, Y. Research on synthesis and thermodynamic properties of 2-methoxycyclohexanol. J. Therm. Anal. Calorim. 2018, 131, 2197-203.
16. Jiang, Y.; Huang, J.; Hunger, M.; Maciejewski, M.; Baiker, A. Comparative studies on the catalytic activity and structure of a Cu-MOF and its precursor for alcoholysis of cyclohexene oxide. Catal. Sci. Technol. 2015, 5, 897-902.
17. Obeso, J. L.; Gabriel, F. J.; Flores, C. V.; et al. Lewis acid-catalyzed ring-opening alcoholysis of cyclohexene oxide: the role of open metal sites in the Bi(III)‐based metal-organic framework SU-101. ChemCatChem 2023, 15, e202300471.
18. Abbasi, K. R.; Shahbaz, M.; Zhang, J.; Irfan, M.; Alvarado, R. Analyze the environmental sustainability factors of China: the role of fossil fuel energy and renewable energy. Renew. Energy. 2022, 187, 390-402.
19. Zhou, H.; Wang, H.; Sadow, A. D.; Slowing, I. I. Toward hydrogen economy: Selective guaiacol hydrogenolysis under ambient hydrogen pressure. Appl. Catal. B. Environ. 2020, 270, 118890.
20. Nakagawa, Y.; Ishikawa, M.; Tamura, M.; Tomishige, K. Selective production of cyclohexanol and methanol from guaiacol over Ru catalyst combined with MgO. Green. Chem. 2014, 16, 2197-203.
21. Hensley, A. J.; Bray, J.; Shangguan, J.; Chin, Y. H.; Mcewen, J. S. Catalytic consequences of hydrogen addition events and solvent-adsorbate interactions during guaiacol-H2 reactions at the H2O-Ru(0001) interface. J. Catal. 2021, 395, 467-82.
22. Wang, B.; Zhou, P.; Yan, X.; Li, H.; Wu, H.; Zhang, Z. Cooperative catalysis of Co single atoms and nanoparticles enables selective CAr-OCH3 cleavage for sustainable production of lignin-based cyclohexanols. J. Energy. Chem. 2023, 79, 535-49.
23. Nania, C.; Bertini, M.; Gueci, L.; Ferrante, F.; Duca, D. DFT insights into competing mechanisms of guaiacol hydrodeoxygenation on a platinum cluster. Phys. Chem. Chem. Phys. 2023, 25, 10460-71.
24. Zhang, K.; Meng, Q.; Wu, H.; et al. Selective hydrodeoxygenation of aromatics to cyclohexanols over ru single atoms supported on CeO2. J. Am. Chem. Soc. 2022, 144, 20834-46.
25. Wang, X.; Zhu, S.; Wang, S.; Wang, J.; Fan, W.; Lv, Y. Ni nanoparticles entrapped in nickel phyllosilicate for selective hydrogenation of guaiacol to 2-methoxycyclohexanol. Appl. Catal. A. Gen. 2018, 568, 231-41.
26. Yamaguchi, A.; Murakami, Y.; Yamazaki, K.; Shirai, M.; Hiyoshi, N. Stereoselective hydrogenation of guaiacol to cis-2-methoxycyclohexanol using supported rhodium catalysts in supercritical carbon dioxide. Catal. Today. 2024, 425, 114356.
27. Kumar, A.; Kumar, J.; Bhaskar, T. High surface area biochar from sargassum tenerrimum as potential catalyst support for selective phenol hydrogenation. Environ. Res. 2020, 186, 109533.
28. Chen, J.; Ji, J.; Tu, T. Selective hydrogenation of phenols to cyclohexanols catalyzed by robust solid NHC-Rh coordination assemblies in water. Green. Chem. 2023, 25, 7541-6.
29. Qian, W.; Lin, L.; Qiao, Y.; et al. Ru subnanoparticles on N-doped carbon layer coated SBA-15 as efficient Catalysts for arene hydrogenation. Appl. Catal. A. Gen. 2019, 585, 117183.
30. Wang, Q.; De, B. M. C. M.; Safonova, O. V.; et al. Tunable catalysis by reversible switching between Ru(III) single sites and Ru0 clusters in solid micelles. J. Catal. 2023, 426, 336-44.
31. Wang, A.; Li, J.; Zhang, T. Heterogeneous single-atom catalysis. Nat. Rev. Chem. 2018, 2, 65-81.
32. Li, L.; Chang, X.; Lin, X.; Zhao, Z. J.; Gong, J. Theoretical insights into single-atom catalysts. Chem. Soc. Rev. 2020, 49, 8156-78.
