Understanding Mg-ion deposition behavior on MgBi alloy in solid-state form
Abstract
Mg alloys have frequently been studied as anodes for Mg-ion batteries due to their high specific capacity and low electrochemical potential. In the present study, we investigated the interfacial stability of MgBi alloy anodes with solid-state electrolytes. The bubble-like solid electrolyte interface (SEI) was observed between the MgBi alloy anode and Mg(BH4)2·1.9NH3 solid-state electrolyte, leading to the unstable Mg stripping/plating on the MgBi alloy. Theoretical simulations suggest that the bubble-like SEI originates from the different Mg-ion dynamics on the eutectic region (e.g., Mg + Mg3Bi2 phases) and the Mg matrix. The addition of MgBr2·2NH3 nanoparticles in Mg(BH4)2·1.9NH3 suppresses the formation of a bubble-like SEI through the etching effect of Br- ions. Consequently, interfacial resistance is lowered and the interfacial stability is drastically enhanced, e.g., Mg stripping/plating for over 1,200 cycles at 0.1 mA cm-2 with a low overpotential around 0.05 V.
Keywords
INTRODUCTION
As one of the most abundant elements globally, constituting approximately 2.3 wt.% of the Earth’s crust, magnesium (Mg) is recognized for its low-cost material benefits, possessing superior properties compared to lithium. The development of Mg-ion batteries (MIBs), especially the batteries in the solid-state form, is limited by the slow Mg2+ migration both in the solid electrolyte and electrode[1]. Recently, great progress has been made in Mg(BH4)2-based materials as solid-state electrolytes (SSEs). By substituting[BH4]- with other anions {e.g., [NH2]-[2-5]} or introducing neutral molecules [e.g., NH2(CH2)2NH2[6], diglyme[7], NH3BH3[8] and NH3[9,10]], the [BH4] tetrahedral cages that confine the Mg2+ cations can be disrupted leading to significant improvement in Mg2+ conductivity. Further, the addition of metal oxide nanoparticles improves the ionic conductivity of Mg(BH4)2∙xNH3 to 10-4 S cm-1 at room temperature[10-12], owing to the coordination-unlock effect of oxygen vacancies on the surface of metal oxide nanoparticles; the nanoparticles also show high reactivity[13]. However, the Mg metal anode is noted for its susceptibility to passivation and poor compatibility with electrolytes[14], decreasing the interfacial stability[15-17].
Electrolyte additives and the structure engineering of anodes have been chosen to eliminate the passivation effect. Combining with density functional theory (DFT) calculations and thermodynamic analysis, compounds such as MgSiN2, MgSe, MgS, and MgX2 (X = Cl, Br, and I) are identified as promising anode coating materials due to their reasonable Mg migration barriers[18]. The addition of both MgCl2 and MgBr2 results in the dissolution of the native passive layer and the formation of a passivating film that permits the diffusion of Mg2+[17,19]. This behavior, associated with the reactivity of Cl- anions, leads to homogeneous plating and stripping of Mg[19]. Impressively, our previous work reported that the in situ decoration of MgBr2∙2NH3 nanoparticles on the surface of Mg(BH4)2∙1.9NH3 offers long-term interfacial stability towards the Mg metal anode[20]. The behavior of Br- leads to the uniform Mg deposition on the surface of Mg metal similar to Cl- anions in organic electrolytes.
