Recent advances in alloying anode materials for sodium-ion batteries: material design and prospects
Abstract
Sodium-ion batteries (SIBs) are close to commercialization. Although alloying anodes have potential use in next-generation SIB anodes, their limitations of low capacities and colossal volume expansions must be resolved. Traditional approaches involving structural and compositional tunings have not been able to break these lofty barriers. This review is devoted to recent progress in research on alloy-based SIB anodes comprising Sn, Sb, P, Ge, and Si. The current level of understanding, challenges, modifications, optimizations employed up to date, and shortfalls faced by alloying anodes are also described. A detailed future outlook is proposed, focusing on advanced nanomaterial tailoring methods and component modifications in SIB fabrication. Utilizing the latest state-of-the-art characterization techniques, including ex-situ and operando characterization tools, can help us better understand the (de)sodiation mechanism and accompanying capacity fading pathways to pave the way for next-generation SIBs with alloying anode materials.
Keywords
INTRODUCTION
Sodium-ion batteries (SIBs) have recently drawn attention as current lithium reservoirs are depleting which poses severe supply issues. This situation is further complicated due to polarization of the world's primary energy economies[1,2]. Although energy density supplied by current generation lithium-ion batteries (LIBs) is fascinating, many other competitors have joined this race and gained subtle success at lower costs and higher safety, including SIBs[3], lithium-sulfur[4], Zinc-ion[5], alkaline metal[6], solid-state[7], metal air[8], redox flow batteries[9], and supercapacitors[10]. Each of these systems offers some advantages in terms of capacity, energy and power density, benignity, safety, and longevity. At the same time, each has certain limitations that need to be fixed soon to delimit their potential[11,12]. Although battery systems with metallic anodes and sulfur cathodes offer ideal performances, they suffer from serious issues presently[13-15]. SIBs share the same components and design assembly as LIBs but with different materials and kinetics. However, the larger ionic radius and molar mass of Na+ than Li+ pose more restrictions whereby the higher redox potential of Na results in lower energy density and theoretical capacity (1,166 mA g-1 for Na and 3,861 mAh g-1 for Li)[16,17]. From a cost perspective, lithium mineral ore price is highly fluctuating, with a 2023 price of around 7,000 Euro/Ton. Its price is expected to increase, although the energy per ton price is speculated to remain stagnant. However, it remains difficult to predict its price due to highly fluctuating geopolitical situations in the world[18-20]. Nevertheless, the high abundance of sodium with uniform distribution across the globe presents much cheaper alternatives to LIBs. In addition, cell assembly for SIBs uses cheaper current collectors, i.e., Al foil, which is not compatible with LIBs anode due to Li-Al alloy formation[21,22]. The compatibility of highly ionic conductive and safe electrolyte additives in SIBs has also opened future gateways for high-rate and wide temperature-sustaining batteries, particularly for grid-scale applications.
SIB anodes have potential to be explored for high capacity with several advantages along with a diverse selection of materials offering multiple mechanisms and optimization possibilities. Therefore, they have opened a wide research window for optimized SIB performance. Notably, graphite, a recognized commercial LIB anode, has a mismatched size for Na+ shutting. The low capacity and unstable performance behavior of current SIB anodes have impeded their commercialization[3,23]. SIB anodes can be categorized into three basic types based on the prevailing Na+ storage mechanism: intercalation, alloying, and conversion-type[24].
Although research on SIBs was initially started with LIBs in the 1990s, the much higher performance of LIBs than SIBs has left little admiration for the advancement of SIBs[25]. Recent improvements in SIB capacity have been bestowed by nanoengineering and adopting advanced tools for material performance with in-situ and postmortem analysis of SIB cells. These adaptabilities have let a paradigm focus on SIB advancements for next-generation batteries. Appreciably, many emerging cathodes, such as NaCoO2, NaMnO2,
There is no doubt that carbon materials have become the foremost choices as SIB anode materials. The incompatibility of the interlayer spacing of graphite for intercalation of large-sized Na+ has given room for expanded graphitic carbon and hard carbon for opting as SIB anode materials. Interestingly, they are proven to be viable as SIB anode materials. In fact, hard carbon from various sources, including biomass-derived hard carbon, has been widely searched for an optimum capacity for delivering SIB anodes[3,31-37]. The high porosity of carbonaceous materials helps sustain a high capacity by introducing more Na+ in pores[34,38,39].
Although diverse efforts have been devoted to the incorporation of defects and functionalities in carbonaceous materials for improved performances of SIB anodes as their effects on capacity and overall performance enhancement are evident in many instances, their participation in capacity-fading pathways hinders their benefits[40,41]. For example, the trapping and reaction of Na+ with defects in functionalities often lead to capacity fading, particularly in initial cycles, contributing to trap effect and some irreversible capacity loss over long cycling. Still, their role in capacity fading has not yet been unveiled[42-44]. Although there are diverse approaches of doping in carbon to generate heterogenous surfaces and compositing these carbonaceous materials to alloying anode materials that bear both intrinsic and extrinsic defects, their participation in side reactions demands close monitoring to have an optimized performance. Also, in some instances, these defects in-situ generated during (dis)charging cycles remain undetected due to non-utilization of conclusive mechanistic approaches[3,17,45]. Although many modifications have been made for carbonaceous anodes, none has fully satisfied the standard of a commercial SIB anode[34,46-49]. This has led to a recent shift towards alloying and conversion/alloying anode materials that show more promise for high-energy-density SIBs.
While conversion-type anodes, including transition metal compounds such as oxides[50], sulfides[51], selenides[52], tellurides[53], phosphides[54], and so on, have been widely focused, they have not achieved performance targets. Amongst all, alloying-type materials that mainly include Sn, Sb, Si, Ge, and P are the most explored SIB anode materials[24,48]. Although these anode materials have high theoretical sodiation capacity, the alloying reaction (xNa+ + xe- + M
Various parameters (volume expansion, average voltage, and theoretical capacity) for sodiation reactions of alloy-based materials
Metal | Alloyed compositions | Volume expansion (%) | Average voltage (vs. Na/Na+) (V) | Theoretical capacity (mAh g-1) |
Si | NaSi/Na0.75Si | 114 | ~0.50 | 954/725 |
Sn | Na15Sn4 | 420 | ~0.20 | 847 |
Ge | NaGe | 205 | ~0.30 | 576 |
Sb | Na3Sb | 390 | ~0.60 | 660 |
Bi | Na3Bi | 250 | ~0.55 | 385 |
P | Na3P | > 300 | ~0.40 | 2,596 |
Although breakthrough has not been achieved yet, their extreme performance potentials have been extensively focused on as they can deliver very high and stable capacities[55]. Many developments have recently been made to improve capacities of alloying SIBs, including electrode materials, morphologies, electrolytes, and binder modulations[17,29,56-59]. Although hundreds of research papers have been added to the literature on alloying SIB materials in the past few years, unfortunately, no recent review has solely covered recent progress on alloying SIB anodes. Therefore, a comprehensive overview of research developments, particularly during the last six years, which are uniquely devoted to alloying anodes, namely Sn, Sb, P, Ge, and Si, is needed.
This review paper addresses alloy materials in five main sections: (1) Alloy-based Anodes for Sodium-Ion Batteries; (2) Materials Design Strategies; (3) Challenges Associated with Sodium-Ion Batteries; (4) Optimizations of Sodium-Ion Batteries; and (5) Summary and Future Prospects. In detail, the current review focuses on recent trends in material design strategies employed to have efficient SIB alloying anodes with details of unresolved challenges, such as initial huge capacity fading accompanied by multifold volume variations. Lastly, various modifications adapted to cope with challenges faced by alloying SIB anodes are briefly detailed, followed by concrete recommendations for future research. In this regard, using advanced characterization tools to unveil the mechanism through spatial and temporal tracing of species evolved during the de(alloying) process is highly encouraged to sustain alloying SIB materials.
ALLOY-BASED ANODES FOR SIBS
Tin-based anodes for siBs
Tin (Sn) is an incredible choice as an anode material in SIBs because of its high theoretical capacity
Broadening the temperature window for commercial batteries has been much desired for advanced electronic applications with more safety. For instance, Yang et al. have presented their tin-based anode with a nanorod morphology incorporated with layers of nitrogen-doped carbon to cope with challenges faced by commonly exploited SIB anodes[75]. Effective surface tuning of this anode enabled it to deliver a good capacity for 10 k cycles. When this anode was employed in full cell (taking NVP as the cathode), the cell delivered an energy density of 215 Wh Kg-1 over a broad temperature range of -20 to 50 °C. The floret-like 3D morphology with improved Na+ diffusion kinetics and high sodium affinity has dually been validated by density functional theory (DFT) calculations. Moreover, their findings also support the formation of a stable hybrid SEI of diethylene glycol dimethyl ether (DEGDME) with inorganic fluoride-rich ionic conductive polyether electrolyte to achieve a capacity of 347 mAh g-1 at 2 A g-1 retained for 10,000 cycles. Such marvelous performance has left behind many other strategies and opened a new door for exploring new electrolyte formulations. The flair of DEGDME for better compatibility with NaPF6 has been proven by DFT calculations that show a higher energy difference for electron promotion from the highest occupied molecular orbital (HOMO) to the lowest unoccupied molecular orbital (LUMO) than in commonly employed carbonate-based electrolytes.
Tin-based oxides
Tin oxide is a promising class of anodic materials for SIBs owing to its intriguing features such as abundance, low toxicity, and high theoretical capacity. Tetragonal tin dioxide (SnO2) and orthorhombic tin monoxide (SnO) are two main types of tin-based oxides. SnO has a layered structure with a Sn-O-Sn sequence. Its large spacing between layers allows easy insertion and extraction of Na+. The reaction mechanism of conversion and alloying is given as follows:
Conversion reactions:
Alloying reaction:
Due to their dual conversion and alloying reactions with Na+, high theoretical specific capacities of 1,375 and 1,150 mAh g-¹ are achieved for SnO2 and SnO, respectively. However, the low reversibility of the conversion reaction can lead to rapid capacity declination during initial cycles that additionally suffer from large volume change and the low electrical conductivity of tin-based oxides hamper their practical applications. One effective strategy is by forming a hybrid, often involving designing a structure with nanosized tin oxides and combining it with nanostructures such as nanofibers (NFs), nanosheets, nan-flowers, yolk shells, and so on[24]. Zhang et al. have prepared a flexible fibrous composite of CNFs decorated with N-doped carbon nanotubes (CNTs) and SnO2 nanoparticles as bare nanostructured SnO2 is prone to fracture and detachment from the conductive matrix, which loses electronic contact during an electrochemical reaction[76]. Processed anodes can deliver a highly stable cycling performance and impressive rate capabilities for SIBs. The optimized composite showed a discharge capacity of 460 mAh g-1 after 200 cycles and 222.2 mAh g-1 at a high current density of 3.2 A g-1. The continuous fibrous structure gave additional stability to the anode, thus retaining its fibrous morphology during sodiation/desodiation.
Oxygen vacancy creation in active nanostructures often can improve capacity and charge transfer kinetics. It has been observed that in SnO2, these vacancies could enhance Na+ storage capacity and increase electrical conductivity. Oxygen vacancies can also cope well with volume changes in the process of (de)sodiation, improve the cyclic stability of SnO2 anodes, and extend the lifespan of batteries. Ma et al. have utilized these oxygen vacancies effectively to present oxygen vacancy-bearing SnO2-x and combine it with porous CNFs (PCNF) to construct a homogeneously confined nanoparticles (SnO2-x/C) composite[77]. The prepared composite was directly used as an anode without a binder or other conductive additives for SIBs. It displayed superb electrochemical properties including high reversible capacity, sustaining rate capability, and ultra-long cyclic stability even after thousands of cycles. The discharge capacity of 565 mAh g-1 over 2,000 cycles at 1A g-1 was attained, whereas the bare SnO2 showed a meager capacity retention of 57 mAh g-1 after 800 cycles. Heterostructure methodology along with C compositing is a promising approach to enhancing the cyclic capacity of electrode materials in SIBs. One such approach has been adopted by
A unique composite structure of 1D ultrafine SnO2 nanorods and 3D graphene aerogel (SnO2NRs/GA) has been fabricated by a reduction-induced self-assembly method. Vitamin C was used to facilitate the reduction of graphene oxide (GO). The combination of 3D aerogel and 1D SnO2 nanorods resulted in a synergistic effect that improved electrochemical performance of the material[79]. As an SIB anode, the material delivered an initial discharge capacity of 232 mAh g-1 at 0.02 A g-1 in 100 cycles. The composite (SnO2 NRs/GA) also demonstrated excellent cycling stability as an SIB anode, with a high reversible capacity of 96 mAh g-1 at a high current density of 1 A g-1 for 500 cycles. Demir et al. have demonstrated that an
An amorphous tin oxide (a-SnOx) with a nano-helical structure containing extended defects has been prepared via a solution and surfactant-free oblique angle deposition method (as shown in
Figure 1. (A) Schematic illustration of the uniquely designed amorphous tin oxide using oblique angle deposition methodology, (B) SEM image, (C) cyclic performance, and (D) rate performance of the synthesized amorphous tin oxide nano-helices. Reproduced with permission from[81]. Copyright © 2019 American Chemical Society.
Han et al. have reported an intriguing structure wherein ultrafine SnO2 nanoparticles are encapsulated into the inner space of holey CNTs by taking advantage of the melt infiltration method[82]. Holey CNTs provided a conductive network and ample void spaces for sodium-ion transportation useful for accommodating volume variations during the charging/discharging process. As an SIB anode, the material delivered a reversible discharge capacity of 184 mAh g-1 at a discharge current density of 1,000 mAh g-1 over 200 cycles. Narsimulu et al. have used a one-step solvothermal method to prepare a freestanding, flexible, and binderless 3D porous nanocomposite of SnO2 onto a conductive carbon cloth[83]. The distinctive morphology [Figure 2A] of the 3D composite demonstrated good reversibility and excellent rate performance when employed as an anode for SIBs [Figure 2B]. The 3D hollow fiber structure and spaces between the SnO2-NPs can accommodate undesirable volume changes during the charging/discharging process. The structural integrity is maintained due to bonding between SnO2 and carbon cloth. Moreover, the excellent conductivity and ultra-small nature of SnO2 nanoparticles enable quick ion electron diffusion passages. The composite displayed a high sodium storage capacity of 498 mAh g-1 at a current density of
Figure 2. (A) Synthetic representation of solvothermal fabrication of SnO2-NPs on carbon cloth. (B) Cycling and rate performance for SIBs. (C) Flexibility testing of the material at different angles (i-iv). Reproduced with permission from[83]. Copyright © 2021 Elsevier.
Tin-based sulfides
Many tin sulfides have been sorted as potential SIB anode materials due to their fascinating features, including high capacity, good electrical conductivity, mechanical endurance, and cost-effectiveness. The unique layered structure of tin sulfides, such as hexagonal SnS2 and orthorhombic SnS, offers more Na+ intake by conversion-alloying reactions:
The alloying reaction follows this conversion reaction:
Although the weak M-S bond endorses better reaction reversibility with theoretical capacity values of SnS2 and SnS being 1,137 and 1,022 mAh g-1, respectively, the large volume alteration during (de)sodiation in
More economical electrode materials can be derived from various bio-waste utilizations that can positively influence the environment and add structural benefits. In this regard, He et al. have prepared an algal waste-derived anode SnS2/EPC (enteromorpha prolifera derived carbon) that can deliver a high capacity of
Controlled interlayer distances in a material can be constructed with another strategy that can ensure the provision of suitable ion transfer pathways. In this regard, a uniquely designed material, SnS2/reduced GO (rGO), with extended interlayer spacing has recently been presented by Jiang et al.[88]. They introduced polyethylene glycol (PEG) as an intercalant. The PEG-SnS2/rGO composite had a conductive graphene channel that ensured high conductivity and an efficient charge transfer process. C-S covalent bonds also strongly cohered C-S covalent bonds between the graphitic skeleton and SnS2, which enabled their structural integrity during (de)sodiation. After 100 cycles at 0.1 A g-1, a capacity of 770 mAh g-1 was attained with an equally competing rate performance capacity of 720 mAh g-1 at 2 A g-1. A 3D 1T-SnS2 structure wrapped with graphene (1T-SnS2/rGO) has been synthesized onto Ni foam by chemical vapor deposition (CVD) and spray coating[89]. The unique compositing with 1T phase and rGO coating was chosen as an SIB anode. It showed initial charge and discharge capacities of 748.7 and 768.8 mAh g-1, respectively [coulombic efficiency (CE) = 97.4%], along with a capacity retention of 84.6% after 100 cycles.
Similar to graphene, SnS and SnS2 from n- and p-type semiconductors have a unique layered structure. The interface between the two materials develops a p-n junction when they are joined to produce a heterostructure, Sn-SnS2. This p-n junction generates an electric field that can facilitate the electron transfer across the material. A monolithic composite SnS-SnS2@GO was constructed by a single-step solvothermal method[90]. Multilayered SnS-SnS2@GO heterostructured nanosheets exhibited high capacity and stability as an anode for SIBs. After 100 cycles, the capacity sustained by the anode was 450.6 mAh g-1 (CE = 69.8%). The capacity sustainability in the composite was commended by the presence of GO, which could alleviate volume expansion effects of the intrinsic SnS-SnS2 material to a certain degree and give excellent cyclic stability.
Yang et al. have recently proposed a ZnS/SnS2 hybrid with N-doped C-fiber encapsulating the ZnS/SnS2-like beads on the thread, as shown in Figure 3A[91]. The material showed excellent structural and capacity retention, as shown in Figure 3B. In the SIB anode, the material retained a capacity of 174.5 mAh g-1 after 1,000 cycles (CE 62%). Its rate performance showed a capacity suspension of ~312 mAh g-1 at 2 A g-1, while a capacity of 601.1 mAh g-1 was restored after reducing the current to 0.1 A g-1. Huang et al. have presented an optimized SIB anode composed of SnO2@SnS2 heterostructured QDs (HQDs) evenly embedded on
Figure 3. (A) (a) Crystal structure of individual ZnS and SnS2. (b) XRD pattern of ZnS/SnS2@NCNFs. (c-n) Electron microscopic details with elemental mapping of ZnS/SnS2@NCNFs. (B) Electrochemical performance characteristics of ZnS/SnS2@NCNFs for SIBs. Reproduced with permission from[91]. Copyright © 2023 American Chemical Society.
A heterostructured SnS2/Mn2SnS4/C hybrid has been developed using a simple methodology [Figure 4A], showing a high SIB anode capacity[93]. A prominent capacity (841.2 mAh g-1) with a high ICE of 90.8% was achieved. After an extended period of cycling 500 times, the composite presented a capacity of
Figure 4. (A) (a) Schematic illustration of SMS/C composite and (b-d) SEM images. (B) (a) Contour plots and (b) in-situ XRD stack pattern during cycling. (C) (a) In-situ TEM and (b) in-situ SAED pattern of fabricated nano-cell during initial cycle. Reproduced with permission from[93]. Copyright © 2019 American Chemical Society.
Tin-based selenides
SnSe and SnSe2 are among 2D transition metal chalcogenides with orthorhombic and hexagonal layered structures, respectively. Due to their wide interlayer spacing, tin selenides (SnSe2) have been considered as promising materials for storing more Na+. They can also absorb significant volume changes. Theoretical capacities of SnSe2 and SnSe are about 756 and 778 mAh g-1, respectively. Although they show electrochemical behavior similar to Sn-based sulfides and oxides, the bond between Sn and Se is relatively weaker which supports faster Na+ kinetics. However, these selenides alone have compromised Na+ storage in most cases due to their low tolerance of volume expansion/contraction. Thus, Tin selenides have mostly been tested in a composite or hybrid form[24]. Zhang et al. have prepared a SnSe2@C nanocomposite, which shows high sodium storage when evaluated for SIB anode applications[94]. For the synthesis of this nanocomposite, carbonization of Sn-based metal-organic framework (MOF) and subsequent selenylation were carried out, which resulted in unique C-Sn bonds anchoring SnSe2 nanoparticles encapsulated in carbon nanoshells. The SnSe2@C nanocomposite delivered an admired stability and a superior rate capability of 324 mAh g-1 at a current density of 2 A g-1 for SIB.
Recently, the tin selenide (SnSe) nanosheet array on carbon cloth has been explored for a binder and current collector-free anode assembly that could pump high Na+, showing an initial capacity of 713 mAh g-1 at 0.1 C, with capacity sustained at 410 mAh g-1 after 50 cycles and 161 mAh g-1 after 250 cycles[95]. Operando XRD studies revealed that it improved phase reversibility compared to a SnSe bulky particle-derived anode. The capacity fading issue was due to the unviable design architecture of carbon cloth that needed further improvisation. Liu et al. have engineered a hierarchical nanobox-designed material using multistep synthesis, as shown in Figure 5A(a-d). The unique structuring strategy comprised a polydopamine (PDA) shell with a heterojunction bimetallic yolk of SnSe2/ZnSe[96]. The SnSe2/ZnSe@PDA nanobox bearing bimetallic heterojunctions created lattice distortions and long-range disorders that not only improved thermodynamic stability of the material, but also affected electronic distributions at heterojunctions that could support Na+ mobility at heterojunctions. Hollow structures can suppress volume changes and increase the contact area between the electrode and electrolyte. The conductive and elastic PDA shell functioned as a buffer layer to guard against aggregation and disintegration of the SnSe2/ZnSe yolk during cycling. The heterostructure nanobox SnSe2/ZnSe@PDA displayed a capacity of mAh g-1 at a current density of
Figure 5. (A) (a) Strategic illustration of the synthesis of SnSe2/ZnSe@PDA nanobox. (b-d) SEM images with size distribution of nanoboxes. (e) Schematic illustration and TEM showing structural changes in the composite during (dis)charging. (B) Electrochemical Na+ storage in SIBs: (a) charge/discharge, (b) cycling performance, (c) rate capability, and (d) Ragone plots of composite
The combined layering effect of SnSe2 and Ti3C2Tx (MXene) has been explored in layered composite
Wang et al. have designed a novel composite (MoSe2/SnSe2@C) showing promise as an SIB anode[98]. The composite had a 2D van der Waals heterostructure representing a loose connectivity of the two distinct layers in the material. At the phase interface, this structure enabled lattice deformation and electron redistribution, accelerating ion migration and charge transfer while the porous carbon increased its electronic conductivity. As a consequence, the MoSe2/SnSe2@C composite showed a good cycling performance for SIBs with a reversible capacity of 591.4 mAh g-1 over 110 cycles at 0.1 A g-1 and a capacity of 334 mAh g-1 after 200 cycles at 0.5 A g-1. Ex-situ high-resolution TEM (HRTEM) elucidated the sequential conversion of the material upon (de)intercalation with MoSe2, showing higher reversible transformation than the SnSe2 phase.
Tin-based phosphides
Sn can also form alloys with P that could reversibly intercalate Na+ in the assembly of anode for SIBs. Among various phosphides, the most common one is Sn4P3 with a rhombohedral crystal structure that has garnered more interest due to its superior electrical conductance, higher gravimetric capacity
Figure 6. (A) TEM imaging studies showing (a) SnO2 microspheres, (b) SnO2/SiO2 microspheres, (c) hollow microspheres of SnO2@C, and (d) hollow microspheres of Sn4P3@C. (e-h) Elemental mapping images of Sn4P3@C, (i) HRTEM image of Sn4P3 with (j) SAED pattern of Sn4P3@C. (B) (a) Discharge/charge profiles of hollow Sn4P3@C electrodes at different current densities, (b) Comparison of cyclic performance of hollow Sn4P3@C with pure Sn4P3 electrodes at a current density of 0.2 A g-1, and (c) Extended cycling of Sn4P3@C hollow microsphere anode at high ampere densities of 2 and 5 A g-1, respectively. Reproduced with permission from[103]. Copyright © 2019 American Chemical Society.
