Electrochemically nitrate remediation by single-atom catalysts: advances, mechanisms, and prospects
Abstract
Electrocatalytic nitrate reduction reaction (NITRR) is highly desirable for remediating nitrate (NO3-) pollution and producing ammonia (NH3) under mild conditions. To date, great efforts have been made to fabricate selective, efficient, and stable electrocatalysts for NITRR. Among the numerous strategies, single-atom catalysts (SACs) have received extensive interest and investigations due to their cost-effective and maximum atomic utilization. However, the further development of SACs-based NITRR remains hindered by a poor understanding of their in-depth mechanisms. Consequently, this review summarizes the recent advances of SACs for the NITRR, including Cu-SACs, Fe-SACs, Zn-SACs, Co-SACs, and single-atom alloys. In addition, the characterization techniques for SACs and reaction pathways of NITRR are presented to give a robust understanding of SACs-based NITRR. Finally, we analyze the current challenges in fabricating SACs for NITRR, while the key factors for further improving NITRR performances are also examined.
Keywords
INTRODUCTION
Nitrogen (N) is significant to the agricultural production[1]. The synthesis and employment of nitrogenous fertilizers have made outstanding contributions to increasing agro-food production and ensuring that human society is no longer suffering from food shortage[2,3]. As the world population grows, the excessive use of nitrogenous fertilizers, fossil fuel combustion, and industrial activities lead to a dramatic increase in discharging nitrate (NO3-) to water sources[4]. The release of nitrate-containing wastewater will breach the balance of the nitrogen cycle and bring a range of ecological issues such as eutrophication and drinking water contamination[5,6]. Since the quality of drinking water relates directly to human health, the remediation of nitrate-containing wastewater is becoming a longstanding and challenging task in the current situation. Theoretically, heterotrophic denitrifying bacteria can completely transform NO3- to the harmless nitrogen gas (N2)[7]. However, the denitrifying system requires a long resident time, restricting its large-scale application. Meanwhile, employing carbon sources will cause secondary pollution to the waterbody[8,9]. Additionally, a highly concentrated NO3- wastewater is also prejudicial to the growth of denitrifying bacteria and not conducive to the denitrifying system[10]. Hence, developing efficient and sustainable methods for nitrate-contaminated water source purification is of great significance to both ecological and environmental governance.
In addition to converting nitrates to N2, ammonia (NH3) synthesis offers a promising approach to mitigate the pollution of NO3- and realize nitrogen recovery[11]. In recent years, electrocatalytic nitrate reduction reaction (NITRR) methods have been considered promising for converting nitrate to ammonia[12-18]. The nine protons and eight electrons transfer process (NO3- + 9H+ + 8e- → NH3 + 3H2O) contributes to an efficient and controllable process to produce NH3 under mild conditions, avoiding the huge energy consumption in the Haber-Bosch (H-B) process[15]. Particularly, the high solubility makes NO3- have great compatibility with the liquid-based electrocatalytic systems and alleviates the reaction energy barrier[16]. On the other hand, the protons in the electrocatalytic process directly originate from water dissociation, which can reduce the CO2 emissions of the industrial H2 production. Using NO3- as the N-feedstock, the relatively weak bond of N=O (204 kJ mol-1) makes NITRR have more positive operating potential than N2 (N≡N bond, 941 kJ mol-1)[15-18]. With the development of renewable energy sources, electric-driven catalytic reactions will have a broader prospect in producing NH3 and other chemical compounds, which positions the NITRR method as a promising alternative to the conventional H-B process in future applications. Therefore, it is crucial to exploit optimal electrocatalytic systems to meet the practical demands of NO3- elimination and NH3 synthesis.
Single-atom materials are defined as uniformly exposed metal atom sites on the surface of conductive supports such as metal-organic frameworks (MOFs), zeolites, porous carbons, etc.[19-21]. By achieving nearly 100% atom utilization efficiency, Single-atom catalysts (SACs) lower the cost in synthesizing effective catalysts and provide model systems to bridge the gap between heterogeneous and homogeneous catalysts[22-25]. Due to their unique electronic properties, SACs have demonstrated the superior activities and capabilities in facilitating the electrocatalytic activities by rational structural design with numerous applications of NITRR[26], hydrogen evolution (HER)[27,28], oxygen evolution (OER)[29,30], oxygen reduction (ORR)[31-34], carbon dioxide reduction (CO2RR)[35-38], nitrogen reduction (NRR)[39], and carbon-nitrogen coupling reactions (C-N coupling)[40]. Since transition metals (TMs) have excellent electronic properties to adsorb and reduce nitrate due to the unique d-orbital electron arrangement, they were employed to fabricate SACs for NITRR in recent studies. Theoretically, SACs are more favorable in producing ammonia since the isolated single atoms cannot form N-N bonds during the NITRR process[41]. To prevent the aggregation of single-atom active sites, the metal loading of SACs is hard to exceed 1 at.% in most strategies. Therefore, the effective site number on SACs is far less than on other catalysts[42]. It can be anticipated that SACs are unsuitable for large-scale ammonia production, but they can still serve as an effective catalyst for nitrogen recovery from nitrate-polluted wastewater. Nevertheless, the specific pathways and mechanisms of SACs for NITRR processes remain poorly understood, highlighting the need for systematic exploration. A comprehensive discussion of SACs for NITRR applications would deepen our understanding of corresponding mechanisms and provide new insights for SAC design.