However, it is crucial to highlight that in the Mg anode, only the α-Mg matrix phase is present[20], making it difficult to directly observe the formation of the passivation layer from the Mg(BH4)2∙1.9NH3 electrolyte. Additionally, the corrosion process induced by Br- ions in a single-phase Mg anode is particularly complex. The interaction between Br- ions and the α-Mg surface can result in localized corrosion phenomena. This complexity is further heightened by the dynamic nature of the electrolyte interface, where passivation layer formation and corrosion occur simultaneously. The lack of structural diversity in the α-Mg phase restricts our ability to identify specific reaction pathways or transient states during solid electrolyte interface (SEI) formation. Addressing these challenges requires advanced characterization methods and a deeper understanding of the electrochemical mechanisms at play, particularly in the context of the
To further investigate the corrosion behavior, a Mg alloy containing a second phase is ideal, especially for SSEs. In recent years, alloy anodes have gained significant attention due to their unique advantages and properties. Elements from groups IIIA, IVA, and VA (denoted as M) can theoretically form MgxM alloys with magnesium, offering high theoretical specific capacities at relatively low alloying potentials. Moreover, alloy materials can exhibit new properties that single metals do not possess, potentially creating synergistic effects that enhance magnesium storage performance. A variety of alloys (i.e., Mg3Bi2[21], Mg2Sn[22,23],
In contrast to Sn, bismuth (Bi) exhibits higher reversibility as an anode material[27]. Theoretical calculations indicate that Mg-ion mobility in Bi anodes is higher than that of Li ions[28]. However, Bi is limited by lower theoretical specific capacity (384 mAh g-1) and its (de)magnesiation occurs at a high voltage of 0.28 V (vs. Mg2+/Mg)[29], which restricts its practical applicability. These challenges highlight the need for significant improvements and structural optimizations to enhance its performance. On the other hand, the rhombic crystal structure of Bi promotes the formation of alloys that offer a high-volume capacity (3,783 mA h cm-3), comparable to that of Mg. Therefore, Bi is considered to be the best anode model for future studies of complete MIB systems[27]. Additionally, MgBi alloys circumvent the initial slow electrochemical alloying process between Mg and Bi, further enhancing their potential as anode materials.
In this work, we investigated the interfacial stability of a MgBi alloy anode with a SSE. For the first time, we observed the formation of a bubble-like SEI between MgBi and Mg(BH4)2∙1.9NH3 electrolytes, which impedes the stable Mg stripping/ platting. The introduction of MgBr2·2NH3 nanoparticles in the electrolyte could suppress the bubble-like SEI, enabling the long-term stable stripping/plating over 600 h at
EXPERIMENTAL
Material preparation
Mg(BH4)2∙1.9NH3 (labeled as MBN) and Mg(BH4)2∙1.9NH3-32 wt.% MgBr2∙2NH3 (labeled as MBN-Br) were synthesized according to the protocol described in the literature[20]. Metallic Mg (99.99%) and Mg20Bi alloys, composed of 80% Mg and 20% Bi, were repeatedly polished in an argon-filled glove box before use and then cut into 6mm diameter pieces for electrochemical measurements.
Structural characterization
X-ray diffraction (XRD) data were collected at room temperature using a Rigaku SmartLab diffractometer equipped with a rotating Cu anode (Cu Kα radiation, 2 kW, λ = 1.54056 Å). Before these analyses, the sheets were embedded in conductive cold-set resin and sanded to a 3,000-mesh finish, followed by mechanical polishing and etching. Due to the varying potentials between the phases in the alloy, each phase experiences different degrees of corrosion, leading to distinct microstructural features. The pristine MgBi alloy sheets were then etched using a solution composed of 10 g of oxalic acid in 100 mL of deionized (DI) water. The microstructure and element analyses of the alloy sheets were examined through optical microscopy (OM) and scanning electron microscopy (SEM). SEM analysis was conducted using SU-8200 equipped with an Oxford X-Max energy-dispersive X-ray spectrometer (EDS). The samples were loaded into a protective accessory and sealed in an argon atmosphere. The X-ray photograph spectroscopy (XPS) spectra were recorded on Thermo Scientific K-Alpha+, an XPS system. The spectra were corrected based on C 1s binding energy at 284.8 eV. Sub-surfaces of different depths were obtained by different argon ion etching times.
Electrochemical measurements
All pellets were prepared in an Ar-filled glovebox with a diameter of 6 mm and a thickness of ~1 mm under a pressure of 0.3 GPa and assembled in a Swagelok-type cell. Electrochemical impedance spectroscopy (EIS) and cyclic voltammetry (CV) tests were using the Multi Autolab M204 electrochemical workstation. A symmetric Mg (Mg20Bi) | MBN (MBN-Br) | Mg (Mg20Bi) cell was used for EIS test. The EIS measurements were conducted in a frequency range of 0.1 Hz to 1 MHz with an alternating current amplitude of 5 mV. An asymmetric Mg (Mg20Bi) | MBN | SS cell configuration was used for CV (scan rate of 10 mV s-1). Galvanostatic cycling was conducted with a LANDCT2001A battery tester at 323 K. For the galvanostatic cycling in the symmetric Mg (Mg20Bi) | MBN (MBN-Br) | Mg (Mg20Bi) cell.