Similarly, the µ-sized Sn4P3 delivered an extremely high reversible capacity of 960.3 mAh g-1 at 100 mA g-1 with an ICE of 89.8% after 100 cycles in a diglyme (DGM)-based electrolyte. Also, a capacity retention of 75.1% was recorded after 100 cycles. Such excellent Na storage performance was due to the flexible, compact, and uniform SEI layer in the ether-based electrolyte, which successfully inhibited the separation and aggregation of active components and provided favorable kinetics[104]. A fascinating biomimetic heterostructured Sn4P3 grown on CNT (Sn4P3@CNT/C) has been developed by a hydrothermal reaction. This biomimetic bottle brush was designed as a structure in which CNTs served as a “stem” to provide an electron-transferring superhighway and mechanical stability. To enhance the contact area of the CNT surface with the electrolyte along with shortened ion diffusion channels, Sn4P3 nanoscale assemblies functioned as a “fructus”. Furthermore, stresses generated during (de)sodiation were recurrently buffered. The Sn4P3@CNT/C hybrid anode exhibited an outstanding electrochemical performance with a steadily high capacity of 742 mAh g-1 after 150 cycles at 0.2 C, together with 449 mAh g-1 at 2 C after 500 cycles. The following conversion-alloying reactions occurred:
Initially sodiated states formed included the irreversible formation of Na15Sn4 and Na3P in the first cathodic scan (at 0.03 V). The anodic scan showed two consistent peaks at 0.55 and 0.68 V that corroborated the desodiation of Na15Sn4 and Na3P to form Sn and P, respectively. This evidence was further proved by ex-situ XRD and TEM[105]. An interesting multiphase SnxPy/rGO nanohybrid with pronounced Na+ storage characteristics has been reported. The multiphasic structure with Sn in the form of Sn4P3 and SnP0.94 was protected by graphene, which, together with the multiphase Sn structure, enabled high volume shuttering along with an excellent structural reversibility as evidenced by ex-situ XRD, SEM, and HRTEM studies where both Sn4P3 and SnP0.94 were detected in disassembled electrodes. The SnxPy/rGO electrode afforded an improved capacitive-dominated Na+ storage capacity of 421.8 mAh g-1 over 100 (dis)charge cycles at a current density of 500 mA g-1, resulting in a capacity retention of 84.7%. Additionally, a capacity of about 200 mAh g-1 was conserved over 200 cycles at 2.0 A g-1, which was superior to many other phosphide SIB anodes with unimpressive capacity retention at high current rates[101].
Fan et al. have recently fabricated template-assisted growth of Sn4P3 hollow nanospheres (HS) dually protected by multifunctional conductive MXene sheeted shells[106]. The highly controlled methodology ensured optimum morphological benefits for Na+ transport assisted by MXene encapsulation, as shown in Figure 7A. The role of conductive shell extends to maintaining the homogeneous ionic flux on the MXenes surface, which upon interaction with the electrolyte ensures a highly thin and stable SEI. The SEI composition in the cycled cells was traced using ex-situ X-ray photoelectron spectroscopy (XPS). In addition to other electrolyte decomposition products, species contributing to SEI stabilization and structural stability that ensured an ICE of about 84% along with a capacity of 390.5 mAh g-1 at 1 A g-1 after 500 cycles in the full cell taking the Sn4P3 HS@MXene anode coupled with the commonly used SIB cathode, NVP, were traced, as demonstrated in Figure 7B.
Figure 7. (A) (a) Schematic representation for synthesis of Sn4P3 HS@MXene nanocomposite. SEM images of (b) Sn4P3 HS and
Fan et al. have reported a method of transforming 2D MXene to the highly conductive 3D conductive network by sandwiching Sn and Sn4P3 nanoparticles between MXene sheets[107]. Due to covalent interaction with Sn4P3 nanoparticles, the uniformly distributed ultra-small Sn nanoparticles (≈ 4 nm) contributed to conductive frameworks both horizontally and vertically to MXene planes. Additionally, metallic Sn nanoparticles imparted further to the conductivity of the composite. This hybrid combination of conductive matrices synchronously activated tin phosphide electrochemically, leading to enhanced specific capacity. Volume changes of Sn and tin phosphides were coped by Sn/MXene during discharging/recharging cycles to enable the anode to deliver an ultra-stable cycle life with a capacity of 143.1 mAh g-1 over 1,000 cycles at
Antimony-based anodes for SIBs
Antimony (Sb) has been ranked as one of the most promising SIB anodes with desirous properties, including high conductive character (electrical conductivity about 2.56 × 106 Sm-1), suitable theoretical capacity (660 mAh g-1 for Na3Sb alloy), and low operational potential (about 0.5 V). However, as with other alloying anodes for SIBs, Sb also has many shortcomings that have plagued its utility on a commercial scale. Primarily, complex amorphous structural evolutions having different alloying capabilities with Na+ and finally reaching full sodiation in the crystalline structure of Na3Sb are still not well understood yet as intermediate states are difficult to be probed using XRD and other common techniques. CV charge/discharge curves of Sb have revealed a two-step sodiation process.
However, detailed Mossbauer and solid-state nuclear magnetic resonance (NMR)-derived spectroscopic evidence has proposed a multistep alloying with amorphous sodium depleted intermediates not in good agreement with various studies[30,108]. Huge volume variations (390%) upon full sodiation have also impeded the utility of Sb anodes. Among common routes of modifications, nanostructuring, intermetallic alloy formations, and hybrid composite structural optimizations have been commonly chosen. Thin-walled, heteroatom-doped 1D Sb nanotubes (NTs) reported by Liu et al. have shown formidable performance as an SIB anode showing long cyclability with 342 mAh g-1 capacity retaining ability at a current density of 1 A g-1 over 6,000 cycles and a rate performance of 286 mAh g-1 at a current density of 10 A g-1[109]. Manifestation of a high operational voltage (2.7 V) and a good energy density (252 Wh kg-1) has also been demonstrated in a full cell configuration when coupled with Na3(VOPO4)2F as a cathode.
Several Sb composites have been presented to improve Na+ storage performances of SIB anodes with more resilience for volume variations. In this regard, again, the favored choice was the utilization of C-based heterogeneous matrices. Qian et al. have previously proposed Sb/C SIB anodes showing a capacity of
It has been reported that 0D Sb nanodots interconnected with 2D C nanosheet composite (Sb-NDs/CN) have an optimum 3D network structure that provides a high surface area, short ion and electronic diffusion paths, and well-dispersed Sb nanodots that could prevent their agglomeration and afford volume buffering[112]. As an SIB anode, the composite showed competing rate performance (271 mAh g-1 at a current of 2 A g-1) and good cyclic stability of about 380 mAh g-1 at a current density of 0.3 A g-1. A thin filmed, porous self-supported Sb-C framework has been proposed previously. It sustained a capacity of 306 mAh g-1 over 5,000 cycles at 2.34 A g-1 and supplemented with a high rate performance of 200 mAh g-1 at a high current rate of 7 A g-1[113]. The superb performance was due to thin patterning with well-dispersed Sb nanodots (~3 nm size) ensured by a conductive C matrix for efficient Na+ and e- transfer kinetics that ensured amorphous/crystalline phase reversibility.
Recently, we have tested an MOF-derived mesoporous carbon composite of Sb along with SiOC as an SIB anode. It showed stable performance. Upon sodiation, it showed multiple plateaus corresponding to the stepwise Sb transformation to Na3Sb that mainly contributed to the capacity[114]. After 200 cycles, a capacity of 403.81 mAh g-1 was traced with 100% CE, while a rate performance of 366.83 mAh g-1 at 5 A g-1 was observed. Cycled electrodes were analyzed by SEM. They showed macro cracks after 200 cycles. SiOC layers effectively buffered volume changes of Sb during sodiation-desodiation cycles. Liu et al. have recently validated yolk-void-shell assembled antimony-graphdiyne (Sb@Void@GDY) nanocuboid structures for classical performance of an SIB anode[115]. They bear definite voids to nullify the volumetric expansion/contraction of Sb during charge/discharge processes [Figure 8]. The unique synthetic strategy illustrated in Figure 8A was validated by XPS and TEM studies [Figure 8B]. Although the ICE was deliberately low (45.6%), the electrode showed more committed performance afterward and offered a capacity of
Figure 8. (A) Schematic diagram for the synthesis of Sb@Void@GDY nanoboxes. (B) (a) Raman spectrum and (b) XPS spectrum of
Various other modifications such as the use of binders and additives have been made for Sb anodes to optimize their performances. For instance, the role of solution additives during the antimony electrodeposition synthesis has been validated recently by Nieto et al.[116]. They utilized surfactants such as SDS (bis(3-sulfopropyl) disulfide) and CTAB (cetyltrimethylammonium bromide) for forming morphologically controlled Sb films. These synthesized materials have been tested as anodes for SIB. The CTAB-assisted Sb anode cell showed better capacity and retention capability, while the SPS-synthesized electrode showed a high capacity of 190 mAh g-1 at 5 C.
Among intermetallics of Sb, alloy heterostructures of Sb with an inactive metal, such as Cu, Ni, Fe, Zn, and so on, are often suitable for SIB anode utility. A Sb/NiSb hybrid has been used as a binder-free SIB anode, exhibiting a superior ICE of 86% along with stable cycling (with a capacity of 521 mAh g-1 at 200 mAh g-1 over 100 cycles), as depicted in Figure 9A[117]. The superior performance of Ni alloying was reflected in the morphological postmortem whereby Sb/NiSb disassembled electrodes (after 100 cycles) presented a stable, intact structure with SEI surface coverage without cracks in contrast to the Sb anode with prominent cracks and no SEI layer visible on the surface of the cycled electrode material. Digital photographs of the same cycled electrode materials also showed that, unlike the Sb/NiSb electrode material that was intact to the Cu collector without delamination, the Sb anode material got delaminated from the current collector almost completely [Figure 9B]. Detailed in-situ XRD [Figure 9C] and ex-situ HRTEM [Figure 9D] analysis paved the way for deep understanding of the mechanism of the (de)sodiation. It was concluded that the initial disappearance of Sb and NiSb peaks during initial sodiation resulted in Na3Sb hexagonal phase formation in multistep sodiation. Additionally, amorphous Ni and Na2CO3 were formed (by decomposition to form SEI). During desodiation, the amorphous Sb formation was traced along with amorphous Ni, which contributed to the electrical conductivity.
Figure 9. (A) (a and b) Charge/discharge profiles of Sb/NiSb and Sb anodes. (c) Cyclic performances of Sb/NiSb and Sb anodes at
A Mo3Sb7 intermetallic SIB anode with extra high capacity has been presented previously by
Current potentials of Sb alloys are inhibited by the formation of powdery material that is not compatible with binders, thus hampering ion penetration and electron transport. Recent endeavors to overcome these issues are much more focused. Shen et al. have fabricated a self-supported intermetallic Sb-Zn alloy anode via pulse electrodeposition[120]. When this anode was employed as a binder-free anode for SIB, it achieved an initial capacity of 377 mAh g-1 at 300 m A g-1. After cycling over 320 cycles, it still maintained a capacity of 261 mAh g-1. The rate performance of the electrode was found to be 308 mAh g-1 at 1,600 mA g-1. In comparison, the fabricated anode using constant potential electrodeposition methodology showed much lower performance. Operando XRD studies showed active participation of Zn and Sb to originate NaZn13 and Na3Sb phases that could transform during the charge/discharge process. The optimum performance of the electrode was ascribed to alloying, structural, and cooperative effects of Zn.
Antimony-based oxides
Oxides of antimony have good alloying potential with Na+. In particular, Sb2O3 and Sb2O4 have high theoretical capacities of 1,103 and 1,120 mAh g-1, respectively. The Sb2O3 with a cubic structure subsequently underwent a conversion-alloying process to yield Sb and Na2O reversibly. However, the low conductivity of Na2O limited its capacity. Akin to tin oxides, low conductivity, huge volume variations, and pulverization problems also persist in Sb2O3 and Sb2O4 anodes[24,121,122]. The conversion-alloying reaction is illustrated as follows:
Octahedral Sb2O3 has been prepared by a low-cost and facile alkaline aqueous synthesis method[123]. As an SIB anode, the material exhibited superior performance (435.6 mAh g-1, 0.1 A g-1 current density, and
A porous Sb2O3@Sb hybrid reported previously by Ma et al. showed a low ICE of 67.9%[125]. However, this hybrid delivered highly regaining capacity in subsequent (de)sodiation cycles with a refined capacity of
Sb2O3@Sb has also been reported by Ye et al. using etching synthetic protocols[127]. The ginger-like Sb having decorated nanosized porous Sb2O3 on its surface efficiently participated in the volume buffering process. The anode showed a capacitive-dominated mechanism of Na+ storage with an optimum storage capacity of 526.2 mAh g-1 over 150 cycles at 1 A g-1. A uniquely presented Sb6O13 with a thin C coating over it has been recently demonstrated as an SIB anode[128]. It showed a cyclic stability of 89.64% (delivering
A recent endeavor to improve SIB anode performance of Sb2O3 has been proposed with exfoliated graphene Sb2O3 in different compositions[129]. The optimum performing SIB electrode showed interesting features unveiled by in-situ impedance spectroscopy whereby varying charge transfer capabilities were detected in the alloying phase, unlike those in the conversion phase. The material showed a respectable potential of
Antimony-based sulfides
The promise of a high theoretical capacity (up to 946 mAh g-1) of antimony sulfide (Sb2S3) has been plagued by a very low electronic conduction (< 1 × 10-5 Scm-1), impractical Na+ diffusion, and huge volume variations in the (de)sodiation process as an SIB anode material. The sodium diffusion process involves the following reactions:
The conversion/alloying reaction involves an overall transfer of 12 mol of Na+. However, a full capacity impact cannot be utilized due to above-mentioned shortcomings. Various nanostructuring, C-matrix addition, and other bimetallic and multimetallic alloys and hybrids have been extensively searched for improved performances of Sb2S3-based materials as SIB anodes[24].
Deng et al. have adopted a “green approach” utilizing natural stibnite ore and sulfur-doped carbon sheets (SCSs)[131]. Sb2S3/SCS composites were developed for SIBs through a quick and effective wet chemical process. The composite Sb2S3/SCS delivered an ICE of 68.82% in comparison with an ICE of 61.27% for stibnite. However, a wide difference in the capacity storage was observed after 100 cycles, where capacities of 455.8 and 190.1 mAh g-1 were retained by Sb2S3/SCS and stibnite, respectively. Xie et al. have proposed a novel material approach by compositing carbon-silicon oxide with Sb2S3 to attain 1D NFs (denoted as
To improve the cycle stability and rate performance of Sb2S3, bimetallic sulfide heterostructure has been developed by adding In2S3 and Sb2S3. To further mitigate the low conductivity, CNTs have been incorporated to attain resultant microspheres[133]. The creation of voids with high surface-active properties led to shorter pathways for rapid Na+ transfer pathways that entrusted a high reversible capacity of
Ternary structured hollow nanorods have been prepared by a solvothermal methodology[134]. They encompassed Sb2S3 at the innermost with intermediate FeS2 sandwiched by N-doped C from outside. The intact structural design could present an outstanding behavior as an SIB anode. It delivered very promising ICE (82.4%), rate capacity (537.9 mAh g-1 at 10 A g-1), and excellent stability (534.8 mAh g-1, CE = 85.7%) after extensive 1,000 cycling at 5 A g-1. Heterogeneous interphases in the superstructure furnished high conductivity and excellent SEI stabilization and accommodated volume variations. In-situ XRD traced the reaction mechanism, with peaks of major active species Sb2S3, Sb0, and Na3Sb detected along with other peaks of NaxFeS2 and Na2S. These were also verified by ex-situ HRTEM. The origin of Na2S was related to accelerated reaction kinetics.
To address pulverization and slow kinetics issues, a Sb2S3@SnS@C nanocomposite has been prepared by
Zhang et al. have proposed a blending wet chemical synthetic strategy for Sb2S3 with the 2D MXenes for optimum SIB anode configuration[136]. The MXene surface-supported Sb2S3 nanoparticles were able to shutter volumetric stresses along with conductive MXene sheets for steadily fast ion/ electron pathways at favored kinetics. The optimized composite (50% Sb2S3@ m-Ti3C2Tx) showed a superior capacity retention (156 mAh g-1 at 0.1 A g-1 ampere density for 100 cycles) and steady performance with a capability of
Figure 10. (A) (a) Stepwise schematic illustration of synthesis of N-C Sb2S3@NCR/MXene, (b) SEM image of N-C ribbons (NCR), (c) Cross-section SEM images of Ti3C2Tx film, and (d) Cross-section SEM images of Sb2S3@NCR/MXene, (e) Rate performances at various current densities, (f) Extended cycling at 1.0 A g-1. (B) (a) Galvanostatic charge-discharge profiles at 0.1 A g-1 and contour maps of in situ XRD pattern for the initial cycle, (b) (Dis)charge profiles with rate behavior at different ampere densities in full cell configuration, (c) Long-term cycling stability at a high current density of 1 A g-1. Inset shows lighting a red LED light when the fabricated full cell battery is bent from 0° to 180°. Reproduced with permission from[137]. Copyright © 2023 Elsevier.
Zhu et al. have reported MoS2@Sb2S3 heterostructure composites enveloped by rGO[138]. The MoS2@Sb2S3 hybrid material with high porosity and a 3D interconnectivity could effectively wet the electrolyte and enhance the kinetics with accelerated ion/electron diffusion at short diffusion pathways. When employed as a negative electrode for SIBs, the composite anode showed fascinating performance (162.1 mAh g-1) after 1,100 cycles. A dual capacitive and diffusion-controlled operated SIB hybrid anode has been fabricated with a simple hydrothermal assisted method by adding Sb salt, rGO, and thioacetamide into a teflon-lined autoclave[102]. The spherical nano-flowered Sb2S3@rGO composite sustained 75.4% (about 544.8 mAh g-1 sustained) of its initial capacity (ICE 72.6%) after 200 cycles at 0.1 A g-1. The 3D assembly assured volume buffering and overall improved kinetics with structural endurance to sustain a rate performance of
Antimony-based selenides
Sb2Se3, a small band gap semiconductor with natural abundance and benignity, has shown a fascinating theoretical capacity of about 670 mAh g-1 as an SIB anode with 12 mol of Na+ per mol of Sb2Se3[139,140]. Simple conversion and alloying reactions for Sb2Se3 can be written as follows:
The persistence of volume changes and sluggish diffusion kinetics in these Sb-based materials are real bottlenecks to achieving high reversible capacities. Recently, many modifications have been reported. They are dominated by those adapting heterostructuring approaches that often utilize carbonaceous compounds. A 2D Sb2Se3 layered anode has been investigated with a focus on mechanistic understandings[141]. Compared to a bare Sb2Se3 electrode with a huge capacity loss, an amorphous carbon composited Sb2Se3 anode retained high gravimetric and volumetric capacities of 378 mAh g-1 and 688 mAh cm-3, respectively, after 50 cycles. The electrode also offered superior rate performance behavior with gravimetric and volumetric rates of about 270 mAh g-1 and 492 mAh cm-3, respectively, at 2C. The capacity drop of the bare Sb2Se3 electrode was related to the formation of Na3Sb accompanied by huge volume expansion, causing large capacity losses after ten cycles. An encapsulated structured Sb2Se3 by rGO has been proposed to achieve high reversibility for the de(sodiation) process[139]. This Sb2Se3/rGO was able to preserve promising capacities (448 and
Figure 11. (A) In-situ XRD patterns of Sb2Se3/rGO SIB anode during first cycle (de)sodiation; (B) XRD patterns within a selected 2θ range during the first cycle against voltage; (C) Stepwise description of the reaction mechanism of the Sb2Se3/rGO hybrid during the (dis)charge process. Reproduced with permission from[139]. Copyright © 2017 John Wiley & Sons, Inc.
Sluggish sodium diffusion has been one of the limiting factors for the low capacity of Sb2Se3-based SIB anodes. Wang et al. have enhanced the capacity by achieving higher Na+-diffusion in their N-doped
A detailed and conceptual representation of the improved performance of the Sb2Se3@CNT hybrid has been reported recently by Ihsan-ul-Haq et al.[143]. The as-synthesized composite could attain a stable rate capability of 454 mAh g-1 at 12.8 A g-1 with 62% capacity retention over 200 cycles at 10 A g-1. Moreover, a power density of 175 Wh kg-1 (at 0.5 C) and an energy density of 5,784 W kg-1 have been achieved in full-cell configuration. Detailed theoretical (ab initio) and experimental investigations were performed along with ex-situ cryo-TEM, CV, and XPS. The CV of the prepared electrode showed highly preserved peaks at potentials of 0.78, 1.36, 1.58, and 1.88 V, proving it reversible desodiation and favorable kinetics. In contrast, the CV of commercial Sb2Se3 signaled highly thick SEI with more electrolyte degradations. Subsequent cycling CV curves showed erratic behavior, signaling unstable SEI and poor reversibility. The cryo-TEM utilization of frozen (-175 °C) electrodes showed a highly thin SEI of about 35.7 nm with a high uniformity in the synthesized Sb2Se3@CNT electrode. Interestingly, the commercially used anode showed a highly irregular and thicker SEI with a thickness of 71.8 nm, which was about double the thickness of the as-prepared electrode.
Hu et al. have also reported a composite derived from Sb2Se3@rGO with a highly commendable performance as an SIB anode[144]. The composite delivered a stable capacity of 260 mAh g-1 at a high ampere density of 20 A g-1. The rGO alleviated volume stresses with reduced particle agglomeration during repeated (de) sodiation cycles. Similarly, Chong et al. have presented an N-doped C-rGO composite of Sb2Se3
A novel combination of Ti3C2Tx sheets and Sb2S3 nanowires has been recently reported[146]. The composite successfully aggravated most of the shortcomings of individual materials symbiotically. On the other hand, the low conductivity, volume stresses, polyselenide shuttling, and slow sodiation kinetics of Sb3Se2 have been bothered by MXenes. As a result, the sheet agglomeration issue of MXenes is delimited by the Sb2Se3. The anode can deliver a capacity of 568.9 mAh g-1 at 0.1 A g-1 after 100 cycles. Even after an extended life span of 500 cycles, a superior capacity (304.1 mAh g-1) at a high current of 1 A g-1 was attained.
Recently, Wu et al. have successfully recognized asymmetric phase transformational characteristics during (de)sodiation of Sb2Se3 nanowires[147]. The multistep mechanism involved in sodiation includes Na+ intercalation, conversion, and sequential alloying involving NaxSb intermediate phases. An incomplete desodiation process has been tracked. It originated from an irreversible capacity fading. The authors were able to trace lateral and surface Na+ diffusion, whereby a fast Na+ surface diffusion was found with radial volume expansions. Moreover, the use of in-situ HRTEM and corresponding fast Fourier transformation (FFT) patterns reflected the tracing of NaxSb2Se3, Na2Se, Sb, NaSb, Na2Sb, and Na3Sb phases showing conversion/alloying transformations.
Phosphorous-based anodes for SIBs
Phosphorous (P) is deemed to be a potential candidate due to its unique properties such as low potential (0.5 V) and ultrahigh theoretical capacity (2,596 mAh g-1). In addition, it is cheap and benign similar to red Phosphorous (RP). Among various allotropes of P, RP has the most optimistic performance characters. In the amorphous phase, it can store high Na+. However, it has a low conductivity (10-14 Scm-1). It also suffers from huge volume changes (490%). The optimum alloying phase in P is Na3P. Although white and layered black P have also been reported to have alloying potential with Na+, unfortunately, white P is toxic and the black P is synthesized under harsh conditions. However, black P has a high conductivity of 300 Scm-1[148,149].
A promising composite with nanoporous RP has been constructed by adding the rGO sheeted matrix to yield the composite (NPRP@rGO). Such addition not only enhanced conductive features of the SIB anode, but also ensured high capacity storage parameters (capacity retainability of 1,249.7 mAh g-1 at 173.26 mA g-1 @ 150 cycles and rate performance of about 656.9 mAh g-1 at 3.462 A g-1)[150]. The improved performance was due to more interfacial contacts and effective electrolyte penetrability along with confinement of NPRP nanoparticles on rGO sheets through P-C chemical bonding. Furthermore, electrochemical impedance spectroscopy (EIS) highlighted the peculiar role of a highly conductive rGO network, which guaranteed effective and quick Na+/e- transportation.