To this end, the review summarizes the advances of SACs for NITRR applications. By categorizing SACs as Cu, Fe, other SACs (zinc (Zn)-, cobalt (Co)-, zirconium (Zr)-, and nickel (Ni)-SACs), and single-atom alloys (SAAs), it explores the relationship between characteristic active sites and properties and the techniques for SAC characterization. By recognizing the unique structures and properties of SACs in NITRR, the mechanisms, advantages, and challenges are fully discussed. It is worth noting that research of SACs for NITRR is still in its early stages. Therefore, by summarizing the recent advances of NITRR-based SACs, we hope this review can refine the routes for electrocatalytic NO3- to NH3 reduction and shed light on the further development of nitrate remediation.
SACS FOR NITRR
Cu SACs
Cu has unpaired d-electrons and similar d-band energy to the LUMO (lowest unoccupied molecular orbital) Π anti-bonding orbital of NO3-[43]. The tunable electronic structure, high abundance, flexible electrochemical activity, low cost, and good electron injection properties make Cu-based catalysts an optimal candidate for NITRR[44]. Although Cu-based catalysts demonstrated relatively high NITRR activities, their large overpotential, nitrite accumulation, and poor catalyst stability still limit their further applications. As mentioned, SACs have nearly 100% atom utilization efficiency, and Cu SACs can be desirable candidates to improve the NITRR performance[22]. Therefore, tremendous investigations have emerged to develop selective, efficient, and robust Cu SACs with lower operating potentials in the past five years.
Zhao et al. proposed a Cu SAC with boron-doped carbon nitride (BCN) heterojunction for treating nitrate to ammonia[45]. By exchanging counter anion NO3- to large-sized closo-[B12H12]2-, the 1,10-phenanthroline-Cu2+ complex exhibits a uniform diamond-shaped nanosheet structure with Cu-N4 sites. As a result, the BCN@Cu performs 83.95 mg h-1 mgcat-1 NH3 yield rate, 97.37% Faradaic efficiency at -0.6 V vs. Reversible Hydrogen Electrode (RHE). Their follow-up study found that the 1,10-phenanthroline-Cu2+-closo-[B12H12]2- structure has the chance to strengthen the attachment to carbon nanotube (CNT) carriers and form BCN@Cu/CNT after pyrolysis[46]. On the other hand, the doping of boron (B) facilitates the formation of the local electric field, enveloping the reaction in a huge electric field atmosphere. As a result, the electronic property and physicochemical stability of the catalyst are greatly improved, and considerable BCN@Cu/CNT conductivity is achieved. With a high NH3 yield of 172.2265 mg h-1 mgcat-1 and 95.32% Faradaic efficiency at -0.6 V vs. RHE, BCN@Cu/CNT exhibits three times NITRR activity than that of BCN@Cu
Figure 1. (A) Structure investigation of BCN@Cu/CNT by SEM characterization; (B) NO3- removal efficiency of BCN@Cu/CNT. This figure is quoted with permission from Zhao et al.[46]; (C) TEM image of Cu-N-C-800; (D) The removal of NO3- and current efficiency using Cu-N-C-800. This figure is quoted with permission from Zhu et al.[47]; (E) Product distribution of NITRR process using Cu SAAs as the electrocatalyst at varying potentials. This figure is quoted with permission from Li et al.[52]; (F) FEs of NH3 and NO2- using
Porous-rich MOFs are great candidates for anchoring single atoms due to their well-defined channel structure and structural diversity. In this class of materials, zeolitic imidazolate frameworks (ZIFs) are the most commonly used substrates owing to their facile synthesis, high surface area, and high porosity.