Computational methods
DFT was performed with Vienna Ab-initio Simulation Package (VASP) 6.3[30]. Perdew-Burke-Ernzerhof (PBE) was utilized for exchange-correlation functional[31]. The wave functions were expanded in plane waves with a kinetic energy cutoff of 450 eV. We used Projector Augmented Wave (PAW) potentials to describe the interaction between the ions and electrons[32]. A Gaussian smearing of 0.02 eV for the
RESULTS AND DISCUSSION
Figure 1 shows the microstructure and phase analysis results of the Mg20Bi binary alloy. The alloy contains two distinct phases: Mg matrix (black area) with surface area ranging from approximately 3,200 μm2 (feature size: 80 µm) to 16,200 μm2 (feature size: 180 µm) and the Mg3Bi2 phase (white area) as the grain boundaries, as shown in Figure 1A. The EDS mapping analysis in Figure 1B and C confirms the presence of Mg matrix surrounded by Bi-enriched regions. The proportion of the Mg3Bi2 phase in Mg20Bi alloys is estimated to be 13.5 wt.% based on the statistical results from OM in Supplementary Figure 1, which is consistent with the phase composition of the alloy[35]. As presented in Figure 1D, elemental composition analysis results indicate that the Mg matrix (Point 1 in Figure 1A) contains 96.7 wt.% Mg and 3.3 wt.% Bi, while the Mg3Bi2 phase (Point 2 in Figure 1A) contains 13.5 wt.% Mg and 86.5 wt.% Bi. The XRD pattern in Figure 1E indicates the presence of three phases in Mg20Bi alloys: 87.3 wt.% α-Mg, 9.1 wt.% Mg3Bi2 and 3.6 wt.% Bi2O3, as shown in Figure 1F. These results are consistent with the phase composition observed through OM [Supplementary Figure 1].
Figure 1. Structural characterization of Mg20Bi alloy. (A-C) SEM images and corresponding EDS mapping of Mg20Bi alloy; (D) EDS results for the two points indicated in Figure 1A. Mg and Bi elements are represented in yellow and green, respectively; (E) Rietveld refinement of XRD patterns for Mg20Bi, showing observed (grey circles) and calculated (line) curves, and a different curve below (grey line), R/E = 1.7; (F) The phase compositions. SEM: Scanning electron microscopy; EDS: Energy-dispersive X-ray spectrometer; XRD:
The electrochemical stability of Mg20Bi alloy anodes with MBN and MBN-Br is compared by CV measurements with a scanning rate of 10 mV s-1 at 50 °C, as illustrated in Supplementary Figure 2. The prominent oxidation peaks observed in both electrolytes indicate their compatibility with Mg20Bi alloys. Notably, a peak current density of 0.33 mA cm-2 observed for MBN-Br surpasses that for MBN electrolyte (0.002 mA cm-2) by around 160 times, suggesting a much smaller interfacial resistance between Mg20Bi and MBN-Br.
The voltage profile of a symmetric cell, Mg20Bi | MBN-Br | Mg20Bi, at different current densities at 50 °C is shown in Supplementary Figure 3. Stable Mg2+ stripping/plating is initially observed at 0.5 mA cm-2. The extended test of 1200 cycles over 600 h was performed at a current density of 0.1 mA cm-2 to assess the compatibility between the anode and electrolyte, as depicted in Figure 2A. The Mg20Bi | MBN | Mg20Bi symmetric cell shows significant polarization; i.e., the overpotential continuously increases from an initial value of 0.18 V to 0.21 V at 100 h, 0.38 V at 300 h, and further to 0.57 V at 500 h. In contrast, the
Figure 2. Electrochemical performance of Mg20Bi alloy anodes with different SSEs. (A) Long-term cycling curves of Mg20Bi alloy anodes with MBN and MBN-Br for a symmetric cell at 0.1 mA cm-2. Enlargements show the detailed voltage plateau of Mg stripping/plating at a selected duration. Nyquist plots of the Mg20Bi | SSEs | Mg20Bi symmetric cell; SSEs (B) MBN and (C) MBN-Br. All measurements are conducted at T = 50 °C. SSEs: Solid-state electrolytes; MBN: Mg(BH4)2·1.9NH3; MBN-Br: Mg(BH4)2·1.9NH3-32 wt.% MgBr2·2NH3.