A durable phosphorus anode for SIBs with an extended cycle life span has been developed through coupling of sulfurized Polyacrylonitrile (SPAN), RP, and C black[151]. The hybrid has plenty of P-S bonds that could help withstand large volume variations. It offers a high capacity of about 1,300 mAh g-1 at a current density of 520 mA g-1, with high CE (> 99%) and good cycling performance (i.e., CE = 91% for 100 cycles).
Capone et al. have utilized synergy of particle size of RP, its retainability in the C matrix, and its correlation with the capacity[152]. Through a two-step milling process using wet and dry ball milling to first reduce the particle size and later composite it with the C (graphite), this methodology has impacted the performance of the anode for SIBs application to achieve 1,354 mAh g-1 as initial charging capacity along with an overall CE of 88% over 100 cycles. Xiao et al. have established a correlation between stability of the RP composite electrode in ambient conditions and its performance[153]. The composited electrode (derived from RP and C black) showed a good initial performance (1,070 mAh g-1 of capacity retention over 200 cycles at
To optimize the SIB anode performance in the C compositing of RP, various modest synthetic modifications have been adapted. For instance, Liu et al. have reported a controlled synthesis of RP-rGO composite using ethylenediamine additive to get ultra-small and well-dispersed RP particles on the surface of rGO[154]. The composite showed highly admiring performance with a storage capacity of 2,057 mAh g-1 at 100 mA g-1 while sustaining 65.6% of the capacity over 5,000 cycles at an ampere density of 2 A g-1. During (dis)charging, the strong interaction between rGO and NRP/Na3P inhibited volume variations.
To better protect the RP-C composites from air exposure and the resultant capacity engulfing process, a protective coating of polypyrrole (PPy) has been proposed, which could enhance the conductivity and impart electrolyte and air stabilization[155]. This strategy resulted in stable electrode material at ambient conditions having a high RP content with a stable reversible capacity of 800 mAh g-1 at a current density of 50 mA g-1. Jin et al. have adopted an assisted double annealing strategy to ensure highly porous conductive networks interconnected inside the P host[156]. The extra-short Na+/e- diffusion pathways in the conductive matrix supported the structure from volume variations and exhibited an astonishing longevity over 2,000 cycles with 1,027 mAh g-1 of steady capacity at 4 A g-1 and an equally competitive rate behavior.
An SIB anode with a distinguished performance and real-time performance-mechanistic evaluation has been reported by Liu et al.[157]. In-situ TEM and simulations detected “liquid-like” properties, metallic character, and structural endurance in the RP CNF composite. The highly encapsulated RP inside the C matrix, akin to a core-shell structure, mitigated side reactions. This observation of liquidity of RP on sodiation was dually verified using time-lapse scanning TEM (STEM) images. The optimized capacity of 1,019 mAh g-1 over 5,000 cycles at 1 A g-1 was a benchmark. Details are shown in Figure 12.
Figure 12. (A) (a) A schematic illustration of in-situ TEM setup. (b) STEM image series captured in real-time showing Pred volume expansion during sodiation (scale bar: 200 nm). (c) Simulated SOS according to the Pred morphological evolution of the region labeled with a blue rectangle in image (b). (d) EDS maps of phosphorus (green), sodium (yellow), and carbon (red) elements of the region labeled with a red rectangle in image (b) (scale bar: 200 nm). (e) Length changes of four Pred segments marked in image (b) as a function of time during the sodiation process. (B) Extended cycling of the Pred@CNF anodes with variable current densities. Reproduced with permission from[157]. Copyright © 2020 Springer Nature Limited.
MXene-supported uniform-sized RP nanoparticles were additionally integrated with multi-walled CNTs (MWCNTs), leading to outstanding stability and improved mobility of Na+/e- transportation[158]. The hybrid showed a capacity of 371.6 mAh g-1 at 0.2 A g-1 over 100 cycles. A facile methodology for high-capacity SIB anodes with high mass loadings has been reported by Zhu et al.[159]. A composite of wood-derived carbon and CNTs was coupled to RP. Multi-channeled ion and electronic pathways with a compact but porous framework synchronously improved the capacity retention ability at high mass loadings. Although optimal redox kinetics at a lower mass loading (8.2 mg cm-2) was achieved even at a high mass loading of
Germanium-based anodes for SIBs
Germanium (Ge) has been highlighted for its strong alloying potential with Na+ and less volume expansion than Sn and Sb conversion-alloying anodes. However, its theoretical capacity (369 mAh g-1) is limited for SIBs because 1 mol of Na+ in the resulting NaGe formulation showed unfavored kinetics in the crystalline Ge, which rendered it below the limelight of energy storage community. Although it has 1,000 times superior electrical conduction than Si, the crystalline Ge has a very minor affinity for Na+ that can only provide a capacity of 20 mAh g-1. Thus, many derivatives, especially those with amorphous Ge, have been proposed both theoretically and experimentally, whereby a theoretical capacity of 576 mAh g-1 (corresponding to Na1.56Ge) has been achieved in the amorphous phase[45,166,167]. Lu et al. have presented detailed in-situ TEM-supported evidence of (de)sodiation changes in amorphous Ge nanowires[45]. The unique appearance of pores in the desodiated state and their reappearance upon sodiation in the Ge nanowires were due to sodiation-induced defects during in-situ HRTEM. The large volume expansion of 300% corresponded to a higher sodiated state than NaGe and closely resembled Na1.56Ge. These findings highlight the structural robustness with higher potential of Ge as an SIB anode.
Avery cheap and benign combination of amorphous Ge loaded onto the graphite network has achieved an augmented performance (526 mAh g-1 over 120 cycles along with 160 mAh g-1 at 10 C of ampere density) that highlights possibilities for more superior capacity Ge-based SIB anodes particularly having 2D and 3D structures[167]. A highly functionalized C source derived from dopamine has been added to mitigate the conductivity and sluggish kinetics and stabilize the Zn2GeO4-derived SIB anode[168]. In comparison with the isolated Zn2GeO4 (capacity = 113 mAh g-1 at 0.1 A g-1 over 50 cycles), the C-coated Zn2GeO4 micro-rods delivered a suitable capacity of 317 mAh g-1 (CE 88%) at 0.1 A g-1 over 50 cycles with a rate capability of
A highly reversible capacity-delivering SIB anode synthesized by a template-free solvothermal approach with controlled nanosized (10 nm) germanium phosphide (GePx) organized into micro-spherical architecture has been presented by Tseng et al.[169]. The superior patterning without an additional C matrix could cope with volume stresses and lead to an excellent performance, which is a testament to the advantage of the structural design approach. A high ICE of 65.28% along with a capacity of 704 mAh g-1 was acquired at 0.2 C after 100 cycles. An excellent mechanistic approach for capacity origination and fading has been adopted by Shen et al.[170]. The material demonstrated promising capacity retention and stability, as shown in Figure 13A. The capacity fading issue was traced using in-situ HRTEM with allied techniques to detect species causing capacity degradation during (de)sodiation cycles, as shown in Figure 13B and C[170]. An ICE of 88.61% and a capacity of 330 mAh g-1 over 100 cycles were offered by the electrode in half cell. Expansion during sodiation was captured by TEM in a time-lapse experimental setup, which showed GeP nanoflake expansion from 0.93 to 1.25 µm without any cracking in the nanoflake. The SAED pattern also recorded anisotropic expansions along different planes, leading to intermediate orthorhombic NaGe3P3 that ultimately resulted in amorphous phase NaGe and Na3P. The in-situ TEM also revealed that after completing the first cycle of sodiation, the amorphous phase could not revert back to the crystalline GeP upon desodiation.
Figure 13. (A) Cycling behavior of GeP anode. (B) Morphology and structural changes in GeP: (a-c) before sodiation and (d-f) after sodiation via TEM, HR-TEM, and SAED. (C) Structural evolution of the GeP nanoflake during sodiation recorded by SAED patterns. (a) GeP crystal before sodiation, (b and c) Na+ intercalation into the GeP lattice with the gradual increase in d-spacing (001), (d) Formation of NaGe3P3 intermediate phase, and (e and f) Amorphous Na-Ge and Na-P alloys. Reproduced with permission from[170]. Copyright © 2019 Elsevier.
Li et al. have trailed the path of sodiation in the GeP@C anode for SIBs[171]. The self-healing anode has the capability of structural reformation under a multistep sodiation process involving intercalation followed by the conversion and, finally, the alloying step. This self-healing property was attained due to a low formation energy (-0.19 eV) of the layered material, which further stabilized the graphitic incorporation, creating P-C bonds that synchronously sustained more Na+ at improved kinetics and conduction. The GeP anode has the potential to achieve a high ICE (93%) and a high sodiation rate capacity (360 mAh g-1 at 2 A g-1). Although inspiring, such performance was lower than that of the GeP@C that sustained a high ICE (above 90%), a long cycling life with a capacity of 850 mAh g-1 at 0.1 A g-1 over 300 cycles and a sodiation rate capacity of 533 mAh g-1 at 2 A g-1. The high metallic conductivity of the intermediate NaxGeP with metallic conductive behavior and interlayer bonding compatibility of graphite and GeP created stronger P-C interactions that alleviated capacity performance and stability.
Besides GeP, GeTe has also been demonstrated by many researchers for SIBs anode capabilities. A GeTe/C composite proposed by Sung et al. can yield good gravimetric capacity (98.5% after 100 cycles) and rate performances (704 mAh g-1 at 1 C and 630 mAh g-1 at 3 C)[172]. The conversion/alloying mechanism in the composite anode of GeTe/C material has been investigated using ex-situ XRD and extended X-ray absorption fine structure (EXAFS) during different (dis)charging states. In the first sodiation state, amorphization led to metallic Ge, followed by Na-Ge bond formation. In contrast, the desodiated state showed reappearance of the Ge-Ge bond proceeded by the reappearance of GeTe, which was evident as presented by the following reactions:
A mechanism-directed approach has revealed the significance of amorphous structures in nullifying the influence of stress-induced limitations and interfacial inhomogeneities that plague the SIB anode capacity[173]. For this purpose, 2D porous GeS2 nanosheets with amorphous structures have been constructed. They demonstrated highly stable capacity and rate performance, as shown in
Figure 14. (A) (a) The (dis)charging curves at 0.1 A g-1, (b) Rate performance at various ampere densities, (c) Cycling behavior at
A Ge-based C NF with N, S doping has been fabricated by centrifugal spinning and subsequent thermal treatment methodology to yield N, S-Ge@PCNF[174]. N, S doping, in addition to the porous CF, improved electronic conduction and ionic mobility in a way to sustain a capacity of 443 mAh g-1 over 200 cycles as an SIB anode. The composite also delivered a rate capacity of 300 mAh g-1 at 1 A g-1 in contrast to a capacity of 236 mAh g-1 delivered by the composite with no N or S functionalities (Ge@PCNF). Detailed characterization of the N, S-Ge@PCNFs composite showed uniformly dispersed Ge nano-assemblies on tubular PCNFs. Overall, N, S-doping imparted conductivity enhancement that, together with interconnected PCNFs framework, facilitated Na+ transfer kinetics and buffered volume changes to maximize Na storage.
Silicon-based anodes for SIBs
Silicon (Si), a cheap, abundant, and environmentally friendly material, is an ideal choice for alloying SIBs whereby a single mol of Na+ in the NaSi can result in a theoretical capacity of 960 mAh g-1. However, a very low practically obtained capacity (less than 40 mAh g-1) has been shown by the crystalline Si. Hence, Si was declared electrochemically inactive previously (with +0.6 eV binding energy of Na)[175]. However, many theoretical studies have verified the potential of Si for SIB anodes, although this needs to be experimentally verified[176,177]. Compared to crystalline Si with limited Si uptake, the performance of amorphous Si as an SIB anode is practically more optimistic. The conversion of crystalline Si to amorphous Si after initial cycles also reincarnates the capacity of Si anodes. Various other prepositions for improved anode characters in Si have been proposed, such as C coating[178], porous structuring[179], and other composite modifications[180].
Regarding composite modifications, Kempf et al. have studied the temperature-dependent performance of ion carbon-rich Sn-SiOC composites for SIB anodes[181]. The best performance was shown by the composite (SnO2) pyrolyzed at 900 C, which could deliver a steady capacity of 234 mAh g-1 at 37.2 mA g-1. At a current density of 2,380 mAh g-1, it could sustain a capacity of 131 mAh g-1. Their findings have opened a door for temperature-dependent material synthesis as temperature-performance correlations have been widely addressed to control the morphology and thereby the corresponding capacity, although the alloying mechanism in Si is unclear and somewhat controversial. Very recently, an electrochemical derivatized varyingly ordered Si has been proposed by Li et al.[175]. The combination of short, medium, and long-range ordering induced strong Na-Si interactions well matched for fast ion/-transfer phenomena. The anode in bare form delivered a capacity of 352.7 mAh g-1 at 50 mA g-1 (95.2% CE), while its C composite Si/C supplied a capacity of 449.5 mAh g-1 [Figure 15]. Interestingly, they proved an adsorption-interaction mechanism instead of the commonly evidenced alloying mechanism.
Figure 15. (A) Electrochemical Na+ storage capabilities of MGO-Si and crystalline Si. (a) Cyclic performances of MGO-Si and pristine crystalline Si at 50 mA g-1. (b) Comparison of rate capabilities. (c) Charge/discharge curves of the MGO-Si electrode at different current densities. (d) Long-term cycling stability of MGO-Si at 500 mA g-1. (B) Investigation of the reaction mechanism of the MGO-Si electrode. (a) Contour plot of ex-situ XRD patterns of the (111) and (200) MGO-Si peaks at different cut-off voltages during the discharge cycles. (b) Ex-situ TEM images and (c) enlarged filtered HR-TEM images of MGO-Si before cycling and when discharged and charged. (d and e) STEM-EELS line scan and corresponding EELS spectra of sodiated MGO-Si. (f and g) TEM line scans of MGO-Si when discharged and charged. Reproduced with permission from[175]. Copyright © 2023 John Wiley & Sons, Inc.
Amorphous Si comprising nanocubes or boxes with hollow structures has been composited with rGO to exploit the SIB anode potential of this novel architecture material[182]. The composite imparted stable performance over 2,000 cycles with rate capacities of 261.2 and 73.3 mAh g-1 at 0.1 A and 3 A g-1, respectively. An ordered mesoporous C with Si/SiO2 hybrid constituting 2D mesochannels was found to be highly effective in enhancing Na+ and e- transfer kinetics, suppressing volume variation effects, and providing highly conductive Na+ diffusion pathways. It showed a daring storage capacity of 423 mAh g-1 at 0.02 A g-1 after 100 cycles with an extended longevity (capacity of 190 mAh g-1 at 1 A g-1 after 500 cycles)[183]. The mechanistic-driven phase conversions have been presented in C-composited SiP2 SIB anodes using
A biomass-derived C-sheathed Si composite has been fabricated by Gong et al.[185]. It showed a good affinity for Na+ (de) insertion as an anode and sustained 99.7% of capacity retention after 1,000 cycles. Recently, a ternary composite formed by hybridizing CoSi3P3, FeSi4P4, and CNTs was first pre-deliathiated to create a LiF-rich SEI film that later sustained during (de)sodiation[186]. This novel strategy showed a superior CE of 80% with high initial discharging and charging capacities of 581 and 464 mAh g-1. It sustained a capacity of 417 mAh g-1 over 150 cycles.
Bismuth-based anodes for SIBs
Bismuth (Bi) has been regarded as a “green” choice for energy storage materials because of its non-toxicity, good electronic properties, and layered structure with a larger interlayer distance of 0.395 nm to accommodate Na+ that can reversibly give Na3Bi an optimal gravimetric capacity of 385 mAh g-1[17,187]. Although Bi suffers low volume expansion (250%) (lower than 290% and 430% in Sb and Sn, respectively) compared to other alloying anodes, it still has commercial real-time performance potential[188,189]. Many modifications of Bi have been proposed for stabilized performance, mostly through C compositing. However, C compositing mostly limits the capacity (less than 200 mAh g-1) by imposing an additional barrier layer for ion diffusion[188,190,191]. Although other derivatives, including intermetallics[30], oxides[192], sulfides[193], selenides[194], tellurides[195], and others, have also been reported for high Na+ affinity as SIB anodes[196-198].
Both bulk and nanosized Bi anodes employing various synthetic and structural modifications have been explored widely in recent years to delimit their capacity. However, they have posed considerable bottlenecks. Mechanistic pathways during sodiation in bulk and nanoscale have not been well understood yet[199,200]. Previously, Sottmann et al. have excellently addressed the impact of crystallite size in Bi SIB anodes using synchrotron XRD and XAS[200]. Ball-milled C composited Bi samples for 20 min (Bi/C-20 min) and
Bi nanosphere-based SB anode has been recently updated by Zhang et al.[201]. It showed an ultrahigh and stable capacity with an impressive longevity (10,000 cycles) at a high current density (i.e., 2 A g-1). Although it delivered an unimpressive ICE (53.4%, 422.4/790.3 mAh g-1, C/D), the electrode’s high CEs of above 99% and a good capacity retention of 333.4 mAh g-1 were sustained at an exceedingly high ampere density of
A potential Bi nanosheet array on CNTs for an SIB anode has been recently reported by Liu et al.[203]. It delivered a stable capacity of 311.68 mAh g-1 over 1,000 cycles at 1 A g-1. Importantly, the material showed an equally admired performance when it was applied in full cell configuration with Na3(VOPO4)F@rGO cathodic material, where an operating voltage of above 4 V was achieved with an overall energy density of 221.99 Wh kg-1.