According to density functional theory (DFT) calculations, the performance of most planer substrate-supported Cu-N4 is unsatisfactory. To this end, Xue et al. reported a Cu site anchored micro-mesoporous N-doped carbon (Cu MNC)[49]. Cu MNC-7 demonstrated a considerable NITRR process with 94.8% NO3- conversion, 92.922 mg h-1 mgcat-1 NH3 yield rate at -0.64 V vs. RHE by changing urea/Cu2+ molar ratio to 7. Compared to Cu-N-C, the specific surface area and pore structure are increased in the skeleton of Cu MNC-7. In Cu MNC-7, the Cu atom formed three single bonds to connect with nitrogen and one bond to connect with carbon, giving Cu-N3-C sites more specific electronic and geometric configurations than
Li et al. reported a functional single-atom aerogel (Cu-SAA) containing 3D channel framework structure with coordinated Cu single-atom sites[52]. In addition to having an 87% FENH3 and > 0.3 mg cm-2 h-1 NH3 yield rate in NITRR, the aerogel also exhibited a remarkable performance for detecting NO3- and NH4+ [Figure 1E]. Further implementing Cu-SAA in a smart and sustainable fertilizing system (SSFS) enables simultaneous ammonia production and monitoring of NO3- and NH4+ concentrations during reaction. In addition, the proposed system realized high feasibility for controlling the real-time concentrations of
Although SACs exhibited great potential in transforming NO3- to NH3, it remains controversial for the key factors and mechanisms in SACs-driven NITRR. To this end, Yang et al. employed Cu-N-C SAC as the model catalyst to investigate the evolution of Cu active sites by operando X-ray absorption spectroscopy (XAS) at the Cu K-edge during the NITRR process[53]. By operating potentials from 0.00 to -1.00 V vs. RHE, a successive morphological transformation of Cu could be observed in the following order: Cu-N4 → Cu-N3 → Cu0 single atoms → Cu0 aggregates. According to the results of X-ray absorption near edge spectroscopy (XANES) spectra, the edge energy of Cu K-edge spectra continuously decreases with changing potentials due to the reduction of Cu species. When the reduction potential was applied to the Cu-N-C catalyst, Cu2+ priorly disappeared in the Cu structure, while Cu+ maintained the catalyst structure with a declined Cu+/Cu0 ratio. Interestingly, minor contributions were achieved from the N-doped carbon embedded Cu+ (48.3%), while the remaining 50% Cu tended to create a dynamic equilibrium between Cu0 and Cu+ and dominated the Faradaic efficiency of NITRR. Compared to NaBH4-reduced CuNPs/N-C, the in-situ formed Cu0 aggregates are more favorable to the NITRR process due to the higher selectivity to NO2- on CuNPs/N-C [Figure 1F]. However, this work only investigated the evolution of one Cu-N-C catalyst in NITRR. The universality of their theory still needs to be verified. In the subsequent SAC-driven NITRR studies, accurately defining the actual active sites is urgent rather than arbitrarily attributing the activity to the metal-N sites.
Fe SACs
Since iron is earth-abundant and has moderate adsorption ability to oxygen and nitrogen, numerous studies have emerged to treat nitrogen based on the Fe-containing materials (Fe-based H-B catalysts, nitrogenase enzymes, etc.)[54]. Zero-valent iron was first used for nitrate removal to produce harmless N2 in water resources, and its particle size directly influenced the nitrogen removal efficiency[55]. However, metallic Fe tends to form iron oxides with poor conductivity and activity which can lead to the passivation, leaching, and dissolution effects on the surface of Fe catalysts. In this case, Fe SACs were designed to minimize the disadvantages with boosted surface area and a significantly improved performance for NITRR.
Wu et al. developed Fe SACs with Fe-N4 active sites for NITRR[56]. The lower thermodynamic barrier of
Figure 2. (A) AC-HAADF-STEM image of FeSAs/g-C3N4; (B) The conversion of NO3--N and FE of NH3 using FeSAs/g-C3N4; (C) N product distributions in the presence of chlorine; (D) Differential charge density of NO3- adsorbed on FeSAs/g-C3N4. This figure is quoted with permission from Song et al.[57]; (E) TEM image of Fe SAC; (F) FENH3 using Fe SAC at different applied potentials; (G) The DOS of the
As aforementioned, the M-N4 structure has a symmetrical electronic density distribution from the coordination of the metal center with four N atoms, which is not conducive to the adsorption and activation of N-intermediates. Based on the recently published studies, introducing intrinsic and heteroatom doping defects is regarded as a better strategy to overcome these obstacles. Xu et al. proposed a P-doped Fe-N-C SAC which performed a 90.3% FENH3 at -0.4 V vs. RHE. At -0.8 V vs. RHE, a partial current density of
Other metal SACs
In addition to Fe and Cu SACs, Zn, Ni, Zr, and Co-based SACs are also investigated and gained better NITRR performances. Zhao et al. proposed a N-doped carbon-supported Zn SAC (ZnSA-MNC) with microporous structure and achieved 97.2% NO3- conversion, 94.9% NH3 selectivity, 39.27 mg h-1 mgcat-1 NH3 yield rate at -1.0 V vs. RHE. Meanwhile, a 94.8% FENH3 can be obtained at -0.9 V vs. RHE [Figure 3A-C][62]. The coordination of Zn and N offers Zn sites a positive charge due to the stronger electronegativity of N (3.04) than that of Zn (1.65). As a result, the capture of NO3- on Zn active sites was greatly improved by means of the redistribution of charge density. ZnSA-MNC also exhibited a 1.46 eV barrier to avoid the desorption of NO*, which facilitated the follow-up N-end hydrogenation process for NH3 production.