The variation of impedance during the Mg stripping/plating process of Mg20Bi | MBN | Mg20Bi and Mg20Bi | MBN-Br | Mg20Bi symmetric cells is recorded, as shown in Figure 2B and C, respectively. The EIS spectra of both symmetric cells, characterized by a semicircle as a typical feature of an ionic conductor, rule out the possibility of a short circuit, as shown in Supplementary Figure 4. The impedance of Mg20Bi | MBN | Mg20Bi cell displays a significant increase from 1 MΩ up to 20 MΩ over 600 h. In contrast, the impedance of the Mg20Bi | MBN-Br | Mg20Bi cell [Figure 2C] varies much more slightly, i.e., from an initial value of 1500 Ω to 1900 Ω at 600 h, which is consistent with the stable Mg stripping/plating behavior observed in the literature [Supplementary Table 1].
To understand the differences in impedance changes over the cycling time, the microstructure of the Mg20Bi alloy anode after cycling with MBN and MBN-Br is investigated. Figure 3A shows the surface morphology of Mg20Bi alloys before the cycling test, which is flat, with visible scratches resulting from sandpapering. After 600 h cycling in the Mg20Bi | MBN | Mg20Bi symmetric cell, as shown in Figure 3B, the surface of the Mg20Bi alloy comprises bubble-like grains with a surface area distribution between 3,200 μm2 (feature size: 70 μm) and 9,900 μm2 (feature size: 110 μm), which is similar to the surface area range of the initial alloy metallograph of the matrix Mg [Figure 1A]. Further, EDS mapping results in Figure 3C indicate that the bubble-like grains contain elements of Mg, B, and Bi. In particular, elements Mg and Bi are majorly distributed inside the bubble, whereas element B is concentrated at the edges of the bubble, suggesting that the edges of the bubble consist of electrolytes. The XRD pattern of the Mg20Bi alloy anode after 600 h of Mg stripping/plating is shown in Supplementary Figure 5. The peaks of Mg and Mg3Bi2 phases shift by approximately 0.32° toward lower angles, indicating lattice expansion due to Mg ion insertion.
Figure 3. Mg20Bi alloy anode after cycling in Mg20Bi | MBN | Mg20Bi symmetric cell. SEM of Mg20Bi alloy anode: (A) 0 h and (B) after 600 h; (C) EDS mapping of the enlarged part in Figure 3B; (D) XPS spectra at different etching times: Mg 2p and Bi 4f in Mg20Bi alloy anode after 600 h stripping/plating. MBN: Mg(BH4)2·1.9NH3; SEM: Scanning electron microscopy; EDS: Energy-dispersive X-ray spectrometer; XPS: X-ray photograph spectroscopy.
Ex situ Mg 2p and Bi 4f XPS spectra are conducted to investigate the Mg and Bi chemical states at different etching times, as shown in Figure 3D. The peak of Mg 2p observed at 50.2 eV at an etching time of 0 s is assigned to MgII. At the same time, the doublet peaks of Bi 4f5/2 and Bi 4f7/2 appear around 163 eV and 158 eV, respectively. The Bi 4f doublet peaks at 0s confirm the presence of BiIII and Bi0, with the proportion of Bi0 being 51.2%, relating to the metallic state from the Mg20Bi solid solution. The 48.8% BiIII originates from intermetallic compounds formed by the newly deposited Mg with Bi, primarily leading to the formation of the intermetallic compound Mg3Bi2. When the etching time is increased to 150 s, the peak of Mg 2p
Combined with the SEM-EDS spectra, the Mg ion stripping/plating mechanism between the MBN electrolyte and the Mg20Bi alloy anode is as follows: During discharge, the high-potential Mg3Bi2 phase discharges first, allowing Mg2+ to diffuse into the electrolyte. The remaining highly reactive Bi and Mg from the anode react with the MBN electrolyte, forming a solid electrolyte interphase (SEI), primarily on the
Figure 4A-C depicts the surface morphology of the Mg20Bi alloy cycled with MBN-Br at different times. At the initial cycle time, the surface of the alloy shows some dependent bubble-like grains with a surface area of 6 μm2 (feature size: 3 μm), increasing to 150 μm2 (feature size: 115 μm) at 200 h. Surprisingly, at the same time, the “bubbles” break, which may be attributed to the etching effect of Br-[19]. When the cycle time is increased to 600 h, the bubble-like grains on the surface disappear and a two-phase structure with features measuring up to 3,000 μm2 (feature size: 60 μm) in surface area is observed. In Figure 4C, corrosion pits are visible in the Mg matrix [Supplementary Figure 6], accompanied by Br accumulation [Figure 4D], suggesting that Br- ions contribute to the etching process, which aligns with the proposed mechanism.