To minimize volume expansion and subsequent pulverization effects in Bi-contained SIB anodes, a novel galvanic replacement (with iodine ion assisted) synthesis of Bi NTs for anodes has been presented
Figure 16. (A) Material characterization. (a) Illustration of Bi-NTs synthesis. (b) TEM image and (c) SEM image of Cu-NWs. (d) XRD powder patterns of Cu-NWs and Bi-NTs. (e and f) TEM images, (g) SEM image, and (h) HRTEM image of Bi-NTs. (i) Elemental mapping and EDS spectrum of Bi-NTs. (B) (a) Rate performance of Bi-NTs, Bi-NPs, and commercial bulk Bi. (b) Comparison of rate performance of Bi-NTs with many previously reported Bi-based anodes for SIBs. Long-term cycling performance of Bi-NTs at (c)
The compromised conductivity and volume enlargements during sodiation/desodiation of Bi anodes necessitates modification at an atomic level to leverage the conductivity along with the addition of some volume buffering media. A Bi-C-based composite has been fabricated in a multistep process that could serve as an ultrafast SIB anode with superb high-temperature utility along with optimum performance at high current densities[189]. The multi-layered design helped mitigate volumetric stresses and particle aggregation during sodiation/desodiation. It also improved ionic mobility, electrolyte wetting, and conductivity [Figure 17A]. The material was tested both in half-cell and full-cell with excellent stability and capacity retention [Figure 17B]. The initial cycle capacity was 301 mAh g-1 at high temperatures. It was diminished to 245 mAh g-1 at high temperatures and a current density of 200 A g-1 with a good reversibility (capacity retention of 78%). The full cell with NVP sustained a capacity of 93 mAh g-1 at 1 A g-1 over 700 cycles and a capacity of 66 mAh g-1 at a current density of 2 A g-1, manifesting an ultrafast charging/discharging in just
Figure 17. (A) Cross-sectional SEM images of (a) CMT, (b) CMT@Bi2O3, and (c) CMT@Bi-C. (d) Image showing surface morphology and (e-g) elemental mapping of CMT@Bi-C. (B) Cycle performance of CMT@Bi-C at 10 A g-1 at high temperature. (C) Post cycling analysis of CMT@Bi-C. Cross-section SEM images before and after battery cycling test for (a and d) CMT, (b and e) CMT@Bi-C, and
Comparison of electrochemical performances of representative alloy-based anode materials for SIBs
Anode materials | Synthetic method | ICE [%] | Charge/ discharge capacity [mAh g-1] | Cycles | Current density [mA g-1] | Electrolyte | Ref. | ||||
Tin-based anode materials | |||||||||||
Tin-based oxides | |||||||||||
SnO2@CNFs/CNTs | Electrospinning | 54.3 | 460.3 | 200 | 100 | NaClO4/EC/PC/FEC | [76] | ||||
SnO2-x/C | Electrospinning | 55.2 | 565 | 2,000 | 1,000 | NaClO4/EC/DEC | [77] | ||||
SnO2/NG | Hydrothermal | 27.8 | 409.6 | 100 | 50 | NaClO4/ PC/EC/FEC | [78] | ||||
SnO2/NiO@C | Electrospinning/deposition/carbonization | 69.0 | 320 | 200 | 100 | NaClO4/EC/DEC/FEC | [205] | ||||
SnO2 NRs/GA | Hydrothermal | 58.4 | 232 | 100 | 50 | NaClO4/EC/DEC | [79] | ||||
SnO2-hard carbon | Hydrothermal carbonization | 36.6 | 184 | 250 | 0.1 C | NaClO4/EC/PC | [80] | ||||
a-SnO2 NH | Oblique angle deposition | 53.1 | 915 | 50 | 2,000 | NaPF6/EC/DMC/FEC | [81] | ||||
SnO2@hCNT | Hydrothermal | 50.0 | 223 | 100 | 100 | NaClO4/EC/DEC | [82] | ||||
SnO2@CC | Solvothermal | 85 | 498 | 100 | 200 | NaClO4/EC/DMC | [83] | ||||
SnO2/MXene | Hydrothermal | 36.54 | 208.6 | 100 | 400 | NaClO4/EC/DEC | [84] | ||||
BTO@SnO2@P-C | Template assisted solvothermal synthesis | - | 144.4 | 10,000 | 10,000 | NaClO4/EC/DEC/FEC | [206] | ||||
Tin-based sulfides | |||||||||||
SnS2/Co3S4 | Co-precipitation and hydrothermal | 59.16 | 1,141.8 | 50 | 100 | NaClO4/DEC/ EC/FEC | [85] | ||||
SnO2@SnS2@NG | Hydrothermal | 54.6 | 100 | 200 | 3,000 | NaClO4/EC/DEC | [92] | ||||
SMS/C NBs | Wet chemical method | 90.8 | 522.5 | 500 | 5,000 | NaCF3SO3/ DEGDME | [93] | ||||
SnS2/EPC | Nanocasting | 68 | 340 | 450 | 2,000 | NaPF6/DME | [86] | ||||
SnS2 QDs/Ti3C2 | Hydrothermal | 55.1 | 345.3 | 600 | 100 | NaClO4/EC/DEC/DMC | [87] | ||||
SnS2/FeS2/rGO | Hydrothermal | 65 | 768.3 | 100 | 100 | NaClO4/EC/DEC/FEC | [207] | ||||
PEG-SnS2/rGO | Hydrothermal | 74.2 | 770 | 100 | 100 | NaPF6/EC/DEC | [88] | ||||
1T-SnS2/RGO | CVD/spray coating | 97.4 | 648.1 | 100 | 500 | NaClO4/EC/ DMC | [89] | ||||
SnS-SnS2@GO | Solvothermal | 69.7 | 450.6 | 100 | 100 | NaClO4/EC/DMC/ MEC/FEC | [90] | ||||
ZnS/SnS2@NCNFs | Electrostatic spinning | - | 174.5 | 1,000 | 5,000 | NaClO4/PC/EC/FEC | [91] | ||||
Tin-based selenides | |||||||||||
SnSe2@C | Solvothermal | 83.1 | 182.7 | 1,000 | 5,000 | NaCF3SO3/DIGLYME | [94] | ||||
SnSe2/ZnSe@PDA | Mechanical mixing | 71.6 | 616 | 1,000 | 1,000 | NaPF6/DME | [96] | ||||
SnSe2 /Ti3C2Tx | Liquid phase reduction and selenylation | - | 245 | 445 | 1,000 | NaPF6/DOL/DIGLYME | [97] | ||||
MoSe2/SnSe2@C | hydrothermal reaction | 63.7 | 591.4 | 110 | 100 | NaClO4/EC/PC/FEC | [98] | ||||
Tin-based phosphide | |||||||||||
Sn4P3-GA | Hydrothermal-phosphidation | 67.3 | 657 | 100 | 100 | NaClO4/ EC/DMC | [102] | ||||
Sn4P3@C | Solvothermal-phosphidation | 64 | 420 | 300 | 200 | NaClO4/ EC/DMC/FEC | [103] | ||||
Micron-sized Sn4P3 | High energy mechanical milling | 89.8 | 719.7 | 100 | 100 | M NaPF6 in diglyme (DGM) | [104] | ||||
Sn4P3@CNT/C | Hydrothermal reaction | 85.2 | 742 | 150 | 200 | NaPF6/DME | [105] | ||||
SnxPy/RGO | Reduction/phosphorization | 54.57 | 421.8 | 100 | 500 | - | [101] | ||||
Sn4P3 HS@MXene | Phosphorization | 82.3 | 373.2 | 150 | 100 | NaPF6/ DEC/ EC/ FEC | [106] | ||||
Sn/Sn4P3@MXene | Solvothermal | 65.7 | 127.8 | 1,000 | 2,000 | NaPF6/ DEC/ EC/ FEC | [107] | ||||
SnxPy/NG | Carbonization-phosphorization | 52.6 | 203.1 | 300 | 1,000 | NaClO4/ EC/DEC/FEC | [99] | ||||
Antimony-based anode materials | |||||||||||
Antimony-based oxides | |||||||||||
Octahedral Sb2O3 | Hydrothermal | 53.3 | 435.6 | 50 | 100 | NaClO4/PC/FEC | [123] | ||||
Sb2O3@Sb | single-step dealloying | 67.9 | 659 | 200 | 29.7 A g1 | NaClO4/PC/FEC | [125] | ||||
Sb/Sb2O3@NCNFs | electrospinning and carbon thermal reduction | 69.9 | 527.3 | 100 | 100 | NaClO4/EC/DMC | [126] | ||||
Sb2O3@Sb | Dealloying/oxidation | 79.8 | 526.2 | 150 | 1,000 | NaPF6/EC/DEC/FEC | [127] | ||||
Sb6O13NPs@C | Hydrothermal | 40.2 | 239 | 170 | 1,000 | NaClO4/EC/DEC/FEC | [128] | ||||
Sb2O3-CNT-Graphene aerogel | Hydrothermal | 46.9 | 360 | 100 | 100 | NaClO4/PC/FEC | [130] | ||||
Sb2O3-EGO | Wet chemical | - | 345 | 100 | 100 | NaClO4/PC/DMC/FEC | [129] | ||||
Antimony-based sulfides | |||||||||||
I-S@MCNTs | Solvothermal | - | 400 | 1,000 | 400 | NaClO4/ EC/ DEC/FEC | [133] | ||||
Sb2S3/SCS | Solvothermal | 61.27 | 455.8 | 100 | 100 | NaClO4/PC/FEC | [131] | ||||
Sb2S3/CS | Electrospinning/hydrothermal | 61 | 321 | 200 | 200 | NaClO4/PC/EC /FEC | [132] | ||||
α-Sb2S3-CuSbS2 | Closed-space sublimation | 82.19 | 506.7 | 50 | 50 | NaCF3SO3 in diglyme | [208] | ||||
Sb2S3@FeS2 | Solvothermal method | 82.4 | 534.8 | 1,000 | 5,000 | NaCF3SO3 in DEGDME | [134] | ||||
Sb/CNTs | Electrochemical desulfurization | 71 | 510 | 200 | 100 | NaClO4/PC/FEC | [209] | ||||
Sb2S3@SnS@C | Hydrothermal/calcination | 79.0 | 442 | 200 | 1,000 | NaClO4/EC/DMC/FEC | [135] | ||||
Sb2S3@m-Ti3C2Tx | Wet Chemical | - | 156 | 100 | 100 | NaClO4/EC/PC/FEC | [136] | ||||
Sb2S3@NCR | Carrboniazation/gas-phase sulfuration | 67.8 | 208 | 100 | 200 | NaClO4/EC/DMC/FEC | [210] | ||||
MoS2@Sb2S3/rGO | Hydrothermal/sulfidation | 80.6 | 162.1. | 1,100 | 5,000 | NaClO4/EC/DEC | [138] | ||||
NF-Sb2S3@rGO | Hydrothermal | 72.6 | 544.8 | 200 | 100 | NaClO4/ PC/EC/FEC | [211] | ||||
Sb2S3/S@S-doped carbon | Template assisted/coupling reaction | 63.5 | 310 | 500 | 1 A g-1 | NaClO4 EC/DEC/DMC/FEC | [212] | ||||
Antimony-based selenides | |||||||||||
α-Sb2Se3/C | Ball milling | 65.7 | 378 | 50 | 50 | NaClO4/EC/PC/FEC | [141] | ||||
ZnS/Sb2S3@NC | Solvothermal | 67.9 | 511.4 | 450 | 1,000 | NaPF6/diEGME | [213] | ||||
Sb2Se3/CNT | Ball milling | 78.9 | 428 | 200 | 50 | NaClO4/EC/PC/FEC | [143] | ||||
Sb2Se3/rGO | Solvothermal | 67.3 | 511 | 50 | 500 | NaClO4/EC/DMC/FEC | [144] | ||||
Sb2Se3@rGO@NC | Solvothermal | 56.0 | 288.5 | 100 | 50 | NaClO4/DEC/EC/FEC | [145] | ||||
Sb2Se3/Ti3C2Tx | Electrostatic Self-assembly method | 87.1 | 568.9 | 100 | 100 | NaClO4/ PC | [146] | ||||
Phosphorous-based anode materials | |||||||||||
NPRP@RGO | Typical redox reaction | 78.5 | 1,249.7 | 150 | 173.261 | NaClO4/PC/FEC | [150] | ||||
P-SPAN | Ball milling | 71.7 | 1,355 | 100 | 520 | NaClO4/EC/DEC/FEC | [151] | ||||
RP/Graphite | Wet milling/dry milling | ≈ 70.0 | 1,354 | 100 | - | NaPF6/EC/DEC/FEC | [152] | ||||
NRP-rGO | Phosphorus-amine-based method | 61.2 | 662 | 1,400 | 2,000 | NaClO4/EC/DEC/FEC | [154] | ||||
RP-Porous C | Ball milling | 87.3 | 1,070 | 200 | 400 | NaClO4/EC/DEC/FEC | [153] | ||||
P@AC@PPy | VDC/interfacial polymerization | - | 484 | 200 | 200 | NaClO4/EC/DEC/FEC | [155] | ||||
RP/CS | vaporization-condensation | 49.3 | 1,027 | 2,000 | 4,000 | HClO4/EC/DMC/FEC | [156] | ||||
Pred@CNF | Vaporization-condensation | - | 1,850 | 500 | 100 | NaPF6/EC/DEC/FEC | [157] | ||||
RP@CNC | Phosphorus-amine method/evacuation-filling process | 67.5 | 1,363 | 150 | 100 | NaClO4/EC/DEC/FEC | [214] | ||||
Sbx-RP(70-x)/C30 | Ball milling | ≈ 90 | 1,650 | 70 | 0.333 C | NaPF6/PC/FEC | [215] | ||||
WDC/CNTs@RP | Vaporization-condensation | 81.21 | 636.3 | 700 | 1 C | NaClO4/EC/DMC/FEC | [159] | ||||
RP-MWCNT/MXene | Blending/grinding | 65 | 371.6 | 100 | 500 | NaPF6/EC/DEC/FEC | [158] | ||||
RP@BP/3DNG | Solvothermal | 65.3 | 706.5 | 200 | 500 | NaClO4/ EC/DMC/ FEC | [216] | ||||
2D-Nanoplatelets RP/SWCNTs | Liquid phase exfoliation | 81 | 451 | 1,000 | 2,500 | NaPF6/ EC/DMC/FEC | [160] | ||||
S-doped P/C | Chemical presodiation strategy | 98.7 | 853.6 | 100 | 200 | NaClO4/ EC/PC/ FEC | [217] | ||||
Germanium-based anode materials | |||||||||||
Zn2GeO4@C | Hydrothermal/pyrolysis | 67.6 | 317 | 50 | 100 | NaOTf/diglyme | [168] | ||||
MGePx | Solvothermal | 65.28 | 704 | 100 | 240 | NaPF6/ FEC/DEC | [169] | ||||
GeP | High temperature - pressure solid state synthesis | 78 | 330 | 100 | 100 | CF3NaO3S/TEGDME | [94] | ||||
GeP/C | Ball milling | 93 | 850 | 300 | 100 | NaCF3SO3/Diglyme | [171] | ||||
GeTe/C | Ball milling | 73 | 315 | 100 | 50 | NaPF6/EC/DMC/FEC | [172] | ||||
GeSe-NWs | Rapid box thermal deposition | 74.5 | 433.4 | 50 | 200 | NaClO4/EC/PC | [105] | ||||
GeS2 | sulfidation- cooling and calcination | 95.1 | 512.8 | 1,000 | 10,000 | NaClO4/PC/FEC | [173] | ||||
N,S-Ge@PCNFs | Centrifugal spinning/heat treatment | - | 443 | 200 | 1,000 | NaClO4/ EC/PC/ FEC | [174] | ||||
Silicon-based anode materials | |||||||||||
Si/SiO2-OMC | Hydrothermal-reduction | - | 423 | 100 | 50 | NaClO4 | [183] | ||||
SiP2/C | Ball milling | 76 | 410 | 100 | 50 | NaClO4/EC/DEC/FEC | [218] | ||||
Si0.07Sb0.93 | Co-sputtering | - | 663 | 140 | 100 | NaClO4/EC/DEC/FEC | [184] | ||||
Si@C | Thermal treatment | - | 174 | 1,000 | 100 | - | [185] | ||||
MGO-Si | - | - | 178.1 | 4,000 | 500 | NaPF6/DEGDME | [175] | ||||
Bismuth-based anode materials | |||||||||||
Bi@N-C | Carbonization | 57.08 | 307 | 400 | 1,000 | NaPF6/DEGDME | [219] | ||||
Bi2Se3@C | Spin coating-thermal treatment | - | 375.3 | 100 | 100 | NaClO4/EC/DMC/EMC/FEC | [220] | ||||
N-C@Bi/G | Hydrothermal/thermal | 78.87 | 260 | 1,000 | 2,000 | - | [221] | ||||
Sn-Bi@C | Hydrothermal/thermal | 83.6 | 461 | 100 | 100 | NaPF6/EGDME | [222] | ||||
Bi@NC | Solvothermal/carbonization | 85.1 | 324.2 | 5,000 | 10,000 | NaPF6/1,2-dimethoxyethane | [223] | ||||
Bi2S3@C@CoS2 | Solvothermal/calcination | 93.6 | 417.7 | 1,600 | 2,000 | NaCF3SO3/DEGDME | [224] | ||||
LC-Bi | Solvothermal | 96 | 264 | 10,000 | 5,000 | NaPF6/DME | [201] | ||||
Bi-NS | Molten thermal reduction | 75.6 | 409.7 | 3,000 | 500 | NaPF6/DIGLYME | [225] |
MATERIAL DESIGN STRATEGIES
Nanostructuring and controlling morphology
Alloying SIB anodes face multiple issues, including high volume expansions, particle aggregation with pulverization, high drop in ICE, low Na+ and electronic mobilities, and unfavored kinetics, all leading to low reversible capacities. From the material’s perspective, many of these limitations can be fixed by opting for rational material design such as nanostructuring and controlled morphological architectures to have a real-world benefit of improved performance. The high and active surface area of nanostructured alloys not only enhances interfacial interactions between electrolyte and electrode, but also offers high Na+ diffusion at shortened paths, particularly over carefully designed nano-morphologies, thereby enhancing the kinetics of ion transfer. Although nanosizing offers the above-mentioned benefits of adding mechanical strength and mitigating pulverization of other detrimental effects, they entail different degrees of drawbacks, including highly reactive nanodomains that participate in parasitic side reactions involving Na+ that can add to unstable SEI, causing more irreversible capacity loss.
The most important challenge in designing alloying materials for SIBs is enhancing their electrochemical performance. In both LIBs and SIBs, the energy storage mechanisms of alloying materials are not significantly different. So, tracking the path of material design strategies for LIB applications has eased the selection of suitable methodologies for uniform and controlled assembling of electrode materials for optimum performances in SIBs. However, it is also important to recognize the differences in the physical properties of Na and Li ions. Many studies have explored the effectiveness of precisely designed morphologies with 0D, 1D, 2D, and 3D patterning ranging from nanowires, NTs, and yolk-shell structures to various porous nano-assembles and many other morphological displays previously proven successful in the LIBs[24,226]. These improved structures have been proven to be effective in volume buffering, improved interfacial contacts, and ionic and electronic conductivities. In many cases, a porous composite structure has dual benefit of involvement of multiple active species at different stages of the sodiation/desodiation, which helps in overall improved kinetics along with shuttering effects of volumetric stresses, which ensures stable capacities over extended cycling[17,28].
Heterostructure and composites design
Recently, compositing methodologies have been much in the limelight for their adaptable surface and bulk properties, matching with desired SIB anode characteristics. In this regard, fabrication of metallic, polymeric, and other hybrid composites often involving a carbonaceous material comes as the foremost choice primarily due to enhanced conductivity and multiple short diffusion pathways with intermittent pores in 3D materials that can afford volume expansions with other added advantages. Often, binary or ternary phase composites have some surface functionalities and defects that garner their effectiveness. Nonetheless, these hybrid phases contribute to side reactions that, in many instances, have been directly linked to initial high-capacity losses[26].
Ahead of others, carbon-based materials always positively contribute to overall performance and long-cyclic capacity retention. It is worth mentioning that capacity boosting, conductivity enhancement, and volume shuttling effect of graphitic carbons in the form of graphene, CNTs, CF, and others often afford 3D interconnected channels that cascade ion shuttling kinetics with improved stability, particularly buffering volumetric expansion and particle aggregation drawbacks[33,227]. Delocalized electrons in the defect/vacancies of graphitic frameworks are grave concerns and entailed as Na+ ion binding sites. Thus, defect/vacancy creation using single and dual heteroatom doping at a certain level is vitally benign in improving SIB anode performance[17,145,228-231].
Tailoring intermetallic alloy composition
Many recent studies have determined the potential of definite intermetallic alloy compositing for SIBs anode. In these advanced heterostructure cum compositing-alloying methodologies, binary, ternary, and even quaternary phases can synergistically and coherently support sodiation/desodiation processes[29,164,232-234]. These synthetic strategies have allowed notable improvisations in the material’s performance as SIB anodes. They have been discussed in detail in optimization strategies.
CHALLENGES ASSOCIATED WITH SIBS
SIBs are on the way to improved performance, particularly aimed at grid-level applications. However, there are still several challenges that need immense attention to dominate in the lithium era. Despite the aforementioned material design strategies in Section “MATERIAL DESIGN STRATEGIES”, there are still main bottlenecks in the vast applicability of alloying SIB anodes. The challenges facing the SIB system include Volume Variations, Voltage Hysteresis, Inadequate Mechanistic Understanding, and Unstable Solid Electrolyte Interphase, as presented in Figure 18. In the case of carbon-composited SIB alloying anodes, scanning the role of defects/functionalities in high initial capacity fading needs to be fixed before presenting SIBs commercially. In the case of intermetallic and conversion-alloying anodes, evolution of intermediate phases and the volume variations need to be probed to deliver sustaining capacities as SIB anodes.
Volume variations
Large-sized Na+ insertion with subsequent alloying during the sodiation causes drastic volume variations that can lead to irreparable structural damage to the alloying host. Accompanying issues include pulverization of the uniquely designed active material to the point of losing electrical contact with current collectors and rendering the SEI unstable, thereby imparting huge initial capacitive losses. Typical volume expansions for alloying anodes vary. They are often reported to range from 200% to 400%, with the highest expansions in the most promising anodes such as Sn, Sb, Bi, and P. Besides universal methodologies of C compositing, various structural engineering methodologies to introduce a volume buffering medium have been vastly reported[17,29].
Voltage hysteresis
Voltage hysteresis is another challenge that both LIBs and SIBs alloying anodes suffer. Despite various prepositions made for the origin of voltage hysteresis, the root cause is not very well understood yet. Voltage hysteresis reflects capacity loss. It has both kinetic and thermodynamic origins. Unstable SEI, low ionic motilities, structural transformations, and other barriers in ionic/electronic transportation have been mostly nominated as causes of voltage hysteresis.
Voltage hysteresis represents a potential gap between discharge and charge cycles. It highlights an unsteady performance of the selected electrode material in SIBs. Electrodes showing large voltage hysteresis have impractical cyclic efficiency. Large voltage hysteresis is associated with other capacity deprivation problems. Reaction overpotential, ohmic polarization, and component inhomogeneity between charged and discharged stages are labeled as culprits for overpotential. They can be determined using galvanostatic and potentiostatic intermittent titrimetric analysis. Among these three factors, composition inhomogeneity arises from diffusion of Na+ in the solid state with different Na+ rich and Na+ deficient layers being formed at the material's surface during sodiation and desodiation, respectively[17,41].
Unstable solid electrolyte interphase
The most addressed and key issue in LIBs and SIBs is unstable SEI formation that is always formed at an electrode-electrolyte interface. Anode materials having high fermi levels can induce reduction of electrolytes, whose LUMO lies below that of anode material. The SEI layer is ion-conducting in nature. It is an electron insulator. However, it does offer much resistance to Na+ diffusion in a thick form. While a stable, suitably thin SEI is vital for suppressing electrolyte side reactions although, in most cases, it is highly difficult to have a stable and non-degrading thin SEI. One of the major capacity-fading roles is displayed by this SEI, which often constantly forms and degrades, causing a substantial loss of Na+. Particularly for alloy anodes, stabilizing SEI is quite difficult. When stress generated at the interface during sodiation/desodiation exceeds the stability limit of SEI, it causes its degradation and makes a fresh surface prone to generation of new SEI that eventually gets peeled off after a certain thickness, making a portion of Na+ unavailable. Many studies have detailed the origin, morphology, and mechanism of SEI formation, along with its detection and evidence during electrochemical charging/discharging. SEI formation and its stabilization have been extensively presented in the literature and various modulating methods such as the use of electrolytes and additives have been proposed[17,66,235-237]. A detailed account is presented in the optimization section of electrolyte.
Inadequate mechanistic understanding
Multicomponent battery systems need detailed mechanistic understandings that vary with the type and morphologies of materials used as anodes, cathodes, electrolyte systems, and many other factors involved in the charging and discharging processes. Unfortunately, for alloying SIB anodes, there is little consensus on the mechanism of charge storage as the compact and sealed battery environment presents challenges in
OPTIMIZATIONS OF SIBS
The development of SIBs started alongside LIBs. However, their low energy density, coupled with other effects partly due to large Na+ size, has not ascended at a commercial scale to replace costly LIBs. Although many commercial-scale SIBs have been presented by companies such as Novasis, Faradion, Natron Energy, AGM, TIAMAT, Altris, and others, they have proven renaissance for improvisations in SIBs performance[238]. Recently, many companies (including China’s BYD and Swedish NORTHVOLT) have claimed breakthroughs in SIBs, nominating them as “Rising stars” for commercial-scale applications[239]. Despite that, it seems realistic that the current decade will be dominated by high-performing LIBs because most research studies on SIBs put forth hitherto suffer from major capacity degradation processes that need to be addressed immediately. In this regard, many optimizations in electrolyte, binder, structural, and surface engineering are necessary to cope with challenges alloying SIB anodes face.
Efficient electrolyte system
A major concern in SIB alloy anodes is their low ICE with a major contributor being the electrolyte, which participates in formation of SEI, which, in turn, determines capacity retention, cyclic stability, and performance. Particularly, the stability of an SEI and the overall capacity and efficiency highly depend on the electrolyte system. Few electrolyte systems have proven their compatibility when coupled with alloying anode materials in SIBs. A relatively thin and sustaining SEI formed from a well-matched electrolyte and additive combination consumes electrolyte molecules and Na+ into the active passivation layer. The electrolyte in SIBs should have the following characteristics: (i) a low difference between its LUMO and the electrode's Fermi level; (ii) a good ionic conductivity along with lower viscosity; (iii) anode and cathode material’s compatibility with the electrolyte; (vi) thermal stability; and (v) cost effectiveness[31].
Commonly employed electrolytes in SIBs include NaClO4, NaPF6, sodium bis(trifluoromethane) sulfonamide (NaTFSI) in conjunction with organic solvents such as ethylene carbonate (EC), propylene carbonate (PC), dimethyl carbonate, and so on[66,240-242]. However, each electrolyte system inherently involves some capacity fading phenomena to a different degree. NaClO4 is less moisture adsorbing than NaPF6. However, when they are combined with carbonate-based solvents, both could not minimize ICE loss in a wide variety of SIBs, although ICE losses are much diminished in ether-based solvent systems (diglyme, triglyme, DME, tetrahydrofuran (THF), and so on)[243]. Other than ether-based additives, the most concerned and effective electrolyte additive in SIBs is fluoroethylene carbonate (FEC), which, in combination with other additives, has a pivotal ICE-sustaining role[26]. Electrolyte additives not only play a vital role in stabilizing the SEI and electrochemical capacity retention, but also have diverse effects on the electrolyte itself. Electrolyte additives are believed to participate in SEI formation and stabilization. They can also inhibit side reactions that deteriorate the SEI[65].
The recent shift toward the utilization of ionic liquid[244-246] and solid[247,248] electrolytes has ample success stories. A wide potential window, low flammability, and high temperature workability are some of the features that have received much interest. However, both ionic liquid[244-246] and solid[247,248] electrolytes need optimizations in terms of electrochemical and techno-economic limitations for their commercialization. Although various models for SEI have been proposed, there is very little knowledge of SEI formation mechanism and its in-situ analysis in alloy-based anodes[249-251]. The composition of the SEI film can give some clues about the species’ involvement along with possible underlying reaction mechanisms. Recently, Zhang et al. have designed 3D carbon-coated Bi nanospheres as SIB anodes[201]. These anodes showed a stable performance of up to 10,000 cycles at 2 A g-1 with a rate capability performance of 94% under
Lately, Yang et al. have also given details about the origin of SEI components by performing in-depth XPS studies[75]. They also validated the presence of organic and inorganic species evolving from electrolyte decomposition in different electrolyte systems with their effects on SEI stabilization. In the DEGDME solvent, a stable fluorinated SEI evolved by decomposition of the NaPF6 electrolyte optimized the SIB performance. Also, the DEGDME and its complex [NaPF6-DEGDME]+ presented a higher HOMO energy and helped form sustaining cathode electrolyte interphase, as shown in Figure 19A. In fact, electrolyte has various interactive roles, among which solvation of Na+ is very critical as it can affect SEI growth and its stability. The DEGDME solvent has recently been shown to be able to create a hybrid organic-inorganic SEI that synergistically involves carbonaceous materials to impart stability to SEI without much ionic hindrance. The DEGDME solvent also interacts with the C in heterostructured composites to maintain structural variations upon cycling. The cyclic performance in DEGDME and EC/PC solvent [Figure 19B] highlighted improved capacity and cyclability. The flair of DEGDME solvent for better compatibility with NaPF6 than the commonly used EC/PC solvent was proved by DFT calculations that showed a higher energy difference for electron promotion from HOMO to LUMO than in commonly employed carbonate-based electrolytes[241].
Figure 19. (A) Diagram of HOMO and LUMO energy levels showing electron acceptance and donation ability of EC, PC, and DEGDME. (b) AIMD simulation snapshots of DEGDME-based electrolyte. (c) Radial distribution functions g(r) of Na-O and Na-F pairs in both electrolytes. (d) Solvation energy and (e) desolvation energy of Na+-solvent complexes. (f) Schematic illustration of reaction kinetics of Sn@NC full cells with DEGDME-based electrolyte. (B) (a) Cyclic performances at 1.0 A g-1 in both electrolytes (b) Long-term cycling for 10,000 cycles of composite anodes at 5.0 A g-1 in DEGDME-based electrolyte. Reproduced with permission from[75]. Copyright © 2023 John Wiley & Sons, Inc.