Figure 3. (A) SEM image of ZnSA-MNC; (B) The CNO3-, SNH3, and FENH3 of ZnSA-MNC at different working potentials; (C) The NH3 yield of ZnSA-MNC, ZnNP-MNC, and MNC at different working potentials. This figure is quoted with permission from Zhao et al.[62]; (D) AC-HAADF-STEM image of Zr-TiON; (E) Stability of Zr-TiON. This figure is quoted with permission from Yang et al.[63]; (F) HR-TEM image of NISA@BNG catalyst; (G) FENH3 using NG, BNG, NISA@NG, NISA@BNG as the catalyst. This figure is quoted with permission from Ajmal et al.[64]; (H) High-resolution TEM and HAADF-STEM images of Co-CN and Co-CNP; (I) NH4+-Faradic efficiency and NH4+-yield rate using Co-CN and Co-CNP at different working potentials. This figure is quoted with permission from Li et al.[65].
Single-atom alloys
Alloying active metals with other heterogeneous metals is an effective avenue to improve the performance of catalysts[66-69]. The atomic structure of active sites and the adsorption energy barrier of reactants and intermediates can be optimized through the alloying step. For instance, Wang et al. utilized the element Ni to build CuNi alloy catalysts by tuning the molar ratios between Cu and Ni[70]. The Cu50Ni50 catalyst exhibits a six-fold activity enhancement than pure Cu at 0 V vs. RHE, which can be attributed to the adsorption energy modification by tuning the d-band center of Cu towards the Fermi level[70]. However, the performance of Cu50Ni50 is unsatisfactory in lower nitrate concentrations, which can be ascribed to the Ni-Ni coordination induced HER on the catalyst surface. Single-atom alloys (SAAs) are a metal matrix with foreign active metal atoms dispersed across the surface. In this case, the active atoms are diluted in the alloy system, providing a unique electronic structure and geometric characteristics to SAAs. As a result, SAAs may have both unique bimetallic alloying effect and maximum atom-utilization efficiency.
Cu-based materials have excellent *NO3 adsorption capacity. However, the weak *H adsorption on Cu limits the hydrogenation steps and affects the overall performance of NITRR. Accordingly, based on the studies of PdCu and NiCu alloying catalysts, doping metal heteroatoms on the surface of Cu substrates would be a better strategy. Du et al. proposed a facet-dependent PdCu SAA by spreading Pd atoms on CuNPs [Figure 4A and B][71]. Given that Pd atoms are uniformly separated, the intercouple of *H was suppressed. Compared to the higher energy barrier of *NOO hydrogenation (0.39 eV) on the Cu (100) facet, the PdCu (100) facet shows a significantly decreased energy barrier of *NHOH (0.10 eV). Consequently, the generated *H intermediates prefer to desorb and react with N-intermediates on the neighboring Cu sites, thus offering PdCu SAAs an ultrahigh 97.1% FENH3 and 0.26 mg h-1 cm-2 NH3 yield rate at -0.6 V vs. RHE. Similarly, Liu et al. alloyed Rh atoms with Cu substrates to overcome the weak *H adsorption on bare Cu. With a low Rh loading (0.6%), the Rh@Cu SAA revealed a partial current density of 162 mA cm-2 for NH3 production with 93% FENH3 and 21.59 mg h-1 cm-2 NH3 yield rate at -0.2 V vs. RHE[72]. The cooperation between Rh and Cu sites on Rh@Cu SAA comprehensively improves NITRR performances. Based on the computational methods, the *H intermediates are preferred to generate on Rh sites, and Rh active sites play an important role in transferring hydrogen for the N hydrogenation process on Cu sites. Therefore, the free energy of NITRR steps is decreased when Rh atoms are spread on Cu substrates. Cai et al. reported a Ni1Cu SAA by in-situ electrochemically incorporating Ni single atoms into a Cu catalyst[73]. They found the alloyed structure of Ni1Cu exhibited a larger electron cloud than pristine Cu-Cu, which increased the energies of unoccupied anti-bonding N2p states above the Fermi level and inhibited byproducts production from NOOH* intermediates desorption. The addition of Ni significantly reduced the operating potential of the proposed NITRR system, thus weakening the implications of the HER process. At -0.55 V vs. RHE, the proposed system obtained a nearly 100% FENH3. Compared to the unincorporated Cu catalyst, the Ni1Cu SAA shows a 10.7 times enhancement of the NH3 yield rate (5.5539 mg h-1 cm-2). In addition, they fabricated a Ni1Cu SAA-based Zn-nitrate battery with a power density of 12.7 mW cm-2 to realize energy output and NH3 production in a single module.