Figure 4. Mg20Bi alloy anode after cycling in Mg20Bi | MBN-Br | Mg20Bi symmetric cell. SEM of Mg20Bi alloy anode: (A) 50 h; (B) 200 h and (C) 600 h; (D) EDS mapping of Figure 4C; (E) XPS spectra at different etching times: Mg 2p and Bi 4f in Mg20Bi alloy anode after 600 h stripping/plating; (F) Variations in BiIII proportion in the Mg20Bi alloy at different cycling and etching times. MBN-Br:
Additionally, EDS mapping of the Mg20Bi alloy anode cycled for 50 and 200 h [Supplementary Figure 7] shows Br concentrated at the edges of the broken bubble-like grains, indicating the presence of electrolytes, while Mg is more concentrated in the center of these grains.
Ex situ Mg 2p and Bi 4f XPS spectra of Mg20Bi alloy anodes are shown in Figure 4E. The Mg 2p spectra at
We compared the variations in BiIII proportion in the Mg20Bi alloy at different cycling and etching times, as shown in Figure 4F. When the cycling time is at 50 h, BiIII proportion decreases from 60% at 0 s to 15% at 150 s and 300 s, consistent with the formation of bubble-like SEI as in MBN. A similar trend is observed after cycling for 200 h, with a decrease from 53% at 0 s to 49% at 150 s and 300 s, accompanied by a significant overall decrease in BiIII proportion, corresponding to the broken of the bubble-like SEI. Finally, when the cycling time was further increased to 600 h, the BiIII proportion is uniformly distributed with 42% at 0 s and 45% at 150 s and 300 s, indicating the deeply uniform Mg stripping/plating process on the Mg20Bi alloy anode.
To compare the magnesiophilicity of Mg20Bi alloys, the interaction between the Mg atom and Mg/Mg3Bi2 phase surface was investigated by DFT calculations. Figure 5A illustrates the optimized configurations of Mg atoms adsorbed on the surface of Mg (101) and Mg3Bi2 (011) at different positions. There are different adsorption sites on the Mg-Mg3Bi2 surfaces, and the Eads is much more negative at the top of Bi atoms
Figure 5. The interaction between Mg atom and the Mg/Mg3Bi2 phase surface. (A) Adsorption configurations and the corresponding adsorption energies of a Mg atom on the surfaces of Mg (101) and Mg3Bi2 (011); (B-C) The calculated Mg migration energy barriers in Mg3Bi2 (011) and Mg (101), respectively. Here, the orange and purple balls represent the Mg and Bi elements, respectively.
The diffusion energy barriers of Mg ions on the surfaces of Mg and Mg3Bi2 are also investigated. The calculated diffusion energy barriers for Mg ions on the Mg3Bi2 and Mg surfaces are 0.23 eV and 0.37 eV, respectively, as illustrated in Figure 5B and C. These results agree with the experimental observations discussed earlier. In comparison to the bare Mg phase, the Mg3Bi2 phase facilitates faster migration of Mg2+ ions. Additionally, the high affinity for Mg2+ and the rapid Mg2+ diffusion of the Mg3Bi2 phase effectively enhance the uniform flux of Mg2+ and promote the smooth deposition of Mg[25,38].
Mg metal anodes are of interest due to their susceptibility to passivation and poor compatibility with electrolytes. In conventional organic non-aqueous electrolytes, such as those containing ClO4-, PF6-, and
In the present study, the strategy and possible formation mechanism of the Mg stripping/plating process on MgBi alloy anodes with Mg(BH4)2∙1.9NH3 and Mg(BH4)2∙1.9NH3-MgBr2∙2NH3 are summarized in Figure 6, each illustrated in four distinct stages. At the initial stage (Stage I), Mg2+ ions directly electro-dissolute from the MgBi alloy anode in the absence of the passive SEI. The stripping potential (Estripping) is mainly determined by the charge-transfer process (Ect), with the values of 0.18 V for Mg(BH4)2∙1.9NH3 and 0.05 V for Mg(BH4)2∙1.9NH3-MgBr2∙2NH3, respectively [Figure 2A]. During the subsequent plating process, the high affinity of Mg2+ and the rapid diffusion of Mg2+ within the Mg3Bi2 phase significantly enhance the homogeneous fluxes of Mg2+, promoting the fast kinetics of Mg deposition on the Mg3Bi2 phase [Figure 5]. As a result, the tiny passive bubble-like SEI will form on the surface of MgBi foil (Stage II). In this stage, the Estripping slightly increases due to the added ionic diffusion through interphases (Eint)[42], resulting in the increase of impedance [Figure 2B and C].