Effective binders
The biggest dilemma of high-volume expansions in alloying anode materials in SIB may have multifactorial origination. However, the role of the binder is very crucial in this regard. The binder not only holds the active material onto the current collector, but also plays a role in managing the stress for volume variations, particularly in alloying anodes. In addition, it safeguards the integrity of isolated electrode particles. Huge volume variations also have detrimental effects on the electrical conductivity of the active material, which is ensured by an efficient network established by the binder. The commonly employed polyvinylidene fluoride (PVDF) binder has been proven to be inefficient in SIBs. Its decomposition products have been found to be responsible for low ICE with a negative impact on long-term cycling performance[26,252,253]. Moreover, the binder has a typical role in minimizing the pulverization issue. It also mitigates regrowth of fresh SEI and sustains SEI to ensure a high ICE. Other than PVDF, many binders have shown to have suitable performances in SIBs, including Chitosan, sodium carboxymethyl cellulose, sodium alginate, and so on[252,254-256]. These binders have very little volume swelling effects in organic electrolytes, which is beneficial for inducing solvent-binder interactive effects that can marginalize the capacity. The 3D cross-linkage connectivity between the binder and the alloying anode material can highly ameliorate adhesion in electrode components, involving greater interaction with the metallic current collector to ensure high electrical conductivity and integrity. Feng et al. have reported that their Sb@C anode shows capacities of 553 and 435 mAh g-1 over 50 cycles at 50 mA g-1 using sodium alginate and PVDF binders, respectively[257].
Performance influence of binder modifications has very recently been detailed for alloy-type SIB anodes by Yao et al. in a comprehensive study focusing on the cross-linking effect of glycerin (GLY) on
Some recent studies have focused on binder-free electrodes where C-fiber often hosts the active material assembly either in the form of a support on which the active material is grown or embedded inside the C matrix. Another approach is to use C foam as a binder-free support[120,259]. A binder-free SIB alloying anode constructed from MOF-derived C and Bi nanodots has shown a higher performance than the bare
Reducing voltage hysteresis
Many recent efforts in reducing voltage hysteresis have shown improved cyclic performance. A recent attempt has been made to identify the role of phase transformations and hysteresis using Na-Sb and Na-P systems with electrode materials having stable phases to minimize polarization effects and have better cyclability[261]. Similarly, the Sb8Bi1 alloy anode in SIBs has been recently selected for delivering a high ICE of 87.1% at 0.1 A g-1. The electrode exhibited low polarization effects. It could furnish a high capacity of
Structural defects and surface engineering
One of the essential and perhaps overemphasized strategies of tuning the performance of the battery materials is by performing structural modifications that often can achieve suitably sustaining performances. Various methodologies have been reported, including nanosizing, designing functional surfaces by introducing functionalities, coatings, elemental doping, multicomponent design, and compositing to construct 1D, 2D, and 3D structures to introduce interconnected channels, pores, and other short pathways to achieve high conductivity with efficient ion/electron transfer and manage volume expansions. Although many of these optimizations for high-capacity SIB electrodes are difficult to achieve without compromising on one or more performance aspects, they always show some negative traits. For example, nanosized alloy particles have a high surface area that can effectively shuttle more Na+ along with high electrode/electrolyte interfacial contacts. However, when the surface area is beyond a certain size, it can enhance mechanical cracking of electrode materials similar to in alloying nanostructures, which bear high volume expansions during charge/discharge. An exceptionally high surface area also offers highly reactive surfaces for undesired reaction pathways. Alongside smaller nanoparticles, incomplete alloying can occur because smaller equilibrium ion concentrations can lessen the overall capacity. Many nano morphological optimizations, including porous architectures, nanorods, nanowires, nanosheets, hollow structures, and yolk-shell morphologies, have effectively yielded high capacity and managed other shortcomings in alloying SIBs[24,228,230,265].
Structural resilience of nanostructured alloying anodes during the charge process is critically important as it is directly linked to the performance. However, in some instances, it has been reported that structural evolutions also have a cohesive impact on capacity retention, as crystalline to amorphous phase conversions are often detected during in-situ characterization[81,173]. Similarly, dual active/inactive phases have also been shown to take part alternatively during charge/discharge phenomena. Versatile and multi-modal stability is often ensured by introducing some carbonaceous material such as CNTs, graphene, N-doped C, C NF, hard C, and others in bulk or using some coating methodology. Besides improving conductivity and Na+ transport kinetics, it offers functional surfaces for sustaining the capacity. Nanosizing effects have also been imparted in C-derived alloy anodes. Volume buffering effects are primarily of interest when carbonaceous hybrid alloying anodes with low ICE are targeted[201,228,266].
Various intermetallic alloys have been tuned with carbonaceous materials for stable SIB anode performance. For example, when ultrasmall 3D-SnSb/NPC (nanoporous carbon) was utilized as an SIB anode, it furnished an optimal capacity of 266.6 mAh g-1 over 15,000 cycles at 2 A g-1 with rate performance of
A recent exciting electrode design has been presented by Kang et al.[268]. They compared anode performances of synthesized AlSb, AlSb@C, and oxide terminated Al/AlSb/SbxOy multicomponent anode with N-doped C. The superb oxide terminated array (Al/AlSb/SbxOy) enabled the anode to deliver a stable 258 mAh g-1 capacity at 10 C, much higher than previously reported micro-sized Sb SIB anodes. The specific nanoparticle structural assembly helps buffering volumetric strains and stable SEI creation, ensuring longevity of the anode in half and full-cell operations.
An intermetallic Sn-Bi SIB anode has been recently proposed, showing stable and sustaining capacity as an SIB anode. The optimized SnBi composition showed a capacity of 508 mAh g-1 in the first cycle and a capacity of 420 mAh g-1 at 1 C after cycling 200 times[269]. The synchrotron operando XRD showed the origin of different Sn and Bi intermetallic alloyed compositions during (dis)charging phases. At different electrochemical potentials, Sn and Bi particles in the intermetallic get sodiated to various levels. When one phase is active (sodiated), the other is dormant. In this way, the system is buffered to manage volumetric stresses. An anode-less configuration has recently been opted to utilize the Bi array deposited onto a copper foil to form Cu@Bi alloy[270]. The fabricated material was tested in both half-cell and full-cell configurations after initial activation. The full cell comprising the Cu@Bi anode and NVP as cathode delivered a capacity of 95.6 mAh g-1 over 80 cycles. A binary melt-spun alloy Fe-As has recently been tested as an SIB anode by Patel et al.[271]. This anode delivered an ultrahigh and stable capacity of 965 mAh g-1 at 50 mA g-1 over 400 cycles Additionally, it demonstrated an excellent sodiation rate performance of 668 mAh g-1 at 2 A g-1, surpassing most of the common SIB anodes. Beyond that, melt-spun fibers showed 770 mAh g-1 of sodiation capacity after 200 cycles at 50 mA g-1 (with over 97% CE). Detailed ex-situ XRD highlighted the compositional and phase transformations, confirming the formation of Na3As during sodiation and the appearance of an iron-rich phase. This study highlighted the excellent potential of intermetallic alloy materials for being opted as commercial anodes for SIBs. Similarly, Sb-Zn electrodeposited intermetallic alloy showed superior Na+ storage capacity as a binder-free anode in SIBs, whereby different Sb and Zn alloying with sodium was traced during the charge/discharge process[120].
Recently, a hybrid SIB anode composed of FeSb2S4/Sb/rGO that offers a highly stable capacity of
In fact, a unique strategy has recently been employed by Li et al. to fabricate a modified anode material[273]. They incorporated Sn into copper sulfide and then partially substituted Sn with Zn, which garnered a sodium uptake capability of the electrode with stable performance and fast charging behavior. The Sn-Zn-based copper sulfide maintained a capacity of 560 mAh g-1 at 0.2 A g-1 with a retention capacity of 100% for 80 K cycles. The material furnished a highly fast charging of 4 s per charge with an input of 190 mAh g-1. A freshly developed mesoporous Sn/SnO2-Ni@C composited alloy has shown marked performance as an SIB anode. The composite furnished a stable capacity of 342.6 mAh g-1 at 0.2 A g-1 after 200 cycles while the anode delivered a capacity of 219.3 mAh g-1 at 1.0 A g-1 when cycled 1,000 times[274]. The excellent interplay between the Sn/SnO2 and Ni has mitigated effects of volume expansion and capacity degradation. Another fascinating study has highlighted a 3D core-shell heterostructured SnO2 coated with barium titanate (acting as core) and covered with phosphorous-doped C (BTO@SnO2@P-C) [Figure 20A][206]. The unique selection provided the built-in electric field effect generated by the BTO layer due to ferroelectric polarization, further augmented by the piezoelectric effect that could increase due to volume expansion during alloying of SnO2 in the core-shell structure. These synchronous effects highly improved the performance as an SIB anode with an awe-inspiring reversible capacity of 144.4 mAh g-1 over 10,000 cycles at 10 A g-1 with extra fast charging (99% charged in 1 min), as shown in Figure 20B. Detailed in-situ XRD studies [Figure 20C] proved that the BTO (110) plane showed evident peak shifts during (dis)charging and participated in the stability of the anode.
Figure 20. (A) (a) XRD pattern of BTO@SnO2@P-C and SnO2@P-C, (b) SEM image, (c) TEM with SAED pattern image (inset), and (d) HR-TEM image. (B) Long cycle performance of BTO@SnO2@P-C at (a) 2 A g-1 and (b) 10 A g-1. (C) In-situ XRD analysis of
A rGO composite of Sn/Sb as an SIB anode delivered a capacity of 320 mAh g-1 over 300 cycles at
Bhar et al. have recently reported a freestanding carbon fiber-based composite electrode composed of
Recently, Sb porous hollow microspheres presented by Hou et al. have shown a good SIB alloying anode potential, delivering an ICE of 64.6% (a charging capacity of 634.6 mAh g-1 at 100 mA g-1) with a reasonable rate performance of about 313 mAh g-a over 100 cycles at 3,200 mA g-1[280]. Sn nanorods modified by
Another common strategy is to create defects and oxygen vacancies with potential to enhance the intrinsic electronic conductivity and sodiation potential of a material[61,229,281,282]. Amorphous-crystalline defect-bearing hetero-conjunctions formed between the amorphous SeP and crystalline graphene conductive framework have been proposed to facilitate the SIB performance of SeP@HCG (high conductive crystalline graphene)[283]. The material effectively sodiated into an amorphous NaxSeP phase, leading to nanocrystalline Na2Se and Na3P to attain a high capacity of 855 mAh g-1 at 0.2 A g-1 with an extended cyclability. After 500 cycles, it maintained a capacity of 732 mAh g-1.
Recently, Liu et al. have reported a high-capacity 3D Bi-derived electrode that undergoes a dual alloying-stripping mechanism, unlike conventional alloying anodes[284]. The bulk Bi material was converted to a 3D framework after activation and alloying, followed by subsequent plating, resulting in a sodiophilic Na@3D Na3Bi framework that dually exploited SIBs and sodium-metal batteries (SMBs)’s characteristic ion exchange process. Above all, the ultimate capacity delivered by the anode was about 7.7 times higher than that of the alloying Bi anode, retaining a capacity of 2,000 mAh g-1 for 800 h at a current density of 1 A g-1. The hybrid 3D-designed anode could shuttle Na+ for alloying in combination with Na+ plating in the inner space of the Na3Bi network, which suppressed dendrite formation, unlike in SNBs. Similarly, MoS2/SnS showed a superior capacity retention behavior to store 634 mA g-1 after 100 cycles at 2 A g-1. It also sustained a capacity of 745 mAh g-1 at 10 A g-1 during rate capacity cycling[285].
Suitable cathode materials
There are a variety of cathode materials in SIBs, including layered sodium transition metal oxide-based materials, polyanionic compounds (sulfate, oxalate, phosphates, etc.), Prussian blue analogs, and others[238,286,287]. Although many of these cathodes have demonstrated their commercial viability, such as oxides, Prussian blue, Prussian white analogs, and Na3V2(PO4)2F3 (NVPF), unfortunately, all of them are coupled with hard carbon anode materials without any major research breakthrough using alloying-based anodes[238,287]. Noteworthy, each category poses specific shortfalls that need to be overcome. For example, oxide-based materials always have stability issues despite their high capacity. Polyanionic cathodes have a poor capacity and low electronic conductivity known to hamper their utilization. Prussian blue analogs (PBAs) suffer from lower tap density and the presence of water of crystallization in their structures known to pose safety threats and thermal runaway issues. The most critical challenge for cell SIBs is the choice of suitable anodes with a matching high capacity and stability cathode material that could offer a high voltage. The potential of composited cathodes can also improve the overall performance of SIBs. This must be considered for alloying-based anode counterparts[59,288]. Vast material, structural, and compositional options for alloying anodes need immense experimental and theoretical screening using the latest machine-learning tools to determine their compatibility with commercially successful cathodes to achieve high-performing SIB cells ultimately.
Other optimization parameters
Alloying SIB anodes have been modified to entrap more (de)sodiation capacity using various strategies including presodiation, dealloying, and so on. The presodiation strategy offers an excellent solution to complement the Na+ concentration consumed and depleted in the SEI formation and side reactions. Presodiation at a certain level in both anode and cathodes is known to augment the SIB performance. Some recent studies have highlighted its importance[289,290]. Presodiation must be optimized to maximize benefits, as in the Sn-C composite anode presented previously. The effectiveness of presodiation has been evident. An optimized presodiated anode can deliver a steady capacity of 130 mAh g-1 without capacity losses for
Various researchers have also adopted dealloying to achieve the optimized morphology for SIB alloying electrode materials, although dealloying has not been explored extensively. In this strategy, some element (sacrificial element) is often selectively removed to achieve the desired 3D porous/hollow/network structure. Some reports have claimed to attain superior and stable capacities using a dealloying methodology. For example, Sb2O3@Sb proposed by Ma et al. could sustain 200 mAh g-1 at a high current rate of 29.7 A g-1 in rate capacity[125]. It showed 99.8% cyclic capacity retention. Recently, a novel approach to improve the performance of alloying SIB anode has been highlighted. In this approach, K+ incorporated into the electrolyte can highly improve the performance of Sn alloying anode, yielding an energy of 565 mAh g-1 over 3,000 cycles at 2 A g-1[71]. Dimensional structure and electrode formulations can also be used for achieving the optimized SIB performance using (a) tin; (b) antimony; (c) phosphorus; (d) germanium; (e) silicon; and (f) bismuth-based anode materials. These are provided in Table 3.
Effects of dimensional structure and electrode formulations on optimized SIB performance using alloy-based anodes
Dimensional structure | Materials | Synthesis methods | Electrolyte system | Electrode composition (%) | ICE (%) | Specific capacity (mAh g-1) | Current density (A g-1) | Cycle number | Rate Performance (mAh g-1) | Current density (A g-1) | Ref. | |||||
Tin-based anode materials | ||||||||||||||||
0D | Sn@CFC | Electrospinning method | 1M NaClO4 in EC/DMC with 5% FEC | Freestanding | 42.3 | 255 | 0.05 | 200 | 100 | 1 | [293] | |||||
Porous carbon nanocages@Sn | Template-assisted CVD and in-situ reduction | 1M NaClO4 in EC/PC with 5% FEC | AM:carbon black:PVDF (80:10:10) | 73 | 202 | 1.28 | 550 | 188 | 2.56 | [294] | ||||||
Sn naniparticles@C | Aerosol spray pyrolysis method | 1M NaClO4 in EC/DEC | AM:super P carbon:CMC (70:15:15) | 67 | 415 | 1 | 500 | 349 | 4 | [295] | ||||||
1D | Sn nanofibers | Electrodeposition | 1M NaClO4 in PC with 2% FEC | - | 68 | 776 | 0.1 C | 100 | ~260 | 5 C | [296] | |||||
Sn@N-doped carbon | Annealing | 1M NaPF6 in DME | AM:acetylene black:sodium carboxymethylcellulose (80:10:10) | 78.4 | 347 | 5 | 10,000 | 437 | 5 | [75] | ||||||
3D | Microsized gray-Sn | Commercial | 1M NaPF6 in DGM | Am:acetylene black:CMC (70:20:10) | 80 | 451 | 2 | 3,500 | 464 | 4 | [297] | |||||
Porous Sn | Replacement reaction | 1M NaPF6 in DME | AM:super P carbon:PVDF (70:20:10) | - | 660 | 2.5 | 400 | 667.6 | 6.5 | [298] | ||||||
Antimony-based anode materials | ||||||||||||||||
0D | Sb@NS-3DPC | Pyrolysis | 1M NaClO4 in PC with 10% FEC | AM:conductive carbon:CMC (70:15:15) | 61.1 | 259 | 10 | 20,000 | 283.8 | 10 | [299] | |||||
1D | SbSn nanoarrays | Electro deposition and annealing | 1M NaPF6 in DME | AM: carbon black:PVDF (80:10:10) | 83 | 511 | 2 C | 800 | 521 | 5 C | [300] | |||||
Bi0.75Sb0.25 pyramid arrays | Electro deposition and annealing | 1M NaPF6 in DME | AM:carbon black:PVDF (80:10:10) | 86 | 284 | 0.5 | 2,000 | 335 | 2.5 | [301] | ||||||
2D | antimonene/C | Liquid-phase exfoliation | 1M NaPF6 in EC/DMC with 5% FEC | - | 71.2 | 451.3 | 0.2 | 150 | 334.5 | /5 | [302] | |||||
few-layer antimonene | Liquid-phase exfoliation | 1M NaClO4 in EC/DEC with 5% FEC | AM: carbon black:CMC (65:20:15) | 64.7 | 620 | 0.5 C | 150 | 429 | 5 C | [303] | ||||||
3D | Nanoporous Bi2Sb6 | Dealloying | 1M NaPF6 in PC with 5% FEC | AM:acetylene black -super P:CMC (60:25:15) | 69.8 | 258 | 0.2 | 2,000 | 304.2/15 | [304] | ||||||
Phosphorous-based anode materials | ||||||||||||||||
0D | P/TiN | Mechanical milling method | 1M NaClO4 in EC/DEC with 5% FEC | AM:super-P carbon:CMC (80:10:10) | 86.8 | 91.3 | 0.2 | 300 | 339 | 20 | [305] | |||||
Red P@ nitrogen-doped porous carbon tubes | Vaporization condensation method | 1M NaClO4 in EC/DMC with 5% FEC | Freestanding electrode | - | 1,157 | 2 | 100 | 572 | 10 | [306] | ||||||
Hierarchical porous carbon nanospheres/P | Vaporization condensation process | 1M NaClO4 in EC/DEC | AM:acetylene black:PVDF (80:10:10) | 75.1 | 861.8 | 5 | 1,000 | 831.1 | 10 | [307] | ||||||
red@black P/N-graphene | Solvothermal treatment | 1M NaClO4 in EC/DMC with 5% FEC | AM:Carbon black:PVDF (80:10:10) | 65.3 | 465.5 | 10 | 1,200 | 521.3 | 10 | [216] | ||||||
P@CNTs backboned mesoporous carbon | Vaporization condensation conversion | 1M NaClO4 in EC/DEC with 5% FEC | AM:C-black:Na-CMC, (75:10:15) | 69.8 | 580 | 2.5 | 800 | 430 | 8 | [308] | ||||||
Nanoscale red P/rGO | Phosphorus-amine-based method | 1M NaClO4 in EC/DEC with 10% FEC | AM:super P acetylene black:PVDF (70:20:10) | 61.2 | 390 | 5 | 5,000 | 718 | 5 | [154] | ||||||
1D | Se-induced fibrous nano red P | Selenium-induced method | 1M NaClO4 in EC/PC with 5% FEC | - | 89.7 | 1,785 | 1C | 2,800 | 1,190 | 25 C | [309] | |||||
Red P@carbon nanofiber | Vaporization condensation method | 1M NaPF6 in EC/DEC | Freestanding films | - | 1,850 | 0.1 | 500 | - | [157] | |||||||
2D | Phosphorene/Ti3C2Tx MXene | Self-assembly | 1M NaClO4 in EC/PC | AM:carbon black:CMC (70:20:10) | 63.1 | 340 | 1 | 1,000 | 193 | 5 | [310] | |||||
phosphorene graphene | Self-assembly | 1M NaPF6 in EC/DEC with 10% FEC | - | ~80 | 2,440 | 0.05 | 100 | - | [311] | |||||||
phosphorene graphene | Electrochemically exfoliated and solution method | 1M NaClO4 in PC with 5% FEC | AM:super P:CMC (70:15:15) | 80.6 | 1,582.6 | 1 | 200 | 1,499 | 5 | [312] | ||||||
Black P/graphene | Pressure synthesis | 1M NaClO4 in DMC with 10% FEC | Freestanding films | 89.5 | 1,250 | 1 | 500 | 720.8 | 40 | [313] | ||||||
3D | Sb7-RP63/C30 | High-energy ball milling process | 1M NaPF6 in PC with 2% FEC | AM:super-P:sodium alginate (70:15:15) | 88 | 2,356 | C/3 | 70 | 1,779 | 2 C | [215] | |||||
Red P@Carbon nanocages | Evacuation-filling process | 1M NaClO4 in EC/DEC with 10% FEC | AM:carbon black:PVDF (70:20:10) | - | 1363 | 0.1 | 150 | 750 | 5 | [214] | ||||||
Nanoporous red P@rGO | Boiling | 1M NaClO4 in PC with 5% FEC | AM:super P:CMC (70:15:15) | 78.5 | 1,250 | 0.17 | 150 | 657 | 3.47 | [150] | ||||||
Bismuth-based anode materials | ||||||||||||||||
0D | Bi@N-doped carbon nanospheres | Annealing | 1M NaPF6 in DME | AM:super P:PVDF (70:20:10) | 36.5 | 235 | 10 | 2,000 | 152 | 100 | [314] | |||||
Bismuth Nanoparticle@Carbon Composite | Annealing | 1M NaPF6 in DME | AM:Super P:PVDF (80:10:10) | 50.3 | 265 | 8 | 30,000 | 232 | 60 | [315] | ||||||
1D | Bi nanowires@graphene film | Vacuum filtration assembly | 1M NaPF6 in DME | - | 82.7 | 276 | 1 | 1,000 | 295 | 5 | [316] | |||||
Bi nanotubes | Iodine-ion-assisted galvanic replacement | 1M NaPF6 in DME | AM:carbon black:CMC (90:5:5) | 69.2 | 355 | 20 | 15,000 | 319 | 150 | [204] | ||||||
2D | Bi@N-doped carbon | Solvothermal and carbonization | 1M NaPF6 in DME | AM:carbon black:PVDF (80:10:10) | 85.1 | ~325 | 10 | 5,000 | 341.5 | 10 | [223] | |||||
3D | Porous Bi/C | Annealing | 1M NaPF6 in DME | AM:carbon black: sodium alginate (70:15:15) | 95.2 | 178 | 50 | 20,000 | 101 | 72 | [317] | |||||
LC-bi composite | Calcination | 1M NaPF6 in DME | AM:super P:PVDF (80:10:10) | 53.4 | 225.6 | 1 | 2,600 | 236.1 | 100 | [201] | ||||||
3D porous Bi | Solution reduction | 1M NaPF6 in DME | AM:acetylene black:PVDF (70:20:10) | 65.9 | 374 | 10 | 3,000 | 354 | 60 | [318] | ||||||
Bulk Bi | Commercial | 1M NaPF6 in diglyme | AM:super P:PVDF (80:10:10) | 94.8 | 389 | 0.4 | 2,000 | 356 | 2 | [319] |
SUMMARY AND FUTURE PROSPECTIVE
Recent trends in synthetic methodologies for robust structural design, material selection, and analysis tools focusing on intermediate evolutions during (de)sodiation were highlighted in detail from a capacity-stability aspect for SIB alloying anode materials. Moreover, challenges faced by the current generation of alloying materials in terms of mechano-electrochemical changes incurred during cycling and strategies diversely employed to overcome those challenges were summarized. Detailed remediation strategies diversely employed to overcome the limitations were also highlighted. Conclusively, more focus on the latest mechanistic-driven characterization tools is obligatory for the upcoming high energy-density SIBs based on alloying anodes.