33. Gong, X.; Song, P.; Han, C.; Xiao, Y.; Mei, X.; Xu, W. Heterogeneous single-atom catalysts for energy process: recent progress, applications and challenges. Energy. Mater. 2023. DOI: 10.20517/energymater.2022.82.
34. Wang, W.; Li, S.; Qiang, Q.; et al. Catalytic refining lignin-derived monomers: seesaw effect between nanoparticle and single-atom Pt. Angew. Chem. Int. Ed. 2024, 63, e202404683.
35. Zhang, L.; Shang, N.; Gao, S.; et al. Atomically dispersed Co catalyst for efficient hydrodeoxygenation of lignin-derived species and hydrogenation of nitroaromatics. ACS. Catal. 2020, 10, 8672-82.
36. Li, J.; Ge, J.; Zhao, Y.; et al. Lignin-tailored fabrication of Ni single atom catalyst with Ni-N3 active site for efficient and selective catalytic transfer hydrogenation of lignin-derived aldehydes. Chem. Eng. J. 2024, 496, 154315.
37. Guo, H.; Zhao, J.; Chen, Y.; et al. Mechanistic insights into hydrodeoxygenation of lignin derivatives over Ni single atoms supported on Mo2C. ACS. Catal. 2024, 14, 703-17.
38. Gao, X.; Ma, R.; Liu, Z.; Wang, S.; Wu, Y.; Song, G. Hydrodeoxygenation of lignin-derived phenols into cycloalkanes by atomically dispersed Pt-polyoxometalate catalysts. Appl. Catal. B. Environ. Energy. 2024, 352, 124059.
39. Jiang, F.; Wang, S.; Liu, B.; et al. Insights into the influence of CeO2 crystal facet on CO2 hydrogenation to methanol over Pd/CeO2 catalysts. ACS. Catal. 2020, 10, 11493-509.
40. Li, H.; Zha, S.; Zhao, Z.; et al. The nature of loading-dependent reaction barriers over mixed RuO2/TiO2 catalysts. ACS. Catal. 2018, 8, 5526-32.
41. Huang, H.; Dai, Q.; Wang, X. Morphology effect of Ru/CeO2 catalysts for the catalytic combustion of chlorobenzene. Appl. Catal. B. Environ. 2014, 158-159, 96-105.
42. Campbell, C. T.; Peden, C. H. Oxygen vacancies and catalysis on ceria surfaces. Science 2005, 309, 713-4.
43. Vayssilov, G. N.; Lykhach, Y.; Migani, A.; et al. Support nanostructure boosts oxygen transfer to catalytically active platinum nanoparticles. Nat. Mater. 2011, 10, 310-5.
44. Liu, P.; Zheng, C.; Liu, W.; Wu, X.; Liu, S. Oxidative redispersion-derived single-site Ru/CeO2 catalysts with mobile Ru complexes trapped by surface hydroxyls instead of oxygen vacancies. ACS. Catal. 2024, 14, 6028-44.
45. Zhao, Z.; Bababrik, R.; Xue, W.; et al. Solvent-mediated charge separation drives alternative hydrogenation path of furanics in liquid water. Nat. Catal. 2019, 2, 431-6.
47. Jiang, W.; Cao, J.; He, Z.; et al. Highly selective hydrogenation of arenes over Rh nanoparticles immobilized on α-Al2O3 support at room temperature. Chem. Eng. Sci. 2023, 270, 118544.
Cite This Article
How to Cite
Download Citation
Export Citation File:
Type of Import
Tips on Downloading Citation
Citation Manager File Format
Type of Import
Direct Import: When the Direct Import option is selected (the default state), a dialogue box will give you the option to Save or Open the downloaded citation data. Choosing Open will either launch your citation manager or give you a choice of applications with which to use the metadata. The Save option saves the file locally for later use.
Indirect Import: When the Indirect Import option is selected, the metadata is displayed and may be copied and pasted as needed.
About This Article
Copyright
Data & Comments
Data
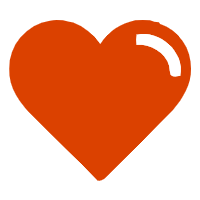
Comments
Comments must be written in English. Spam, offensive content, impersonation, and private information will not be permitted. If any comment is reported and identified as inappropriate content by OAE staff, the comment will be removed without notice. If you have any queries or need any help, please contact us at [email protected].