Figure 6. Schematic diagram of SEI evolution and Mg deposition behavior for (A) Mg(BH4)2·1.9NH3 and (B) Mg(BH4)2·1.9NH3-
This bubble-like SEI, similar to “hot spots” on Mg foil at high current densities[43], expands and increases over cycling time, leading to a nonuniform Mg deposit on the anode (Stage III in Figure 6A). As a result,
CONCLUSION
We have investigated the Mg20Bi alloy as an anode for MIBs, focusing on its interfacial stability with solid state electrolytes (SSEs). Bubble-like SEI is formed between the MgBi alloy and
DECLARATIONS
Authors’ contributions
Investigation, DFT calculation, writing - original draft: Wang, Q.
Software supervision, writing - review and editing: Li, H.
Material characterization: Xu, T.
Supervision, funding acquisition, writing - review and editing: Yan, Y.
Supervision: Chen, Y.
Availability of data and materials
The data that support the findings of this study are available from the corresponding author upon reasonable request.
Financial support and sponsorship
This work was financially supported by the National Natural Science Foundation of China (No. 21975168). The authors appreciate Dr. Zhu, Y. for his assistance with SEM measurement. The authors would like to thank Shiyanjia Lab (www.shiyanjia.com) for the XPS tests. Wang, Q. acknowledges the financial support from the China Scholarship Council (202306240092). Li, H. acknowledges the Center for Computational Materials Science, Institute for Materials Research, Tohoku University for the use of MASAMUNE-IMR (No. 202312-SCKXX-0203), and the Institute for Solid State Physics (ISSP) at the University of Tokyo for the computational resources.
Conflicts of interest
All authors declared that there are no conflicts of interest.
Ethical approval and consent to participate
Not applicable.
Consent for publication
Not applicable.
Copyright
© The Author(s) 2025.
Supplementary Materials
REFERENCES
1. Zhao, Q.; Stalin, S.; Zhao, C.; Archer, L. A. Designing solid-state electrolytes for safe, energy-dense batteries. Nat. Rev. Mater. 2020, 5, 229-52.
2. Unemoto, A.; Matsuo, M.; Orimo, S. Complex hydrides for electrochemical energy storage. Adv. Funct. Mater. 2014, 24, 2267-79.
3. Higashi, S.; Miwa, K.; Aoki, M.; Takechi, K. A novel inorganic solid state ion conductor for rechargeable Mg batteries. Chem. Commun. 2014, 50, 1320-2.
4. Ruyet R, Berthelot R, Salager E, Florian P, Fleutot B, Janot R. Investigation of Mg(BH4)(NH2)-based composite materials with enhanced Mg2+ ionic conductivity. J. Phys. Chem. C. 2019, 123, 10756-63.
5. Le, R. R.; Fleutot, B.; Berthelot, R.; et al. Mg3(BH4)4(NH2)2 as inorganic solid electrolyte with high Mg2+ ionic conductivity. ACS. Appl. Energy. Mater. 2020, 3, 6093-7.
6. Roedern, E.; Kühnel, R. S.; Remhof, A.; Battaglia, C. Magnesium ethylenediamine borohydride as solid-state electrolyte for magnesium batteries. Sci. Rep. 2017, 7, 46189.
7. Burankova, T.; Roedern, E.; Maniadaki, A. E.; et al. Dynamics of the coordination complexes in a solid-state Mg electrolyte. J. Phys. Chem. Lett. 2018, 9, 6450-5.
8. Kisu, K.; Kim, S.; Inukai, M.; Oguchi, H.; Takagi, S.; Orimo, S. Magnesium borohydride ammonia borane as a magnesium ionic conductor. ACS. Appl. Energy. Mater. 2020, 3, 3174-9.
9. Yan, Y.; Dononelli, W.; Jørgensen, M.; et al. The mechanism of Mg2+ conduction in ammine magnesium borohydride promoted by a neutral molecule. Phys. Chem. Chem. Phys. 2020, 22, 9204-9.
10. Yan, Y.; Grinderslev, J. B.; Jo̷rgensen, M.; Skov, L. N.; Skibsted, J.; Jensen, T. R. Ammine magnesium borohydride nanocomposites for all-solid-state magnesium batteries. ACS. Appl. Energy. Mater. 2020, 3, 9264-70.