Figure 4. (A) AC-HAADF-STEM image of PdCu SAA; (B) FENH3 of Cu and PdCu SAA. This figure is quoted with permission from
Yin et al. proposed an Au/Cu SAA to combine the advantages of Au SACs and Cu-based catalysts[74]. By anchoring Au atoms on the Cu (100) facet, the NITRR performance was greatly improved on Au/Cu (100) SAAs [Figure 4C and D]. Additionally, incorporating Au single atoms effectively avoids the formation of
While d-block TMs are widely used in fabricating single atom-based catalysts, p-block metals are rarely investigated as active centers for building SAAs. To this end, Chen et al. proposed a Pd-supported Bi-SAA (Bi1Pd) catalyst for NITRR [Figure 4E-H][76]. Depending on the DFT calculations and experimental results, NO3- can be activated on both Pd and Bi1Pd sites due to the electron transfer and accumulation mechanisms. The alloying between Bi and Pd facilitated the tuning of the energy barrier of the potential determining step of *NO to *NOH and the thermodynamic parameters of NITRR. Benefiting from the less negative binding free energy of *H on Pd and Bi1Pd surfaces, the *H tends to bind with NO3- rather than combine with other *H on the active sites. As a result, an ~100% FENH3 and 33.8 mg h-1 cm-2 NH3 yield rate were achieved by the Bi1Pd catalyst at -0.6 V vs. RHE. Xie et al. proposed a p-block metal incorporated intermetallic SAA In-Pd bimetallene (ISAA InPdene) for NITRR. The p-d hybridization between Pd and In provides a narrowed energy band, facilitating the rate-limiting step from *NO to *NHO[77]. Benefiting from the intermetallic structures, single atom characteristics, and metalline properties, ISAA InPdene exhibits an 87.2% FENH3 and 28.06 mg h-1 cm-2 NH3 yield rate at -0.6 V vs. RHE in neutral electrolytes.
In recent studies, most SAAs have focused on transforming NO3- to NH3, while few studies are dedicated to transferring NO3- to N2. In environmental considerations, the N2 generation pathway would be preferred for water remediation scenarios. To this end, Wu et al. developed PdCu alloy catalyst and PdCu SAA for thermo-catalytic and electrocatalytic treatment of NO3- towards N2, respectively [Figure 4I-L][78]. They revealed that PdCu alloys and PdCu SAAs benefit from the thermo-catalytic and electrocatalytic processes, respectively. By changing the molar ratios of Pd and Cu in PdCu alloys and PdCu SAAs, Pd/Cu(3:1) shows great N2 selectivity (77%) with 19% NH4+ selectivity under thermo-catalytic conditions. On the other hand, the N2 selectivity reached 94% with the capability to suppress HER under electrocatalytic conditions on Pd/Cu(1:100) SAA. The authors investigate the differentiated mechanisms behind the thermo-catalytic and electrocatalytic processes of Pd/Cu(1:100) by DFT calculations. A higher NO3* stabilization on Pd/Cu(1:100) can be achieved by the electrocatalytic process, while the adsorbed NO3- is more likely to desorb into the solution under the thermo-catalytic conditions, thus leading to a higher electrocatalytic NO3- reduction activity. Moreover, due to the localized pH effects and proton extraction capability, N*-N* coupling shows a lower formation energy barrier than the *N-*H coupling process on Pd/Cu(1:100) under electrocatalytic conditions. As a result, the electrocatalytic process is conducive to N2 generation.
SYNTHETIC METHODS OF SACS FOR NITRR
Constructing strong interaction between metal single atoms and supports is a key step to improving the atom-utilization efficiency and the stability of SACs[79]. Typically, impregnation, coordination site construction (MOFs and polymerization stabilized), and defect engineering are the major “bottom-up” strategies for building SACs [Figure 5]. This section summarizes the synthetic methods based on recent advances in SACs-based NITRRs.
Figure 5. (A) Strategies for synthesizing Cu NC, Cu MNC, and Cu MNC-x. This figure is quoted with permission from Xue et al.[49]; (B) Synthesis routes for Cu-N-C-T. This figure is quoted with permission from Zhu et al.[47]; (C) Schematic illustration of the synthetic procedure of Cu-N-C. This figure is quoted with permission from Chen et al.[41]; (D) Schematic illustration of FeSAs/g-C3N4 synthesis. This figure is quoted with permission from Song et al.[57]; (E) Strategy for synthesizing Cu-N3 SACs/NCNT. This figure is quoted with permission from Wang et al.[50]; (F) Schematic illustration of the synthesis of Ni1Cu-SAA. This figure is quoted with permission from
Impregnation and coprecipitation strategy
To effectively disperse individual metal atoms onto suitable supports, impregnation and coprecipitation are usually used in synthesizing SACs. Through the impregnation process, metal precursors are uniformly dispersed on the employed support at the initial step. After impregnating for optimal times, the obtained materials will be dried and calcined at high temperatures in the presence of a specific atmosphere. For impregnation strategy, metal loading, precursor selection, and pyrolysis parameters extremely influence the distribution and the performance of metal atoms. Therefore, the optimal chemical environment should be fully investigated during the synthesis.