To ascertain capabilities of alloying anodes for next-generation SIBs, many modifications are necessary. Optimized alloying anode’s structure, composition, matching cathodes, binders, and electrolyte systems need extensive sorting. Structural modification is a prerequisite to accommodate some alloying anodes' volume changes, voltage hysteresis, and low electronic conductivity. However, effective modulation from the commercial perspective may take years to see SIBs on the energy horizon. Without deep tunneling into mechanistic paths and limitations, it seems unlikely.
Nanosized composite/alloy hybrid design with porous 3D electronically conductive materials must focus on alloy compositions for highly stable electrode configurations. Similarly, many recent studies have extensively validated the superiority of ether-based solvent systems as they offer stable and low energy solvation structuring with Na+, superior wettability, electrolyte penetration, and fast and reversible (de)sedation kinetics, ensuring thin and stable SEI. These ether-based electrolyte systems should be widely explored for various SIB alloying anodes along with recent approaches using binder-free and anodeless configurations and shifting towards solid electrolytes with potential to direct the future of alloying anodes.
Diverse formulations and materials choices in alloying SIB anodes have further been diversified with nanostructuring, doping, vacancy creation, alloying, and compositing with multicomponents, enhancing possibilities and prospects for high-stability electrode materials. In this regard, hard carbon materials with already proven promise as SIB anodes need more attention when compositing with alloying materials. A straightforward approach to optimize and validate an alloying-based electrode's performance from the bulk of theoretical and experimental literature reports is to use machine learning tools and theoretical models to obtain more efficient methodologies and material combinations, thereby reducing weary efforts in random material/methodology selection.
Other than morphology, material selection, and electrolyte systems, many other recently introduced strategies such as ion incorporation including (K+), sacrificial cathode additives, and presodiation of anodes have a good impact on capacity enhancement. However, current SIBs have much less energy density than prevailing LIBs. Most of the capacity destructive processes arise in the initial cycle, causing a low ICE, particularly in the case with alloying SIB anodes. For alloying anodes to ensure high ICE and stable long-term performance, remodeling of different constituents, especially the electrolyte system and its compatibility with the nanostructured alloying material, needs more focus. In this regard, the formation process of SEI needs to be closely observed to ensure thin, stable, non-destructive SEI that can further assist in fast kinetics.
Although promising results of alloying SIB anodes have been reported, giving some glimpse into commercial-scale achievement, they are usually limited to lab-scale breakthroughs. To sort the right combination of alloying materials and other constituents, the fundamental aspect of capacity fading must first be sorted. Notably, a complete mechanistic understanding of phases evolved during (de)sodiation needs operando characterization tools that can trace the formation and reconversion of species during continuous cycling. Unfortunately, most reports lack this vital information. The multistep alloying and alloying-conversion mechanism must be fully tracked using different operando tools. Mainly, in-situ TEM analysis is capable of giving atomistic details using HAADF-STEM (with corresponding SAED and FFT patterns) and EDS mapping that can ensure intermediate species while the charging-discharging proceeds. However, the current level of in-situ TEM has certain limitations, such as using the Na2O electrolyte in a nano-battery setup, which could not match or withstand the same as real-world batteries. Moreover, in-situ TEM of the nano-battery could not offer evidence of SEI formation. Nonetheless, coupling these in-situ findings with other in-situ and theoretical studies can decipher the mechanism involved in (de)sodiation, capacity fading, and these anode materials to a greater extent. In this regard, the continually varying interphase behavior can be tracked using in-situ XRD under (dis)charging conditions. Ex-situ XRD,
DECLARATIONS
Authors’ contributions
Writing-original draft: Rehman Au, Saleem S
Data curation: Ali S
Investigation: Rehman Au, Abbas SM, Choi M
Visualization: Choi M
Project administration: Choi W
Availability of data and materials
Not applicable.
Financial support and sponsorship
This research was supported by the program through the National Research Foundation of Korea (NRF) funded by the Ministry of Science and ICT (No. RS-2024-00404414, Development of High Energy Density Core Materials and High-Efficiency Cell Technology for 220Wh/kg-Class Sodium-Ion Batteries). Additional support was provided by the Korea Institute for Advancement of Technology (KIAT) grant funded by the Korean Government (MOTIE) (P0012748, HRD Program for Industrial Innovation).
Conflicts of interest
All authors declared that there are no conflicts of interest
Ethical approval and consent to participate
Not applicable.
Consent for publication
Not applicable.
Copyright
© The Author(s) 2024.
REFERENCES
1. Berry C. The paradox of green growth: challenges and opportunities in decarbonizing the lithium-ion supply chain. In: Kalantzakos S, editor. Critical minerals, the climate crisis and the tech imperium. Cham: Springer Nature Switzerland; 2023. pp. 107-23.
2. Uslu S, Pirinççi F. The impact of COVID-19 on global energy security and energy geopolitics. In: Akıllı E, Gunes B, editors. World politics in the age of uncertainty. Cham: Springer Nature Switzerland; 2023. pp. 219-33.
3. Singh AN, Islam M, Meena A, et al. Unleashing the potential of sodium-ion batteries: current state and future directions for sustainable energy storage. Adv Funct Mater 2023;33:2304617.
4. Zhou G, Chen H, Cui Y. Formulating energy density for designing practical lithium-sulfur batteries. Nat Energy 2022;7:312-9.
5. Tian H, Li Z, Feng G, et al. Stable, high-performance, dendrite-free, seawater-based aqueous batteries. Nat Commun 2021;12:237.
6. Wang X, Tang S, Guo W, Fu Y, Manthiram A. Advances in multimetallic alloy-based anodes for alkali-ion and alkali-metal batteries. Mater Today 2021;50:259-75.
7. Wang X, Zhang C, Sawczyk M, et al. Ultra-stable all-solid-state sodium metal batteries enabled by perfluoropolyether-based electrolytes. Nat Mater 2022;21:1057-65.
8. Sun Q, Dai L, Luo T, Wang L, Liang F, Liu S. Recent advances in solid-state metal-air batteries. Carbon Energy 2023;5:e276.
9. Zhang L, Feng R, Wang W, Yu G. Emerging chemistries and molecular designs for flow batteries. Nat Rev Chem 2022;6:524-43.
10. Olabi AG, Abbas Q, Al Makky A, Abdelkareem MA. Supercapacitors as next generation energy storage devices: properties and applications. Energy 2022;248:123617.
12. Dutta A, Mitra S, Basak M, Banerjee T. A comprehensive review on batteries and supercapacitors: development and challenges since their inception. Energy Stor 2023;5:e339.
13. Hao H, Hutter T, Boyce BL, Watt J, Liu P, Mitlin D. Review of multifunctional separators: stabilizing the cathode and the anode for alkali (Li, Na, and K) metal-sulfur and selenium batteries. Chem Rev 2022;122:8053-125.
14. Huang L, Lu T, Xu G, et al. Thermal runaway routes of large-format lithium-sulfur pouch cell batteries. Joule 2022;6:906-22.
15. Hong X, Mei J, Wen L, et al. Nonlithium metal-sulfur batteries: steps toward a leap. Adv Mater 2019;31:e1802822.
16. Yabuuchi N, Kubota K, Dahbi M, Komaba S. Research development on sodium-ion batteries. Chem Rev 2014;114:11636-82.
17. Tan H, Chen D, Rui X, Yu Y. Peering into alloy anodes for sodium-ion batteries: current trends, challenges, and opportunities. Adv Funct Mater 2019;29:1808745.
18. Sadik-zada ER, Gatto A, Scharfenstein M. Sustainable management of lithium and green hydrogen and long-run perspectives of electromobility. Technol Forecast Soc Change 2023;186:121992.
19. Li X, Sengupta T, Si Mohammed K, Jamaani F. Forecasting the lithium mineral resources prices in China: evidence with facebook prophet (Fb-P) and artificial neural networks (ANN) methods. Resour Policy 2023;82:103580.
20. Frith JT, Lacey MJ, Ulissi U. A non-academic perspective on the future of lithium-based batteries. Nat Commun 2023;14:420.
21. Vaalma C, Buchholz D, Weil M, Passerini S. A cost and resource analysis of sodium-ion batteries. Nat Rev Mater 2018;3:1-11.
22. Nayak PK, Yang L, Brehm W, Adelhelm P. From lithium-ion to sodium-ion batteries: advantages, challenges, and surprises. Angew Chem Int Ed 2018;57:102-20.
23. Zhao L, Hu Z, Lai W, et al. Hard carbon anodes: fundamental understanding and commercial perspectives for Na-ion batteries beyond Li-ion and K-ion counterparts. Adv Energy Mater 2021;11:2002704.
24. Song K, Liu C, Mi L, Chou S, Chen W, Shen C. Recent progress on the alloy-based anode for sodium-ion batteries and potassium-ion batteries. Small 2021;17:e1903194.
25. Luo W, Shen F, Bommier C, Zhu H, Ji X, Hu L. Na-ion battery anodes: materials and electrochemistry. ACC Chem Res 2016;49:231-40.
26. He H, Sun D, Tang Y, Wang H, Shao M. Understanding and improving the initial Coulombic efficiency of high-capacity anode materials for practical sodium ion batteries. Energy Stor Mater 2019;23:233-51.
27. Patrike A, Yadav P, Shelke V, Shelke M. Research progress and perspective on lithium/sodium metal anodes for next-generation rechargeable batteries. ChemSusChem 2022;15:e202200504.
28. Chen J, Adit G, Li L, Zhang Y, Chua DHC, Lee PS. Optimization strategies toward functional sodium-ion batteries. Energy Environ Mater 2023;6:e12633.
29. Qiao S, Zhou Q, Ma M, Liu HK, Dou SX, Chong S. Advanced anode materials for rechargeable sodium-ion batteries. ACS Nano 2023;17:11220-52.
30. Sarkar S, Peter SC. An overview on Sb-based intermetallics and alloys for sodium-ion batteries: trends, challenges and future prospects from material synthesis to battery performance. J Mater Chem A 2021;9:5164-96.
31. Xu G, Amine R, Abouimrane A, et al. Challenges in developing electrodes, electrolytes, and diagnostics tools to understand and advance sodium-ion batteries. Adv Energy Mater 2018;8:1702403.
32. Hou Z, Lei D, Jiang M, et al. Biomass-derived hard carbon with interlayer spacing optimization toward ultrastable Na-ion storage. ACS Appl Mater Interfaces 2023;15:1367-75.
33. Yang G, Ilango PR, Wang S, et al. Carbon-based alloy-type composite anode materials toward sodium-ion batteries. Small 2019;15:e1900628.
34. Wang W, Wang B, Li Y, et al. Hard carbon derived from different precursors for sodium storage. Chem Asian J 2024;19:e202301146.
35. Veerasubramani GK, Park M, Nakate UT, et al. Intrinsically nitrogen-enriched biomass-derived hard carbon with enhanced performance as a sodium-ion battery anode. Energy Fuels 2024;38:7368-78.
36. Tang Y, He J, Peng J, et al. Electrochemical behavior of the biomass hard carbon derived from waste corncob as a sodium-ion battery anode. Energy Fuels 2024;38:7389-98.
37. Zhang G, Chen C, Xu C, et al. Unraveling the microcrystalline carbon evolution mechanism of biomass-derived hard carbon for sodium-ion batteries. Energy Fuels 2024;38:8326-36.
38. Molaiyan P, Dos Reis GS, Karuppiah D, Subramaniyam CM, García-alvarado F, Lassi U. Recent progress in biomass-derived carbon materials for Li-ion and Na-ion batteries - a review. Batteries 2023;9:116.
39. Hu H, Xiao Y, Ling W, et al. A stable biomass-derived hard carbon anode for high-performance sodium-ion full battery. Energy Tech 2021;9:2000730.
40. Li N, Wang Y, Liu L, et al. “Self-doping” defect engineering in SnP3@gamma-irradiated hard carbon anode for rechargeable sodium storage. J Colloid Interface Sci 2021;592:279-90.
41. Fang L, Bahlawane N, Sun W, et al. Conversion-alloying anode materials for sodium ion batteries. Small 2021;17:e2101137.
42. Li X, Guo Y, Hu Z, et al. Improving the initial coulombic efficiency of sodium-storage antimony anodes via electrochemically alloying bismuth. ACS Appl Mater Interfaces 2023;15:45926-37.
43. Zhang H, Hasa I, Passerini S. Beyond insertion for Na-ion batteries: nanostructured alloying and conversion anode materials. Adv Energy Mater 2018;8:1702582.
44. Zhao S, Guo Z, Yang J, Wang C, Sun B, Wang G. Nanoengineering of advanced carbon materials for sodium-ion batteries. Small 2021;17:e2007431.
45. Lu X, Adkins ER, He Y, et al. Germanium as a sodium ion battery material: in situ TEM reveals fast sodiation kinetics with high capacity. Chem Mater 2016;28:1236-42.
46. Chen Y, Li F, Guo Z, et al. Sustainable and scalable fabrication of high-performance hard carbon anode for Na-ion battery. J Power Sources 2023;557:232534.
47. Tang Z, Zhang R, Wang H, et al. Revealing the closed pore formation of waste wood-derived hard carbon for advanced sodium-ion battery. Nat Commun 2023;14:6024.
48. Perveen T, Siddiq M, Shahzad N, Ihsan R, Ahmad A, Shahzad MI. Prospects in anode materials for sodium ion batteries - a review. Renew Sust Energy Rev 2020;119:109549.
49. Xiao B, Rojo T, Li X. Hard carbon as sodium-ion battery anodes: progress and challenges. ChemSusChem 2019;12:133-44.
50. Fang S, Bresser D, Passerini S. Transition metal oxide anodes for electrochemical energy storage in lithium- and sodium-ion batteries*. In: Nanda J, Augustyn V, editors. Transition metal oxides for electrochemical energy storage. Wiley; 2022. pp. 55-99.
51. Lim YV, Li XL, Yang HY. Recent tactics and advances in the application of metal sulfides as high-performance anode materials for rechargeable sodium-ion batteries. Adv Funct Mater 2021;31:2006761.
52. Hao Z, Shi X, Yang Z, Li L, Chou S. Developing high-performance metal selenides for sodium-ion batteries. Adv Funct Mater 2022;32:2208093.
53. Fan H, Mao P, Sun H, et al. Recent advances of metal telluride anodes for high-performance lithium/sodium-ion batteries. Mater Horiz 2022;9:524-46.
54. Zhang W, Liu T, Wang Y, et al. Strategies to improve the performance of phosphide anodes in sodium-ion batteries. Nano Energy 2021;90:106475.
55. Li G, Guo S, Xiang B, et al. Recent advances and perspectives of microsized alloying-type porous anode materials in high-performance Li- and Na-ion batteries. Energy Mater 2022;2:200020.
56. Shao R, Sun Z, Wang L, et al. Resolving the origins of superior cycling performance of antimony anode in sodium-ion batteries: a comparison with lithium-ion batteries. Angew Chem Int Ed 2024;136:e202320183.
57. Chen Z, Wu X, Sun Z, et al. Enhanced fast-charging and longevity in sodium-ion batteries through nitrogen-doped carbon frameworks encasing flower-like bismuth microspheres. Adv Energy Mater 2024;14:2400132.
58. Yao Q, Zheng C, Liu K, et al. Bi nanospheres embedded in N-doped carbon nanowires facilitate ultrafast and ultrastable sodium storage. Adv Sci 2024;11:e2401730.
59. Li W, Ke L, Wei Y, et al. Highly reversible sodium storage in a GeP5/C composite anode with large capacity and low voltage. J Mater Chem A 2017;5:4413-20.
60. Li X, Qu J, Zhao Y, Lai Q, Wang P, Yi T. Reaction mechanisms, recent progress and future prospects of tin selenide-based composites for alkali-metal-ion batteries. Compos Part B Eng 2022;242:110045.
61. Wu X, Lan X, Hu R, Yao Y, Yu Y, Zhu M. Tin-based anode materials for stable sodium storage: progress and perspective. Adv Mater 2022;34:e2106895.
62. Zheng C, Yao Q, Li R, et al. Construction of robust solid-electrolyte interphase via electrode additive for high-performance Sn-based anodes of sodium-ion batteries. Energy Stor Mater 2024;67:103334.
63. Huang J, Guo X, Du X, et al. Nanostructures of solid electrolyte interphases and their consequences for microsized Sn anodes in sodium ion batteries. Energy Environ Sci 2019;12:1550-7.
64. Duan YK, Li ZW, Zhang SC, et al. Stannate-based materials as anodes in lithium-ion and sodium-ion batteries: a review. Molecules 2023;28:5037.
65. Tian Z, Zou Y, Liu G, et al. Electrolyte solvation structure design for sodium ion batteries. Adv Sci 2022;9:e2201207.
66. Huang Y, Zhao L, Li L, Xie M, Wu F, Chen R. Electrolytes and electrolyte/electrode interfaces in sodium-ion batteries: from scientific research to practical application. Adv Mater 2019;31:e1808393.
67. Mou H, Xiao W, Miao C, Li R, Yu L. Tin and tin compound materials as anodes in lithium-ion and sodium-ion batteries: a review. Front Chem 2020;8:141.
68. Liang J, Zhang L, Xili D, Kang J. Research progress on tin-based anode materials for sodium ion batteries. Rare Met 2020;39:1005-18.
69. Sadan MK, Kim H, Kim C, et al. Ultra-long cycle life of flexible Sn anode using DME electrolyte. J Alloys Compd 2021;871:159549.
70. Daali A, Zhou X, Zhao C, et al. In situ microscopy and spectroscopy characterization of microsized Sn anode for sodium-ion batteries. Nano Energy 2023;115:108753.
71. Zheng C, Ji D, Yao Q, et al. Electrostatic shielding boosts electrochemical performance of alloy-type anode materials of sodium-ion batteries. Angew Chem Int Ed 2023;62:e202214258.
72. Yao Q, Zhu Y, Zheng C, et al. Intermolecular cross-linking reinforces polymer binders for durable alloy-type anode materials of sodium-ion batteries. Adv Energy Mater 2023;13:2202939.
73. Shen H, An Y, Man Q, et al. Chemical prelithiation/presodiation strategies toward controllable and scalable synthesis of microsized nanoporous tin at room temperature for high-energy sodium-ion batteries. Adv Funct Mater 2024;34:2309834.
74. Ying H, Han WQ. Metallic Sn-based anode materials: application in high-performance lithium-ion and sodium-ion batteries. Adv Sci 2017;4:1700298.
75. Yang J, Guo X, Gao H, et al. A high-performance alloy-based anode enabled by surface and interface engineering for wide-temperature sodium-ion batteries. Adv Energy Mater 2023;13:2300351.
76. Zhang S, Yue L, Wang M, Feng Y, Li Z, Mi J. SnO2 nanoparticles confined by N-doped and CNTs-modified carbon fibers as superior anode material for sodium-ion battery. Solid State Ionics 2018;323:105-11.
77. Ma D, Li Y, Mi H, et al. Robust SnO2-x nanoparticle-impregnated carbon nanofibers with outstanding electrochemical performance for advanced sodium-ion batteries. Angew Chem Int Ed 2018;57:8901-5.
78. Fan L, Song X, Xiong D, Li X. Nitrogen-doping of graphene enhancing sodium storage of SnO2 anode. J Electroanal Chem 2019;833:340-8.
79. Wang Y, Jin Y, Zhao C, Pan E, Jia M. 1D ultrafine SnO2 nanorods anchored on 3D graphene aerogels with hierarchical porous structures for high-performance lithium/sodium storage. J Colloid Interface Sci 2018;532:352-62.
80. Demir E, Aydin M, Arie AA, Demir-cakan R. Apricot shell derived hard carbons and their tin oxide composites as anode materials for sodium-ion batteries. J Alloys Compd 2019;788:1093-102.
81. Choi IY, Jo C, Lim WG, et al. Amorphous Tin oxide nanohelix structure based electrode for highly reversible Na-ion batteries. ACS Nano 2019;13:6513-21.
82. Han B, Zhang W, Gao D, et al. Encapsulating tin oxide nanoparticles into holey carbon nanotubes by melt infiltration for superior lithium and sodium ion storage. J Power Sources 2020;449:227564.
83. Narsimulu D, Nagaraju G, Chandra Sekhar S, Ramulu B, Su Yu J. Three-dimensional porous SnO2/carbon cloth electrodes for high-performance lithium- and sodium-ion batteries. Appl Surf Sci 2021;538:148033.
84. Chen Y, Sun Y, Geng M, et al. SnO2/MXene nanoparticles as a superior high-rate and cycling-stable anode for sodium ion batteries. Mater Lett 2021;304:130704.
85. Wu YQ, Yang HX, Yang Y, et al. SnS2/Co3S4 hollow nanocubes anchored on S-doped graphene for ultrafast and stable Na-ion storage. Small 2019;15:e1903873.
86. He X, Liu J, Kang B, et al. Preparation of SnS2/enteromorpha prolifera derived carbon composite and its performance of sodium-ion batteries. J Phys Chem Solids 2021;152:109976.
87. Ding J, Tang C, Zhu G, et al. Integrating SnS2 quantum dots with nitrogen-doped Ti3C2Tx MXene nanosheets for robust sodium storage performance. ACS Appl Energy Mater 2021;4:846-54.
88. Jiang Y, Liu G, Lu S, et al. A novel interlayer-expanded tin disulfide/reduced graphene oxide nanocomposite as anode material for high-performance sodium-ion batteries. J Colloid Interface Sci 2022;611:215-23.
89. Li Z, Zheng J, Xiao M, et al. Three-dimensional 1T-SnS2 wrapped with graphene for sodium-ion battery anodes with highly reversible sodiation/desodiation. Scr Mater 2022;211:114500.
90. Li Q, Yu F, Cui Y, Wang J, Zhao Y, Peng J. Multilayer SnS-SnS2@GO heterostructures nanosheet as anode material for Sodium ion battery with high capacity and stability. J Alloys Compd 2023;937:168392.
91. Yang X, Miao Z, Zhong Q, et al. ZnS/SnS2 heterostructures encapsulated in N-doped carbon nanofibers for high-performance alkali metal-ion batteries. ACS Appl Mater Interfaces 2023;15:46881-94.
92. Huang S, Wang M, Jia P, Wang B, Zhang J, Zhao Y. N-graphene motivated SnO2@SnS2 heterostructure quantum dots for high performance lithium/sodium storage. Energy Stor Mater 2019;20:225-33.
93. Ou X, Cao L, Liang X, et al. Fabrication of SnS2/Mn2SnS4/Carbon heterostructures for sodium-ion batteries with high initial coulombic efficiency and cycling stability. ACS Nano 2019;13:3666-76.
94. Zhang F, Shen Y, Shao M, et al. SnSe2 nanoparticles chemically embedded in a carbon shell for high-rate sodium-ion storage. ACS Appl Mater Interfaces 2020;12:2346-53.
95. Yang W, Chen Y, Yin X, Lai X, Wang J, Jian J. SnSe nanosheet array on carbon cloth as a high-capacity anode for sodium-ion batteries. ACS Appl Mater Interfaces 2023;15:42811-22.
96. Liu P, Han J, Zhu K, Dong Z, Jiao L. Heterostructure SnSe2/ZnSe@PDA nanobox for stable and highly efficient sodium-ion storage. Adv Energy Mater 2020;10:2000741.
97. Fan T, Wu Y, Li J, et al. Sheet-to-layer structure of SnSe2/MXene composite materials for advanced sodium ion battery anodes. New J Chem 2021;45:1944-52.
98. Wang W, Hu L, Li L, et al. Constructing a rapid ion and electron migration channels in MoSe2/SnSe2@C 2D heterostructures for high-efficiency sodium-ion half/full batteries. Electrochim Acta 2023;449:142239.