11. Yan, Y.; Grinderslev, J. B.; Burankova, T.; et al. Fast room-temperature Mg2+ conductivity in Mg(BH4)2·1.6NH3-Al2O3 nanocomposites. J. Phys. Chem. Lett. 2022, 13, 2211-6.
12. Wang, Q.; Li, H.; Zhang, R.; et al. Oxygen vacancies boosted fast Mg2+ migration in solids at room temperature. Energy. Storage. Mater. 2022, 51, 630-7.
13. Zhang, J.; Wang, W.; Chen, X.; Jin, J.; Yan, X.; Huang, J. Single-atom Ni supported on TiO2 for catalyzing hydrogen storage in MgH2. J. Am. Chem. Soc. 2024, 146, 10432-42.
14. Liu, F.; Cao, G.; Ban, J.; et al. Recent advances based on Mg anodes and their interfacial modulation in Mg batteries. J. Magnes. Alloys. 2022, 10, 2699-716.
15. Son, S. B.; Gao, T.; Harvey, S. P.; et al. An artificial interphase enables reversible magnesium chemistry in carbonate electrolytes. Nat. Chem. 2018, 10, 532-9.
16. Tang, K.; Du, A.; Dong, S.; et al. A stable solid electrolyte interphase for magnesium metal anode evolved from a bulky anion lithium salt. Adv. Mater. 2020, 32, e1904987.
17. Chinnadurai, D.; Lieu, W. Y.; Kumar, S.; Yang, G.; Li, Y.; Seh, Z. W. A passivation-free solid electrolyte interface regulated by magnesium bromide additive for highly reversible magnesium batteries. Nano. Lett. 2023, 23, 1564-72.
18. Chen, T.; Sai, G. G.; Canepa, P. Ionic transport in potential coating materials for Mg batteries. Chem. Mater. 2019, 31, 8087-99.
19. Hebié, S.; Ngo, H. P. K.; Leprêtre, J. C.; et al. Electrolyte based on easily synthesized, low cost triphenolate-borohydride salt for high performance Mg(TFSI)2-glyme rechargeable magnesium batteries. ACS. Appl. Mater. Interfaces. 2017, 9, 28377-85.
20. Wang, Q.; Li, Z.; Deng, H.; Chen, Y.; Yan, Y. Enhanced interface stability of ammine magnesium borohydride by in situ decoration of MgBr2·2NH3 nanoparticles. Chem. Commun. 2023, 59, 6726-9.
21. Xu, X.; Chao, D.; Chen, B.; et al. Revealing the magnesium-storage mechanism in mesoporous bismuth via spectroscopy and Ab-initio simulations. Angew. Chem. Int. Ed. Engl. 2020, 59, 21728-35.
22. Chen, X.; Wei, S.; Tong, F.; Taylor, M. P.; Cao, P. Electrochemical performance of Mg-Sn alloy anodes for magnesium rechargeable battery. Electrochimica. Acta. 2021, 398, 139336.
23. Ikhe, A. B.; Han, S. C.; Prabakar, S. J. R.; Park, W. B.; Sohn, K.; Pyo, M. 3Mg/Mg2 Sn anodes with unprecedented electrochemical performance towards viable magnesium-ion batteries. J. Mater. Chem. A. 2020, 8, 14277-86.
24. Wang, L.; Welborn, S. S.; Kumar, H.; et al. High-rate and long cycle-life alloy-type magnesium-ion battery anode enabled through (de) magnesiation-induced near-room-temperature solid-liquid phase transformation. Adv. Energy. Mater. 2019, 9, 1902086.
25. Chai, X.; Xie, H.; Zhang, T.; et al. Ternary Mg alloy-based artificial interphase enables high-performance rechargeable magnesium batteries. Energy. Storage. Mater. 2024, 70, 103460.
26. Singh, N.; Arthur, T. S.; Ling, C.; Matsui, M.; Mizuno, F. A high energy-density tin anode for rechargeable magnesium-ion batteries. Chem. Commun. 2013, 49, 149-51.
27. Kravchyk, K. V.; Piveteau, L.; Caputo, R.; et al. Colloidal bismuth nanocrystals as a model anode material for rechargeable Mg-ion batteries: atomistic and mesoscale insights. ACS. Nano. 2018, 12, 8297-307.
28. Jung, S. C.; Han, Y. Fast magnesium ion transport in the Bi/Mg3Bi2 two-phase electrode. J. Phys. Chem. C. 2018, 122, 17643-9.