Xue et al. proposed a two-step strategy to prepare Cu MNC-x SAC[49]. To synthesize Cu MNC, SiO2-coated Cu-ZIF-8 (Cu-ZIF-8@SiO2) was pyrolyzed at 650 °C in an argon atmosphere, followed by a SiO2 etching process in NaOH solution. Next, the Cu MNC (650) was reacted with different urea/Cu2+ molar ratios in a solution. The urea/Cu2+ can adsorb on the pores of Cu MNC (650) and form urea/Cu2+-Cu MNC (650), which further underwent a pyrolysis process at 950 °C in an argon atmosphere. In the product Cu MNC-x, Cu2+ ions can stabilize on the vacancies generated from the evaporated Zn and form stable Cu-N3-C sites with specific surface area and pore structure to prevent the aggregation of Cu atoms. Yang et al. prepared Cu-N-C SAC to investigate the reconstruction of Cu single atoms during the NITRR process[53]. In the initial step, precursors were synthesized by polymerizing 2,6-diaminopyridine on the SiO2 template. The obtained solid was then pyrolyzed in an NH3/He gas atmosphere at 800 °C to get SiO2@N-C. Next, SiO2 was leached by HF solution, and the remaining product is the N-C support. After impregnating N-C supports by Cu(NO3)2 solutions, the Cu-doped N-C was further pyrolyzed in a N2 gas atmosphere at 800 °C to get the Cu-N-C SACs. Yin et al. prepared Au/Cu SAAs through a H-spillover method[74]. Using Cu2O with (100) facets as the support material, ultrapure water dispersed Cu2O, HAuCl4 solution, and urea were mixed for
MOFs stabilized SACs
The spatial confinement strategy is an efficient way to construct SACs by immobilizing single atoms on the surface of supports to maximize catalytic efficiency. As a case in point, MOFs show great promise to spatially separate metal atoms in their porous skeletons. Meanwhile, MOFs that contain nitrogen ligands can also provide N and O sites to stabilize metal atoms. Typically, MOF-based SACs undergo a process as follows: (1) the construction of MOFs with specific skeletons; (2) the pyrolysis of MOFs through a programmed thermal treatment process; and (3) the pickling process to remove unwanted metallic residues and impurities using acid.
Zhu et al. reported Cu-entrapped nitrogenated carbon nanosheets (Cu-N-C) using Cu-MOFs as the precursor[47]. The Cu-MOF was first prepared by mixing the metal salts and ligands. After drying the collected Cu-MOF, the precipitate was mixed with dicyandiamide and ground into a homogeneous precursor. Subsequently, the precursor was pyrolyzed at 700-1,000 °C in an argon atmosphere. The obtained products were then leached by HCl to remove the unwanted metallic residues. By raising the pyrolysis temperature to 900 °C, the active Cu atoms tend to aggregate and generate CuNPs with weakened NITRR activities, which suggests the pyrolysis temperature is a key factor in influencing the interactions between N sites and metal atoms. Chen et al. reported a one-pot ionic exchange method to construct a Cu-N-C structure[41]. Firstly, Cu(NO3)2, Zn salt and 2-methylimidazole were mixed in methanol and stirred for 12 h at room temperature to get Cu-doped ZIF-8. After pyrolyzing the precipitate at 950 °C in an argon atmosphere, a ZIF-8-shaped porous framework with Cu-N4 sites was successfully obtained. Zhao et al. proposed a similar strategy to stabilize Cu SACs by direct carbonization of Cu-doped ZIF-8[48]. Unlike the strategy by Chen et al., the ligands and Cu salt underwent a hydrothermal process in a Teflon-lined autoclave. After a carbonizing process at 1,000 °C in a N2 atmosphere, a N-doped porous carbon anchored Cu SAC with Cu-N sites was successfully prepared.
Polymerization stabilized SACs
Polymer materials usually contain N heteroatoms, which is beneficial in supplying coordination sites to build and separate single-atom sites. The polymerization process could employ hard templates, such as MnO2, SiO2, and CNTs, to achieve porous structures of catalysts after the pyrolysis and etching processes.