99. Kong Z, Liang Z, Huang M, et al. Yolk-shell tin phosphides composites as superior reversibility and stability anodes for lithium/sodium ion batteries. J Alloys Compd 2023;930:167328.
100. Liu C, Yang X, Liu J, Ye X. Theoretical prediction of two-dimensional SnP3 as a promising anode material for Na-ion batteries. ACS Appl Energy Mater 2018;1:3850-9.
101. Kong Z, Yao X, Shao Y, et al. SnxPy nanoplate/reduced graphene oxide composites as anode materials for lithium-/sodium-ion batteries. ACS Appl Nano Mater 2021;4:12335-45.
102. Pan E, Jin Y, Zhao C, et al. Mesoporous Sn4P3-graphene aerogel composite as a high-performance anode in sodium ion batteries. Appl Surf Sci 2019;475:12-9.
103. Pan E, Jin Y, Zhao C, et al. Conformal hollow carbon sphere coated on Sn4P3 microspheres as high-rate and cycle-stable anode materials with superior sodium storage capability. ACS Appl Energy Mater 2019;2:1756-64.
104. Zhang J, Wang W, Li B. Enabling high sodium storage performance of micron-sized Sn4P3 anode via diglyme-derived solid electrolyte interphase. Chem Eng J 2020;392:123810.
105. Ran L, Luo B, Gentle IR, et al. Biomimetic Sn4P3 anchored on carbon nanotubes as an anode for high-performance sodium-ion batteries. ACS Nano 2020;14:8826-37.
106. Fan W, Gao Y, Hui Q, et al. A closed-ended MXene armor on hollow Sn4P3 nanospheres for ultrahigh-rate and stable sodium storage. Chem Eng J 2023;465:142963.
107. Fan W, Gao Y, Liu H, Xia X. Rational design of conductive MXenes-based networks by Sn and Sn4P3 nanoparticles for durable sodium-ion battery. J Power Sources 2023;562:232750.
108. Baggetto L, Ganesh P, Sun C, Meisner RA, Zawodzinski TA, Veith GM. Intrinsic thermodynamic and kinetic properties of Sb electrodes for Li-ion and Na-ion batteries: experiment and theory. J Mater Chem A 2013;1:7985-94.
109. Liu Y, Zhou B, Liu S, Ma Q, Zhang WH. Galvanic replacement synthesis of highly uniform sb nanotubes: reaction mechanism and enhanced sodium storage performance. ACS Nano 2019;13:5885-92.
110. Qian J, Chen Y, Wu L, Cao Y, Ai X, Yang H. High capacity Na-storage and superior cyclability of nanocomposite Sb/C anode for Na-ion batteries. Chem Commun 2012;48:7070-2.
111. Xu X, Dou Z, Gu E, Si L, Zhou X, Bao J. Uniformly-distributed Sb nanoparticles in ionic liquid-derived nitrogen-enriched carbon for highly reversible sodium storage. J Mater Chem A 2017;5:13411-20.
112. Wu C, Shen L, Chen S, et al. Top-down synthesis of interconnected two-dimensional carbon/antimony hybrids as advanced anodes for sodium storage. Energy Stor Mater 2018;10:122-9.
113. Kong B, Zu L, Peng C, et al. Direct superassemblies of freestanding metal-carbon frameworks featuring reversible crystalline-phase transformation for electrochemical sodium storage. J Am Chem Soc 2016;138:16533-41.
114. Park J, Kim M, Choi M, et al. Sb/C composite embedded in SiOC buffer matrix via dispersion property control for novel anode material in sodium-ion batteries. J Power Sources 2023;568:232908.
115. Liu Y, Qing Y, Zhou B, et al. Yolk-shell Sb@Void@Graphdiyne nanoboxes for high-rate and long cycle life sodium-ion batteries. ACS Nano 2023;17:2431-9.
116. Nieto K, Windsor DS, Kale AR, Gallawa JR, Medina DA, Prieto AL. Structural control of electrodeposited sb anodes through solution additives and their influence on electrochemical performance in Na-ion batteries. J Phys Chem C 2023;127:12415-27.
117. Zheng X, You J, Fan J, et al. Electrodeposited binder-free Sb/NiSb anode of sodium-ion batteries with excellent cycle stability and rate capability and new insights into its reaction mechanism by operando XRD analysis. Nano Energy 2020;77:105123.
118. Baggetto L, Allcorn E, Unocic RR, Manthiram A, Veith GM. Mo3Sb7 as a very fast anode material for lithium-ion and sodium-ion batteries. J Mater Chem A 2013;1:11163-9.
119. Song Z, Wang G, Chen Y, Lu Y, Wen Z. In situ three-dimensional cross-linked carbon nanotube-interspersed SnSb@CNF as freestanding anode for long-term cycling sodium-ion batteries. Chem Eng J 2023;463:142289.
120. Shen H, Zheng X, Kang Q, et al. High-performance and sodiation mechanism of a pulse potential-electrodeposited Sb-Zn alloy as an anode for sodium-ion batteries. Appl Surf Sci 2023;609:155243.
121. Chen B, Liang M, Wu Q, Zhu S, Zhao N, He C. Recent developments of antimony-based anodes for sodium- and potassium-ion batteries. Trans Tianjin Univ 2022;28:6-32.
122. Zhou X, Deng H, Wang A, Song J, Lei Z, Xu Y. Antimony oxides-based anode materials for alkali metal-ion storage. Chemistry 2023;29:e202300506.
123. Deng M, Li S, Hong W, et al. Octahedral Sb2O3 as high-performance anode for lithium and sodium storage. Mater Chem Phys 2019;223:46-52.
124. Kim S, Qu S, Zhang R, Braun PV. High volumetric and gravimetric capacity electrodeposited mesostructured Sb2O3 sodium ion battery anodes. Small 2019;15:e1900258.
125. Ma W, Wang J, Gao H, et al. A mesoporous antimony-based nanocomposite for advanced sodium ion batteries. Energy Stor Mater 2018;13:247-56.
126. Li D, Li J, Cao J, Fu X, Zhou L, Han W. Highly flexible free-standing Sb/Sb2O3 @N-doped carbon nanofiber membranes for sodium ion batteries with excellent stability. Sustain Energy Fuels 2020;4:5732-8.
127. Ye J, Xia G, Zheng Z, Hu C. Facile controlled synthesis of coral-like nanostructured Sb2O3@Sb anode materials for high performance sodium-ion batteries. Int J Hydrogen Energy 2020;45:9969-78.
128. Liao S, Wang X, Hu H, Chen D, Zhang M, Luo J. Carbon-encapsulated Sb6O13 nanoparticles for an efficient and durable sodium-ion battery anode. J Alloys Compd 2021;852:156939.
129. Subramanyan K, Palmurukan MR, Lee Y, Aravindan V. Exfoliated graphene oxide@ Sb2O3 octahedrons as alloy-conversion anode for high-performance Na-ion batteries with P2-type Na2/3Ni1/3Mn2/3O2 cathode. Electrochim Acta 2023;470:143308.
130. Lakshmi K, Deivanayagam R, Shaijumon M. Carbon nanotube ‘wired’ octahedral Sb2O3/graphene aerogel as efficient anode material for sodium and lithium ion batteries. J Alloys Compd 2021;857:158267.
131. Deng M, Li S, Hong W, et al. Natural stibnite ore (Sb2S3) embedded in sulfur-doped carbon sheets: enhanced electrochemical properties as anode for sodium ions storage. RSC Adv 2019;9:15210-6.
132. Xie J, Xia J, Yuan Y, et al. Sb2S3 embedded in carbon-silicon oxide nanofibers as high-performance anode materials for lithium-ion and sodium-ion batteries. J Power Sources 2019;435:226762.
133. Huang Y, Wang Z, Jiang Y, et al. Conductivity and pseudocapacitance optimization of bimetallic antimony-indium sulfide anodes for sodium-ion batteries with favorable kinetics. Adv Sci 2018;5:1800613.
134. Cao L, Gao X, Zhang B, Ou X, Zhang J, Luo WB. Bimetallic sulfide Sb2S3@FeS2 hollow nanorods as high-performance anode materials for sodium-ion batteries. ACS Nano 2020;14:3610-20.
135. Lin J, Yao L, Zhang C, et al. Construction of Sb2S3@SnS@C tubular heterostructures as high-performance anode materials for sodium-ion batteries. ACS Sustain Chem Eng 2021;9:11280-9.
136. Zhang H, Ren M, Jiang W, Yao J, Pan L, Yang J. Hierarchical Sb2S3@m-Ti3C2Tx composite anode with enhanced Na-ion storage properties. J Alloys Compd 2021;887:161318.
137. Li D, Li J, Liu H, et al. Ti3C2Tx constrained Sb2S3 composite biomass-derived carbon ribbon film achieves stable sodium storage for flexible quasi-solid full-battery. Chem Eng J 2023;477:147045.
138. Zhu M, Li J, Yang X, Li X, Wang L, Lü W. 3D reduced graphene oxide wrapped MoS2@Sb2S3 heterostructures for high performance sodium-ion batteries. Appl Surf Sci 2023;624:157106.
139. Ou X, Yang C, Xiong X, et al. A new rGO-overcoated Sb2Se3 nanorods anode for Na+ battery: in situ X-ray diffraction study on a live sodiation/desodiation process. Adv Funct Mater 2017;27:1606242.
140. Li J, Zhang W, Zheng W. Metal selenides find plenty of space in architecting advanced sodium/potassium ion batteries. Small 2024;20:e2305021.
141. Nam K, Park C. 2D layered Sb2Se3-based amorphous composite for high-performance Li- and Na-ion battery anodes. J Power Sources 2019;433:126639.
142. Wang Y, Cao D, Zhang K, et al. Cation-exchange construction of ZnSe/Sb2Se3 hollow microspheres coated by nitrogen-doped carbon with enhanced sodium ion storage capability. Nanoscale 2020;12:17915-24.
143. Ihsan-ul-haq M, Huang H, Wu J, et al. Unveiling solid electrolyte interface morphology and electrochemical kinetics of amorphous Sb2Se3/CNT composite anodes for ultrafast sodium storage. Carbon 2021;171:119-29.
144. Hu L, Pan J, Zhao P, Shi G, Wang B, Huang F. A new method of synthesis of Sb2Se3/rGO as a high-rate and low-temperature anode for sodium-ion batteries. Mater Adv 2022;3:3554-61.
145. Chong S, Ma M, Yuan L, et al. Hierarchical encapsulation and rich sp2N assist Sb2Se3-based conversion-alloying anode for long-life sodium- and potassium-ion storage. Energy Environ Mater 2023;6:e12458.
146. Yang J, Li J, Lu J, et al. Synergistically boosting reaction kinetics and suppressing polyselenide shuttle effect by Ti3C2Tx/Sb2Se3 film anode in high-performance sodium-ion batteries. J Colloid Interf Sci 2023;649:234-44.
147. Wu Y, Luo W, Gao P, et al. Unveiling the microscopic origin of asymmetric phase transformations in (de)sodiated Sb2Se3 with in situ transmission electron microscopy. Nano Energy 2020;77:105299.
148. Wang Y, Niu P, Li J, Wang S, Li L. Recent progress of phosphorus composite anodes for sodium/potassium ion batteries. Energy Stor Mater 2021;34:436-60.
149. Dong S, Wang L, Huang X, Liang J, He X. Challenges and prospects of phosphorus-based anode materials for secondary batteries. Batteries Supercaps 2023;6:e202300265.
150. Liu S, Xu H, Bian X, et al. Nanoporous red phosphorus on reduced graphene oxide as superior anode for sodium-ion batteries. ACS Nano 2018;12:7380-7.
151. Hu Y, Li B, Jiao X, Zhang C, Dai X, Song J. Stable cycling of phosphorus anode for sodium-ion batteries through chemical bonding with sulfurized polyacrylonitrile. Adv Funct Mater 2018;28:1801010.
152. Capone I, Hurlbutt K, Naylor AJ, Xiao AW, Pasta M. Effect of the particle-size distribution on the electrochemical performance of a red phosphorus-carbon composite anode for sodium-ion batteries. Energy Fuels 2019;33:4651-8.
153. Xiao W, Sun Q, Banis MN, et al. Unveiling the interfacial instability of the phosphorus/carbon anode for sodium-ion batteries. ACS Appl Mater Interf 2019;11:30763-73.
154. Liu W, Ju S, Yu X. Phosphorus-amine-based synthesis of nanoscale red phosphorus for application to sodium-ion batteries. ACS Nano 2020;14:974-84.
155. Fang K, Liu D, Xiang X, et al. Air-stable red phosphorus anode for potassium/sodium-ion batteries enabled through dual-protection design. Nano Energy 2020;69:104451.
156. Jin H, Lu H, Wu W, et al. Tailoring conductive networks within hollow carbon nanospheres to host phosphorus for advanced sodium ion batteries. Nano Energy 2020;70:104569.
157. Liu Y, Liu Q, Jian C, et al. Red-phosphorus-impregnated carbon nanofibers for sodium-ion batteries and liquefaction of red phosphorus. Nat Commun 2020;11:2520.
158. Subramaniyam CM, Kang MA, Li J, VahidMohammadi A, Hamedi MM. Additive-free red phosphorus/Ti3C2Tx MXene nanocomposite anodes for metal-ion batteries. Energy Adv 2022;1:999-1008.
159. Zhu Z, Pei Z, Liu B, et al. Hierarchical ion/electron networks enable efficient red phosphorus anode with high mass loading for sodium ion batteries. Adv Funct Mater 2022;32:2110444.
160. Kaur H, Konkena B, Gabbett C, et al. Amorphous 2D-nanoplatelets of red phosphorus obtained by liquid-phase exfoliation yield high areal capacity Na-ion battery anodes. Adv Energy Mater 2023;13:2203013.
161. Li Z, Zhao H. Recent developments of phosphorus-based anodes for sodium ion batteries. J Mater Chem A 2018;6:24013-30.
162. Chang G, Zhao Y, Dong L, et al. A review of phosphorus and phosphides as anode materials for advanced sodium-ion batteries. J Mater Chem A 2020;8:4996-5048.
163. Zhou J, Shi Q, Ullah S, et al. Phosphorus-based composites as anode materials for advanced alkali metal ion batteries. Adv Funct Mater 2020;30:2004648.
164. Shen H, Han X, Zheng X, et al. One-step electrochemical synthesis and optimization of Sb-Co-P alloy anode for sodium ion battery. Electrochim Acta 2023;438:141529.
165. Zhang N, Chen X, Zhao J, He P, Ding X. Mass produced Sb/P@C composite nanospheres for advanced sodium-ions battery anodes. Electrochim Acta 2023;439:141602.
166. Jung SC, Jung DS, Choi JW, Han YK. Atom-level understanding of the sodiation process in silicon anode material. J Phys Chem Lett 2014;5:1283-8.
167. Liu C, Jiang Y, Meng C, Liu X, Li B, Xia S. Amorphous germanium nanomaterials as high-performance anode for lithium and sodium-ion batteries. Adv Mater Technol 2023;8:2201817.
168. Li M, Zhang Z, Ge X, et al. Enhanced electrochemical properties of carbon coated Zn2GeO4 micron-rods as anode materials for sodium-ion batteries. Chem Eng J 2018;331:203-10.
169. Tseng K, Huang S, Chang W, Tuan H. Synthesis of mesoporous germanium phosphide microspheres for high-performance lithium-ion and sodium-ion battery anodes. Chem Mater 2018;30:4440-7.
170. Shen H, Ma Z, Yang B, et al. Sodium storage mechanism and electrochemical performance of layered GeP as anode for sodium ion batteries. J Power Sources 2019;433:126682.
171. Li W, Li X, Liao J, et al. Structural design of Ge-based anodes with chemical bonding for high-performance Na-ion batteries. Energy Stor Mater 2019;20:380-7.
172. Sung G, Nam K, Choi J, Park C. Germanium telluride: layered high-performance anode for sodium-ion batteries. Electrochim Acta 2020;331:135393.
173. Wang C, Wang D, Ma X, et al. Isotropy-induced stress relaxation and strong-tolerance for high-rate and long-duration sodium storage by amorphous structure engineering. Adv Funct Mater 2022;32:2204687.
174. Yanilmaz M, Cihanbeyoğlu G, Kim J. Centrifugally spun binder-free n, s-doped Ge@PCNF anodes for Li-ion and Na-ion batteries. ACS Omega 2023;8:16987-95.
175. Li Y, Wu F, Li Y, et al. Multilevel gradient-ordered silicon anode with unprecedented sodium storage. Adv Mater 2024;36:e2310270.
176. Arrieta U, Katcho NA, Arcelus O, Carrasco J. First-principles study of sodium intercalation in crystalline NaxSi24 (0 ≤ x ≤ 4) as anode material for Na-ion batteries. Sci Rep 2017;7:5350.
177. Majid A, Hussain K, Ud-din Khan S, Ud-din Khan S. First principles study of SiC as the anode in sodium ion batteries. New J Chem 2020;44:8910-21.
178. Zhao Q, Huang Y, Hu X. A Si/C nanocomposite anode by ball milling for highly reversible sodium storage. Electrochem Commun 2016;70:8-12.
179. Han Y, Lin N, Xu T, et al. An amorphous Si material with a sponge-like structure as an anode for Li-ion and Na-ion batteries. Nanoscale 2018;10:3153-8.
180. Jangid MK, Vemulapally A, Sonia FJ, Aslam M, Mukhopadhyay A. Feasibility of reversible electrochemical Na-storage and cyclic stability of amorphous silicon and silicon-graphene film electrodes. J Electrochem Soc 2017;164:A2559-65.
181. Kempf A, Kiefer S, Graczyk-zajac M, Ionescu E, Riedel R. Tin-functionalized silicon oxycarbide as a stable, high-capacity anode material for Na-ion batteries. Open Ceram 2023;15:100388.
182. Zhang Y, Tang YC, Li XT, et al. Porous amorphous silicon hollow nanoboxes coated with reduced graphene oxide as stable anodes for sodium-ion batteries. ACS Omega 2022;7:30208-14.
183. Zeng L, Liu R, Han L, et al. Preparation of a Si/SiO2-ordered-mesoporous-carbon nanocomposite as an anode for high-performance lithium-ion and sodium-ion batteries. Chemistry 2018;24:4841-8.
184. Kalisvaart WP, Olsen BC, Luber EJ, Buriak JM. Sb-Si alloys and multilayers for sodium-ion battery anodes. ACS Appl Energy Mater 2019;2:2205-13.
185. Gong H, Du T, Liu L, et al. Self-source silicon embedded in 2D biomass-based carbon sheet as anode material for sodium ion battery. Appl Surf Sci 2022;586:152759.
186. Nazarian-samani M, Nazarian-samani M, Haghighat-shishavan S, Kim K. Predelithiation-driven ultrastable Na-ion battery performance using Si,P-rich ternary M-Si-P anodes. Energy Stor Mater 2022;49:421-32.
187. Din MA, Li C, Zhang L, Han C, Li B. Recent progress and challenges on the bismuth-based anode for sodium-ion batteries and potassium-ion batteries. Mater Today Phys 2021;21:100486.
188. Sun J, Li M, Oh JAS, Zeng K, Lu L. Recent advances of bismuth based anode materials for sodium-ion batteries. Mater Technol 2018;33:563-73.
189. Park B, Lee S, Han D, et al. Multiscale hierarchical design of bismuth-carbon anodes for ultrafast-charging sodium-ion full battery. Appl Surf Sci 2023;614:156188.
190. Hu C, Zhu Y, Ma G, et al. Sandwich-structured dual carbon modified bismuth nanosphere composites as long-cycle and high-rate anode materials for sodium-ion batteries. Electrochim Acta 2021;365:137379.
191. Xue P, Wang N, Fang Z, et al. Rayleigh-instability-induced bismuth nanorod@nitrogen-doped carbon nanotubes as a long cycling and high rate anode for sodium-ion batteries. Nano Lett 2019;19:1998-2004.
192. Yin H, Cao M, Yu X, et al. Self-standing Bi2O3 nanoparticles/carbon nanofiber hybrid films as a binder-free anode for flexible sodium-ion batteries. Mater Chem Front 2017;1:1615-21.
193. Liu R, Yu L, He X, et al. Constructing heterointerface of Bi/Bi2S3 with built-in electric field realizes superior sodium-ion storage capability. eScience 2023;3:100138.
194. Lin J, Lu S, Zhang Y, Zeng L, Zhang Y, Fan H. Selenide-doped bismuth sulfides (Bi2S3-xSex) and their hierarchical heterostructure with ReS2 for sodium/potassium-ion batteries. J Colloid Interf Sci 2023;645:654-62.
195. Pang S, Hu Z, Fan C, et al. Insights into the sodium storage mechanism of Bi2Te3 nanosheets as superior anodes for sodium-ion batteries. Nanoscale 2022;14:1755-66.
196. Meija R, Lazarenko V, Rublova Y, et al. Electrochemical properties of bismuth chalcogenide/MXene/CNT heterostructures for application in Na-ion batteries. Sustain Mater Technol 2023;38:e00768.
197. Wang Y, Xu X, Li F, et al. Rational design of bismuth metal anodes for sodium-/potassium-ion batteries: recent advances and perspectives. Batteries 2023;9:440.
198. Li X, Ni J, Savilov SV, Li L. Materials based on antimony and bismuth for sodium storage. Chemistry 2018;24:13719-27.
199. Ellis LD, Wilkes BN, Hatchard TD, Obrovac MN. In situ XRD study of silicon, lead and bismuth negative electrodes in nonaqueous sodium cells. J Electrochem Soc 2014;161:A416-21.
200. Sottmann J, Herrmann M, Vajeeston P, et al. How crystallite size controls the reaction path in nonaqueous metal ion batteries: the example of sodium bismuth alloying. Chem Mater 2016;28:2750-6.
201. Zhang X, Qiu X, Lin J, et al. Structure and interface engineering of ultrahigh-rate 3D bismuth anodes for sodium-ion batteries. Small 2023;19:e2302071.
202. Liang Y, Song N, Zhang Z, et al. Integrating Bi@C nanospheres in porous hard carbon frameworks for ultrafast sodium storage. Adv Mater 2022;34:e2202673.
203. Liu Y, Wang Y, Wang H, et al. Binder-free 3D hierarchical Bi Nanosheet/CNTs arrays anode for full sodium-ion battery with high voltage above 4 V. J Power Sources 2022;540:231639.
204. Pu B, Liu Y, Bai J, et al. Iodine-ion-assisted galvanic replacement synthesis of bismuth nanotubes for ultrafast and ultrastable sodium storage. ACS Nano 2022;16:18746-56.
205. Zhang W, Cao P, Li L, et al. Carbon-encapsulated 1D SnO2/NiO heterojunction hollow nanotubes as high-performance anodes for sodium-ion batteries. Chem Eng J 2018;348:599-607.
206. Li R, Zhang G, Zhang P, et al. Accelerating ion transport via in-situ formation of built-in electric field for fast charging sodium-ion batteries. Chem Eng J 2022;450:138019.
207. Chen Y, Liu H, Guo X, et al. Bimetallic sulfide SnS2/FeS2 nanosheets as high-performance anode materials for sodium-ion batteries. ACS Appl Mater Interf 2021;13:39248-56.
208. Zhou J, Dou Q, Zhang L, et al. A novel and fast method to prepare a Cu-supported α-Sb2S3@CuSbS2 binder-free electrode for sodium-ion batteries. RSC Adv 2020;10:29567-74.
209. Li X, Qu J, Hu Z, Xie H, Yin H. Electrochemically converting Sb2S3/CNTs to Sb/CNTs composite anodes for sodium-ion batteries. Int J Hydrogen Energy 2021;46:17071-83.
210. Li D, Yuan Z, Li J, et al. A bioconfined synthesis strategy of Sb2S3@N-doped carbon ribbons for boosting ultralong-life sodium storage. J Power Sources 2022;546:231875.
211. Zhou J, Ding Y, Dou Q, et al. Enhancing sodium-ion batteries performance enabled by three-dimensional nanoflower Sb2S3@rGO anode material. Mater Chem Phys 2023;303:127837.