29. Yaghoobnejad Asl, H.; Fu, J.; Kumar, H.; Welborn, S. S.; Shenoy, V. B.; Detsi, E. In situ dealloying of bulk Mg2Sn in Mg-ion half cell as an effective route to nanostructured Sn for high performance Mg-ion battery anodes. Chem. Mater. , 30, 1815-24.
30. Kresse, G.; Furthmüller, J. Efficient iterative schemes for ab initio total-energy calculations using a plane-wave basis set. Phys. Rev. B. 1996, 54, 11169-86.
31. Perdew, J. P.; Burke, K.; Ernzerhof, M. Generalized gradient approximation made simple. Phys. Rev. Lett. 1996, 77, 3865-8.
32. Kresse, G.; Joubert, D. From ultrasoft pseudopotentials to the projector augmented-wave method. Phys. Rev. B. 1999, 59, 1758-75.
33. Monkhorst, H. J.; Pack, J. D. Special points for brillouin-zone integrations. Phys. Rev. B. 1976, 13, 5188-92.
34. Henkelman, G.; Uberuaga, B. P.; Jónsson, H. A climbing image nudged elastic band method for finding saddle points and minimum energy paths. J. Chem. Phys. 2000, 113, 9901-4.
35. Nayeb-hashemi, A. A.; Clark, J. B. The Bi-Mg (Bismuth-Magnesium) system. Bull. Alloy. Phase. Diagrams. 1985, 6, 528-33.
36. Pradhan, B.; Dalui, A.; Paul, S.; Roy, D.; Acharya, S. Solution phase synthesis of large-area ultra-thin two dimensional layered Bi2Se3: role of Cu-intercalation and substitution. Mater. Res. Express. 2019, 6, 124005.
37. Xu, X.; Ye, C.; Chao, D.; et al. Synchrotron X-ray spectroscopic investigations of in-situ-formed alloy anodes for magnesium batteries. Adv. Mater. 2022, 34, e2108688.
38. Li, Y.; Yang, G.; Zhang, C.; et al. Grain-boundary-rich triphasic artificial hybrid interphase toward practical magnesium metal anodes. Adv. Funct. Mater. 2023, 33, 2210639.
39. Ding, M. S.; Diemant, T.; Behm, R. J.; Passerini, S.; Giffin, G. A. Dendrite growth in Mg metal cells containing Mg(TFSI)2/glyme electrolytes. J. Electrochem. Soc. 2018, 165, A1983-90.
40. Yoo, H. D.; Han, S. D.; Bolotin, I. L.; et al. Degradation mechanisms of magnesium metal anodes in electrolytes based on (CF3SO2)2N- at high current densities. Langmuir 2017, 33, 9398-406.
41. Dewitt, S.; Hahn, N.; Zavadil, K.; Thornton, K. Computational examination of orientation-dependent morphological evolution during the electrodeposition and electrodissolution of magnesium. J. Electrochem. Soc. 2016, 163, A513-21.
42. Zhang, Y.; Li, J.; Zhao, W.; et al. Defect-free metal-organic framework membrane for precise ion/solvent separation toward highly stable magnesium metal anode. Adv. Mater. 2022, 34, e2108114.
Cite This Article
How to Cite
Wang, Q.; Li, H.; Xu, T.; Chen, Y.; Yan, Y. Understanding Mg-ion deposition behavior on MgBi alloy in solid-state form. Energy Mater. 2025, 5, 500022. http://dx.doi.org/10.20517/energymater.2024.102
Download Citation
Export Citation File:
Type of Import
Tips on Downloading Citation
Citation Manager File Format
Type of Import
Direct Import: When the Direct Import option is selected (the default state), a dialogue box will give you the option to Save or Open the downloaded citation data. Choosing Open will either launch your citation manager or give you a choice of applications with which to use the metadata. The Save option saves the file locally for later use.
Indirect Import: When the Indirect Import option is selected, the metadata is displayed and may be copied and pasted as needed.
About This Article
Copyright
Data & Comments
Data
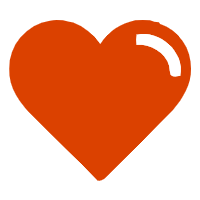
Comments
Comments must be written in English. Spam, offensive content, impersonation, and private information will not be permitted. If any comment is reported and identified as inappropriate content by OAE staff, the comment will be removed without notice. If you have any queries or need any help, please contact us at support@oaepublish.com.