Wang et al. prepared Cu-N/P SACs through a template-free oxidative polymerization method[51]. Initially, the pyrrole monomer (C and N sources) and phytic acid (P source) were mixed in an ethanol/water mixture. Next, CuCl2 solution was added to the above mixture to form hydrogel at 4 °C. After the purification and freeze-drying process, the powder was further pyrolyzed in an argon gas atmosphere at
Using CNTs as the hard template, Zhao et al. further improved the performance of BCN@Cu SACs in the NITRR process[46]. Initially, CNTs were added to the mixture of 1,10-phenanthroline and Cu salt solution. The mixture solution was then sonicated for 30 min to obtain a metal boron cluster organic polymer (MBOP)/CNT precursor. After a pyrolysis process at 1,000 °C, MBOP/CNT was converted to the BCN@Cu/CNT and achieved greater conductivity and NITRR activity than BCN@Cu. Wang et al. proposed Cu-N3 SACs/NCNT through a polymerization-surface modification-electrostatic adsorption-carbonization strategy. Firstly, pyrrole and aniline were polymerized on the surface of MnO2 nanowires, which generated N-rich polymer PPy-co-PANI with hollow structures. The surface of the obtained poly(pyrrole-co-aniline) (PPy-co-PANI) was further modified by sodium dodecyl benzene sulfonate (SDBS) to modify its surface charge state and wettability. Since the surface of SDBS-modified PPy-co-PANI is more hydrophilic, Cu2+ ions were more likely to adsorb on PPy-co-PANI through the electrostatic attraction. After the carbonization at 800 °C in an argon gas atmosphere, the Cu-N3 SACs/NCNT was successfully prepared. Wu et al. prepared Fe SACs through the polymerization-pyrolysis process using SiO2 as the hard template[56]. o-phenylenediamine, FeCl3, and SiO2 powder were initially mixed into isopropyl alcohol and stirred for 12 h. The mixture was dried to a solid state through a rotary evaporator, following pyrolysis in an argon gas atmosphere at 800 °C. Finally, the as-prepared product was leached by alkaline and acid to remove the SiO2 template and poorly cooperated metals to get Fe SAC.
Defects stabilized SACs and SAAs
Defects engineering of support materials is an emerging approach to optimize catalyst performance and build SACs with unsaturated structures. The defects on the support can be vacancies, interstitial atoms, or substitutional atoms. Introducing defects on the support materials can affect charge density at the active sites, thus influencing the stability and reactivity of SACs.
Wang et al. prepared Fe-TiO2 SACs with interfacial defect by incorporating Fe atoms on the TiO2 support through adsorption and subsequent reduction strategy[61]. Firstly, the as-prepared TiO2 nanowire and iron acetylacetonate were added into a CH3CN solution and stirred for 12 h. After washing and drying the obtained suspension, the solid was then calcined in an Ar/H2 gas atmosphere at 350 °C to get Fe-TiO2 SACs. Du et al. proposed PdCu SAA by the galvanic replacement of partial Cu atoms to Pd atoms. At the initial step, CuNPs were deposited on the carbon paper through an electrochemical process under the potential of -0.3 V vs. Ag/AgCl[71]. Subsequently, carbon paper containing CuNPs was further immersed into a Na2PdCl4 solution. The galvanic replacement reaction was spontaneously driven due to the differences of reduction potential between Cu and Pd. After the washing and drying, PdCu SAA was successfully obtained on the carbon paper. Similar approaches are also applied by Liu et al. to build Rh@Cu SAA[72]. To build NiCu-SAA, Cai et al. immersed a piece of Ni foam into a solution containing Cu(NO3)2, NH4F, and urea, following a hydrothermal synthesis process[73]. After washing and drying steps, the obtained NiCu oxide or hydroxide nanosheet catalyst (NiCu-NS) foam material underwent an in-situ electrochemical reconstruction to get NiCu-SAA. Zhang et al. designed VCu-Au1Cu SAAs by combining galvanic replacement and vacancy engineering strategies[75]. For the galvanic replacement, an aqueous solution of HAuCl4 was added dropwise to the HCl solution containing CuNS. The mixture was then thermal treated at 100 °C for 20 min. After filtration, washing, and drying steps, Au1Cu SAAs were obtained. To get vacancy-engineered SAAs, these SAAs were then immersed into the acetic acid solution and thermal treated at 60 °C for 1 h.
In addition to the above strategies, the microwave-assisted, atomic layer deposition, chemical vapor deposition, and other methods are also efficient for building SACs[80-82]. Combining the further quantification of ammonia and other byproducts in NITRR, these strategies provide opportunities to realize high-performance NITRR processes in future applications.
METHODS FOR AMMONIA AND BYPRODUCTS QUANTIFICATION
Quantifying ammonia and byproducts is beneficial for determining the validity of SACs in the NITRR processes. To ensure accurate results, a combination of different quantification methods is suggested.
Nessler’s reagent method for ammonia quantification
The colorimetric quantification of ammonia by Nessler’s reagent is based on the reaction between ammonia and mercury(II) ions in an alkaline medium[83].