212. Li K, Yue L, Hu J, et al. Construction of hollow core-shell Sb2S3/S@S-doped C composite based on complexation reaction for high performance anode of sodium-ion batteries. Appl Surf Sci 2023;613:156111.
213. Dong C, Shao H, Zhou Y, et al. Construction of ZnS/Sb2S3 heterojunction as an ion-transport booster toward high-performance sodium storage. Adv Funct Mater 2023;33:2211864.
214. Liu W, Du L, Ju S, et al. Encapsulation of red phosphorus in carbon nanocages with ultrahigh content for high-capacity and long cycle life sodium-ion batteries. ACS Nano 2021;15:5679-88.
215. Liu X, Xiao B, Daali A, et al. Stress- and interface-compatible red phosphorus anode for high-energy and durable sodium-ion batteries. ACS Energy Lett 2021;6:547-56.
216. Ma X, Ji C, Li X, Liu Y, Xiong X. Red@Black phosphorus core-shell heterostructure with superior air stability for high-rate and durable sodium-ion battery. Mater Today 2022;59:36-45.
217. Song J, Wu M, Fang K, Tian T, Wang R, Tang H. NaF-rich interphase and high initial coulombic efficiency of red phosphorus anode for sodium-ion batteries by chemical presodiation. J Colloid Interf Sci 2023;630:443-52.
218. Saddique J, Zhang X, Wu T, et al. Enhanced silicon diphosphide-carbon composite anode for long-cycle, high-efficient sodium ion batteries. ACS Appl Energy Mater 2019;2:2223-9.
219. Ababaikeri R, Sun Y, Wang X, et al. Scalable fabrication of Bi@N-doped carbon as anodes for sodium/potassium-ion batteries with enhanced electrochemical performances. J Alloys Compd 2023;935:168207.
220. He B, Cunha J, Hou Z, Li G, Yin H. 3D hierarchical self-supporting Bi2Se3-based anode for high-performance lithium/sodium-ion batteries. J Colloid Interf Sci 2023;650:857-64.
221. Wang M, Li H, Cheng X, Tian S, Wang X. Graphene-encapsulated nitrogen-doped carbon@Bi enables rapid, ultrahigh and durable sodium storage. Batteries Supercaps 2023;6:e202300055.
222. Chen J, Zhang G, Xiao J, et al. A stress self-adaptive bimetallic stellar nanosphere for high-energy sodium-ion batteries. Adv Funct Mater 2024;34:2307959.
223. Wei S, Li W, Ma Z, Deng X, Li Y, Wang X. Novel bismuth nanoflowers encapsulated in N-doped carbon frameworks as superb composite anodes for high-performance sodium-ion batteries. Small 2023;19:e2304265.
224. Wang J, Bai W, Zhou Y, et al. Sea cucumber-inspired multi-phase metal sulfides with hierarchical structure towards energy storage with promoted safety. J Energy Stor 2024;76:109743.
225. Hu K, Chen Y, Zheng C, et al. Molten salt-assisted synthesis of bismuth nanosheets with long-term cyclability at high rates for sodium-ion batteries. RSC Adv 2023;13:25552-60.
226. Ma D, Cao Z, Hu A. Si-based anode materials for Li-ion batteries: a mini review. Nanomicro Lett 2014;6:347-58.
227. Pan Q, Wu Y, Zheng F, et al. Facile synthesis of M-Sb (M = Ni, Sn) alloy nanoparticles embedded in N-doped carbon nanosheets as high performance anode materials for lithium ion batteries. Chem Eng J 2018;348:653-60.
228. Guo S, Feng Y, Wang L, Jiang Y, Yu Y, Hu X. Architectural engineering achieves high-performance alloying anodes for lithium and sodium ion batteries. Small 2021;17:e2005248.
229. Ma D, Li Y, Zhang P, Lin Z. Oxygen vacancy engineering in tin(IV) oxide based anode materials toward advanced sodium-ion batteries. ChemSusChem 2018;11:3693-703.
230. Liang S, Cheng Y, Zhu J, Xia Y, Müller-buschbaum P. A chronicle review of nonsilicon (Sn, Sb, Ge)-based lithium/sodium-ion battery alloying anodes. Small Methods 2020;4:2000218.
231. Wang X, Feng B, Huang L, et al. Superior electrochemical performance of Sb-Bi alloy for sodium storage: understanding from alloying element effects and new cause of capacity attenuation. J Power Sources 2022;520:230826.
232. Zheng Y, Wei S, Shang J, Wang D, Lei C, Zhao Y. High-performance sodium-ion batteries enabled by 3D nanoflowers comprised of ternary Sn-based dichalcogenides embedded in nitrogen and sulfur dual-doped carbon. Small 2023;19:e2303746.
233. Gao H, Wang Y, Guo Z, et al. Dealloying-induced dual-scale nanoporous indium-antimony anode for sodium/potassium ion batteries. J Energy Chem 2022;75:154-63.
234. Fu R, Pan J, Wang M, et al. In situ atomic-scale deciphering of multiple dynamic phase transformations and reversible sodium storage in ternary metal sulfide anode. ACS Nano 2023;17:12483-98.
235. Wu J, Ihsan-ul-haq M, Chen Y, Kim J. Understanding solid electrolyte interphases: advanced characterization techniques and theoretical simulations. Nano Energy 2021;89:106489.
236. Peled E, Menkin S. Review - SEI: past, present and future. J Electrochem Soc 2017;164:A1703-19.
237. Yu F, Du L, Zhang G, Su F, Wang W, Sun S. Electrode engineering by atomic layer deposition for sodium-ion batteries: from traditional to advanced batteries. Adv Funct Mater 2020;30:1906890.
238. Yadav P, Shelke V, Patrike A, Shelke M. Sodium-based batteries: development, commercialization journey and new emerging chemistries. Oxford Open Mater Sci 2023;3:itac019.
239. Eddie Spence, Annie Lee; Bloomberg. Tesla rival BYD and other battery giants are betting on sodium for EVs and energy storage - and challenging the dominance of lithium-ion. Available from: https://fortune.com/2023/11/26/battery-giants-sodium-bet-electric-vehicles-energy-storage-lithium-ion/ [Last accessed on 1 Jul 2024].
240. Gebert F, Knott J, Gorkin R, Chou S, Dou S. Polymer electrolytes for sodium-ion batteries. Energy Stor Mater 2021;36:10-30.
241. Li Y, Wu F, Li Y, et al. Ether-based electrolytes for sodium ion batteries. Chem Soc Rev 2022;51:4484-536.
242. Sirengo K, Babu A, Brennan B, Pillai SC. Ionic liquid electrolytes for sodium-ion batteries to control thermal runaway. J Energy Chem 2023;81:321-38.
243. Westman K, Dugas R, Jankowski P, et al. Diglyme based electrolytes for sodium-ion batteries. ACS Appl Energy Mater 2018;1:2671-80.
244. Kulova TL, Skundin AM. Electrode/electrolyte interphases of sodium-ion batteries. Energies 2022;15:8615.
245. Usui H, Domi Y, Fujiwara K, et al. Charge-discharge properties of a Sn4P3 negative electrode in ionic liquid electrolyte for Na-ion batteries. ACS Energy Lett 2017;2:1139-43.
246. Domingues LS, de Melo HG, Martins VL. Ionic liquids as potential electrolytes for sodium-ion batteries: an overview. Phys Chem Chem Phys 2023;25:12650-67.
247. Ahmad H, Kubra KT, Butt A, Nisar U, Iftikhar FJ, Ali G. Recent progress, challenges, and perspectives in the development of solid-state electrolytes for sodium batteries. J Power Sources 2023;581:233518.
248. Gandi S, Chidambara Swamy Vaddadi VS, Sripada Panda SS, et al. Recent progress in the development of glass and glass-ceramic cathode/solid electrolyte materials for next-generation high capacity all-solid-state sodium-ion batteries: a review. J Power Sources 2022;521:230930.
249. Tripathi AM, Su WN, Hwang BJ. In situ analytical techniques for battery interface analysis. Chem Soc Rev 2018;47:736-851.
250. Zhou L, Cao Z, Wahyudi W, et al. Electrolyte engineering enables high stability and capacity alloying anodes for sodium and potassium ion batteries. ACS Energy Lett 2020;5:766-76.
251. Zhang J, Gai J, Song K, Chen W. Advances in electrode/electrolyte interphase for sodium-ion batteries from half cells to full cells. Cell Rep Phys Sci 2022;3:100868.
252. Li Z, Wu Z, Wu S, et al. Designing advanced polymeric binders for high-performance rechargeable sodium batteries. Adv Funct Mater 2024;34:2307261.
253. Chen H, Zhang S, Liu G, Yan C. Polymeric binders in modern metal-ion batteries. In: Zhang S, Lu J, editors. Functional polymers for metal-ion batteries. New York: Wiley; 2023. pp. 61-117.
254. Li RR, Yang Z, He XX, et al. Binders for sodium-ion batteries: progress, challenges and strategies. Chem Commun 2021;57:12406-16.
255. Bresser D, Buchholz D, Moretti A, Varzi A, Passerini S. Alternative binders for sustainable electrochemical energy storage - the transition to aqueous electrode processing and bio-derived polymers. Energy Environ Sci 2018;11:3096-127.
256. Rasheed T, Anwar MT, Naveed A, Ali A. Biopolymer based materials as alternative greener binders for sustainable electrochemical energy storage applications. ChemistrySelect 2022;7:e202203202.
257. Feng J, Wang L, Li D, Lu P, Hou F, Liang J. Enhanced electrochemical stability of carbon-coated antimony nanoparticles with sodium alginate binder for sodium-ion batteries. Prog Nat Sci 2018;28:205-11.
258. Patra J, Rath PC, Li C, et al. A water-soluble NaCMC/NaPAA binder for exceptional improvement of sodium-ion batteries with an SnO2-ordered mesoporous carbon anode. ChemSusChem 2018;11:3923-31.
259. Sarkar S, Roy S, Zhao Y, Zhang J. Recent advances in semimetallic pnictogen (As, Sb, Bi) based anodes for sodium-ion batteries: structural design, charge storage mechanisms, key challenges and perspectives. Nano Res 2021;14:3690-723.
260. Zhang Y, Su Q, Xu W, et al. A confined replacement synthesis of bismuth nanodots in MOF derived carbon arrays as binder-free anodes for sodium-ion batteries. Adv Sci 2019;6:1900162.
261. Choi Y, Lee J. Continuous/reversible phase transition behaviors and their effect on the hysteresis energy loss of the anodes in Na-ion batteries. Electrochim Acta 2019;328:135106.
262. Huang Z, Zheng X, Liu H, et al. Long cycle life and high-rate sodium metal batteries enabled by an active/inactive Co-Sn alloy interface. Adv Funct Mater 2024;34:2302062.
263. Sarkar S, Mukherjee PP. Synergistic voltage and electrolyte mediation improves sodiation kinetics in µ-Sn alloy-anodes. Energy Stor Mater 2021;43:305-16.
264. Wang XZ, Zuo Y, Qin Y, et al. Fast Na+ kinetics and suppressed voltage hysteresis enabled by a high-entropy strategy for sodium oxide cathodes. Adv Mater 2024;36:e2312300.
265. Liu G, Sun Z, Shi X, et al. 2D-layer-structure Bi to quasi-1D-structure NiBi3: structural dimensionality reduction to superior sodium and potassium ion storage. Adv Mater 2023;35:e2305551.
266. Feng D, Tang S, Xu H, Zeng T. High performance sodium-ion anodes based on FeSb2S4/Sb embedded within porous reduced graphene oxide/carbon nanotubes matrix. J Alloys Compd 2023;931:167576.
267. Li C, Pei YR, Zhao M, Yang CC, Jiang Q. Sodium storage performance of ultrasmall SnSb nanoparticles. Chem Eng J 2021;420:129617.
268. Kang J, Lee JI, Choi S, Choi Y, Park S, Ryu J. Nonporous oxide-terminated multicomponent bulk anode enabling energy-dense sodium-ion batteries. ACS Appl Mater Interf 2023;15:26576-84.
269. Gandharapu P, Das A, Tripathi R, Srihari V, Poswal HK, Mukhopadhyay A. Facile and scalable development of high-performance carbon-free Tin-based anodes for sodium-ion batteries. ACS Appl Mater Interf 2023;15:37504-16.
270. Cheng X, Li D, Peng S, et al. In-situ alloy-modified sodiophilic current collectors for anode-less sodium metal batteries. Batteries 2023;9:408.
271. Patel PC, Awasthi S, Mishra PK, Lakharwal P, Kashyap J. Fe-as intermetallic alloys: a way out for sodium-ion batteries. Energy Fuels 2023;37:16062-71.
272. Li H, He Y, Li X, et al. Pomegranate-like Sn-Ni nanoalloys@N-doped carbon nanocomposites as high-performance anode materials for Li-ion and Na-ion batteries. Appl Surf Sci 2023;611:155672.
273. Li W, Yu C, Huang S, et al. Synergetic Sn incorporation-Zn substitution in copper-based sulfides enabling superior Na-ion storage. Adv Mater 2024;36:e2305957.
274. Ye W, Feng Z, Xiong D, He M. Mesoporous C-covered Sn/SnO2-Ni nanoalloy particles as anode materials for high-performance lithium/sodium-ion batteries. Electrochim Acta 2023;471:143401.
275. Sohan A, Kumar A, Narayanan TN, Kollu P. Tin antimony alloy based reduced graphene oxide composite for fast charging sodium-ion batteries. J Energy Stor 2023;74:109312.
276. Chen X, Zhang N, He P, Ding X. High-capacity Sb2SnO5 with controlled Sb/Sn phase modulation as advanced anode material for sodium-ion batteries. J Alloys Compd 2023;938:168472.
277. Meng F, Chen X, Zhou H, et al. Controllable fabrication of Sn/Sb nanodomains improved Sb2SnO5 anodes for sodium ion batteries. ChemistrySelect 2023;8:e202302417.
278. Bhar M, Pappu S, Bhattacharjee U, Bulusu SV, Rao TN, Martha SK. Designing a freestanding electrode of intermetallic Ni-Sn alloy deposit as an anode for lithium-ion and sodium-ion batteries. J Electrochem Soc 2023;170:040501.
279. Priyanka P, Nalini B, Soundarya GG, Christopher Selvin P, Dutta DP. Effect of pulverisation on sulfide and tin antimonide anodes for sodium-ion batteries. Front Energy Res 2023;11:1266653.
280. Hou H, Jing M, Yang Y, et al. Sb porous hollow microspheres as advanced anode materials for sodium-ion batteries. J Mater Chem A 2015;3:2971-7.
281. Kebede MA. Tin oxide-based anodes for both lithium-ion and sodium-ion batteries. Curr Opin Electrochem 2020;21:182-7.
282. Li Z, Zheng Y, Liu Q, et al. Recent advances in nanostructured metal phosphides as promising anode materials for rechargeable batteries. J Mater Chem A 2020;8:19113-32.
283. Sang J, Zhang X, Liu K, et al. Effective coupling of amorphous selenium phosphide with high-conductivity graphene as resilient high-capacity anode for sodium-ion batteries. Adv Funct Mater 2023;33:2211640.
284. Liu M, Zhang J, Sun Z, et al. Dual mechanism for sodium based energy storage. Small 2023;19:e2206922.
285. Ru J, He T, Chen B, et al. Covalent assembly of MoS2 nanosheets with SnS nanodots as linkages for lithium/sodium-ion batteries. Angew Chem Int Ed 2020;59:14621-7.
286. Xu S, Dong H, Yang D, et al. Promising cathode materials for sodium-ion batteries from lab to application. ACS Cent Sci 2023;9:2012-35.
287. Dai Z, Mani U, Tan HT, Yan Q. Advanced cathode materials for sodium-ion batteries: what determines our choices? Small Methods 2017;1:1700098.
288. Jing WT, Yang CC, Jiang Q. Recent progress on metallic Sn- and Sb-based anodes for sodium-ion batteries. J Mater Chem A 2020;8:2913-33.
289. Lin K, Liu Q, Zhou Y, et al. Fluorine substitution and pre-sodiation strategies to boost energy density of V-based NASICON-structured SIBs: combined theoretical and experimental study. Chem Eng J 2023;463:142464.
290. Li F, Yu X, Tang K, Peng X, Zhao Q, Li B. Chemical presodiation of alloy anodes with improved initial coulombic efficiencies for the advanced sodium-ion batteries. J Appl Electrochem 2023;53:9-18.
291. Oh SM, Myung ST, Jang MW, Scrosati B, Hassoun J, Sun YK. An advanced sodium-ion rechargeable battery based on a tin-carbon anode and a layered oxide framework cathode. Phys Chem Chem Phys 2013;15:3827-33.
292. Liu M, Yang Z, Shen Y, et al. Chemically presodiated Sb with a fluoride-rich interphase as a cycle-stable anode for high-energy sodium ion batteries. J Mater Chem A 2021;9:5639-47.
293. He W, Chen K, Pathak R, et al. High-mass-loading Sn-based anode boosted by pseudocapacitance for long-life sodium-ion batteries. Chem Eng J 2021;414:128638.
294. Chen S, Ao Z, Sun B, Xie X, Wang G. Porous carbon nanocages encapsulated with tin nanoparticles for high performance sodium-ion batteries. Energy Stor Mater 2016;5:180-90.
295. Liu Y, Zhang N, Jiao L, Tao Z, Chen J. Ultrasmall Sn nanoparticles embedded in carbon as high-performance anode for sodium-ion batteries. Adv Funct Mater 2015;25:214-20.
296. Nam DH, Kim TH, Hong KS, Kwon HS. Template-free electrochemical synthesis of Sn nanofibers as high-performance anode materials for Na-ion batteries. ACS Nano 2014;8:11824-35.
297. Zhu Y, Yao Q, Shao R, et al. Microsized gray Tin as a high-rate and long-life anode material for advanced sodium-ion batteries. Nano Lett 2022;22:7976-83.
298. Wang L, Ni Y, Lei K, Dong H, Tian S, Li F. 3D porous Tin created by tuning the redox potential acts as an advanced electrode for sodium-ion batteries. ChemSusChem 2018;11:3376-81.
299. Chen B, Zhang H, Liang M, et al. NaCl-pinned antimony nanoparticles combined with ion-shuttle-induced graphitized 3D carbon to boost sodium storage. Cell Rep Phys Sci 2022;3:100891.
300. Li X, Xiao S, Niu X, Chen JS, Yu Y. Efficient stress dissipation in well-aligned pyramidal SbSn alloy nanoarrays for robust sodium storage. Adv Funct Mater 2021;31:2104798.
301. Ni J, Li X, Sun M, et al. Durian-inspired design of bismuth-antimony alloy arrays for robust sodium storage. ACS Nano 2020;14:9117-24.
302. Zhang R, Yang Y, Guo L, Luo Y. A fast and high-efficiency electrochemical exfoliation strategy towards antimonene/carbon composites for selective lubrication and sodium-ion storage applications. Phys Chem Chem Phys 2022;24:4957-65.
303. Tian W, Zhang S, Huo C, et al. Few-layer antimonene: anisotropic expansion and reversible crystalline-phase evolution enable large-capacity and long-life Na-ion batteries. ACS Nano 2018;12:1887-93.
304. Gao H, Niu J, Zhang C, Peng Z, Zhang Z. A dealloying synthetic strategy for nanoporous bismuth-antimony anodes for sodium ion batteries. ACS Nano 2018;12:3568-77.
305. Li W, Han C, Gu Q, Chou S, Liu HK, Dou SX. Three-dimensional electronic network assisted by TiN conductive pillars and chemical adsorption to boost the electrochemical performance of red phosphorus. ACS Nano 2020;14:4609-17.
306. Wu Y, Xing F, Xu R, et al. Spatially confining and chemically bonding amorphous red phosphorus in the nitrogen doped porous carbon tubes leading to superior sodium storage performance. J Mater Chem A 2019;7:8581-8.
307. Liu B, Zhang Q, Li L, et al. Encapsulating red phosphorus in ultralarge pore volume hierarchical porous carbon nanospheres for lithium/sodium-ion half/full batteries. ACS Nano 2019;13:13513-23.
308. Liu D, Huang X, Qu D, et al. Confined phosphorus in carbon nanotube-backboned mesoporous carbon as superior anode material for sodium/potassium-ion batteries. Nano Energy 2018;52:1-10.
309. Zhu L, Xu K, Fang Y, et al. Se-induced fibrous nano red P with superior conductivity for sodium batteries. Adv Funct Mater 2023;33:2302444.
310. Guo X, Zhang W, Zhang J, et al. Boosting sodium storage in two-dimensional Phosphorene/Ti3C2Tx MXene nanoarchitectures with stable fluorinated interphase. ACS Nano 2020;14:3651-9.
311. Sun J, Lee HW, Pasta M, et al. A phosphorene-graphene hybrid material as a high-capacity anode for sodium-ion batteries. Nat Nanotechnol 2015;10:980-5.
312. Shuai H, Ge P, Hong W, et al. Electrochemically exfoliated phosphorene-graphene hybrid for sodium-ion batteries. Small Methods 2019;3:1800328.
313. Liu Y, Liu Q, Zhang A, et al. Room-temperature pressure synthesis of layered black phosphorus-graphene composite for sodium-ion battery anodes. ACS Nano 2018;12:8323-9.
314. Yang H, Xu R, Yao Y, Ye S, Zhou X, Yu Y. Multicore-shell Bi@N-doped carbon nanospheres for high power density and long cycle life sodium- and potassium-ion anodes. Adv Funct Mater 2019;29:1809195.
315. Xiong P, Bai P, Li A, et al. Bismuth nanoparticle@carbon composite anodes for ultralong cycle life and high-rate sodium-ion batteries. Adv Mater 2019;31:e1904771.
316. Cheng X, Yang H, Wei C, et al. Synergistic effect of 1D bismuth nanowires/2D graphene composites for high performance flexible anodes in sodium-ion batteries. J Mater Chem A 2023;11:8081-90.
317. Guo S, Wei C, Wang L, et al. Micro-sized porous bulk bismuth caged by carbon for fast charging and ultralong cycling in sodium-ion batteries. Cell Rep Phys Sci 2023;4:101463.
318. Cheng X, Shao R, Li D, et al. A self-healing volume variation three-dimensional continuous bulk porous bismuth for ultrafast sodium storage. Adv Funct Mater 2021;31:2011264.
319. Wang C, Wang L, Li F, Cheng F, Chen J. Bulk bismuth as a high-capacity and ultralong cycle-life anode for sodium-ion batteries by coupling with glyme-based electrolytes. Adv Mater 2017;29:1702212.
320. Hou D, Xia D, Gabriel E, et al. Spatial and temporal analysis of sodium-ion batteries. ACS Energy Lett 2021;6:4023-54.
Cite This Article
How to Cite
Rehman, A. u.; Saleem S.; Ali S.; Abbas S. M.; Choi M.; Choi W. Recent advances in alloying anode materials for sodium-ion batteries: material design and prospects. Energy Mater. 2024, 4, 400068. http://dx.doi.org/10.20517/energymater.2024.06
Download Citation
Export Citation File:
Type of Import
Tips on Downloading Citation
Citation Manager File Format
Type of Import
Direct Import: When the Direct Import option is selected (the default state), a dialogue box will give you the option to Save or Open the downloaded citation data. Choosing Open will either launch your citation manager or give you a choice of applications with which to use the metadata. The Save option saves the file locally for later use.
Indirect Import: When the Indirect Import option is selected, the metadata is displayed and may be copied and pasted as needed.
About This Article
Special Issue
Copyright
Data & Comments
Data
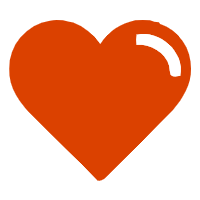
Comments
Comments must be written in English. Spam, offensive content, impersonation, and private information will not be permitted. If any comment is reported and identified as inappropriate content by OAE staff, the comment will be removed without notice. If you have any queries or need any help, please contact us at support@oaepublish.com.