After stabilizing for a few minutes, the yellow-brown HgOHg(NH2)I complex shows characteristic absorbance at the wavelength between 410 and 425 nm. By plotting a calibration curve through the standard ammonia solutions with known concentrations, the concentrations of ammonia in unknown samples can be detected. Given that mercury ions are present in Nessler’s reagent, experimental rules must be strictly followed during the storage and use process to avoid hazards caused by mercury ingestion. Recently, portable equipment for ammonia testing has also been developed based on Nessler’s reagent method, which avoids the inaccurate results caused by ammonia volatilization.
Indophenol method for ammonia quantification
Indophenol is another widely used colorimetric technique for quantifying ammonia in aqueous solutions[84]. Under alkaline conditions, the reaction between ammonia, sodium hypochlorite, and phenol tends to form an indophenol complex, with color changing from yellow to blue. The indophenol blue complex shows characteristic absorbance at the wavelength between 630 and 660 nm. In recent studies, phenol is replaced by sodium salicylate to reduce the output of phenol waste from the colorimetric test. Similarly, the reaction between ammonia, sodium hypochlorite, and sodium salicylate also generates complexes with characteristic absorbance at the wavelength between 630 and 660 nm. By establishing a calibration curve, the concentrations of ammonia in unknown samples can be detected by the spectrophotometric measurements.
1H NMR method for ammonia quantification
The proton nuclear magnetic resonance (1H NMR) method can also quantify ammonia in various samples[85]. By choosing suitable solvents such as D2O with a standard compound (e.g., dimethyl sulfoxide (DMSO)), the unique proton signals in the spectrum will show typical triple peaks of 14NH4+ and double peaks of 15NH4+ around 7-8 ppm. A calibration curve can be generated according to the peak area from samples with known ammonia concentrations.
Naphthalene ethylenediamine hydrochloride method for nitrite quantification
In this method, p-aminobenzene-sulfonamide, N-(1-Naphthyl) ethylenediamine dihydrochloride, and phosphoric acid were mixed to obtain a color reagent with acidic micro-environment[86]. After adding the color reagent to the nitrite-containing solutions, naphthalene ethylenediamine reacts with nitrite ions to form a purple diazonium salt with a characteristic absorbance at a wavelength of around 540 nm. A calibration curve allows the detection of nitrite concentrations in unknown samples through spectrophotometric measurements.
1,10-phenanthroline method for hydroxylamine quantification
The 1,10-phenanthroline method relies on the formation of Fe2+ from the reaction between Fe3+ and hydroxylamine[87]. Subsequently, 1,10-phenanthroline tends to react with Fe2+ and form a ferrous complex, which shows characteristic absorbance at the wavelength around 510 nm. Similarly, by establishing a calibration curve, spectrophotometric measurements can detect the concentrations of hydroxylamine in unknown samples.
TECHNIQUES FOR SACS-BASED NITRR CHARACTERIZATION
Characterizations are crucial to reveal the intrinsic mechanism of SACs in NITRR. Accompanied by experimental results, the comprehensive combination of characterization techniques will guide us in finding an optimal way to enhance the performance of the SACs-based NITRR system. In addition to regular X-ray diffraction (XRD), Scanning Electron Microscope (SEM), Transmission Electron Microscope (TEM), Fourier Transform infrared spectroscopy (FTIR), Ultraviolet-visible (UV-vis) absorption spectra, and X-ray Photoelectron Spectroscopy (XPS) techniques, the emerging approaches can provide in-depth insights to the relevant reaction kinetics during the NITRR process, thus obtaining actual active sites and mechanisms[88].
Aberration-corrected high-angle/medium-angle annular dark-field scanning transmission electron microscopy (AC-HAADF-STEM) offers capabilities for observing the precise locations and microenvironments of materials supported by single atoms, which allows researchers to identify the atomic metal dispersion through the bright spots in the HAADF-STEM images[89]. The AC-HAADF-STEM provides information in distinguishing different element species in the atom-support composites. Through the AC-HAADF-STEM images, the arrangement of single atoms and their interaction with the support material will be recognized[90]. Scanning tunneling microscopes (STM) are another effective tool to characterize surface geometric and electronic structures of SACs at atomic resolution and provide insights into single-atom catalysis[91]. In STM, the topographical and electronic differences of metals can be discerned and exhibited different corrugations under certain tip conditions. Therefore, the bright protrusions of single atoms can be observed and distinguished from their supports. In addition, STM can reveal the interactions between single atoms and coordinative sites, facilitating the understanding of adsorption capability in different local coordination environments. Since structural evolution is also a key parameter for SACs-based reactions, the environmental TEM (ETEM) was developed for in-situ monitoring dynamics and atomic structural transformations of single atoms during actual chemical reactions[92].
In addition to these direct observation methods, XAS is also a powerful tool to investigate the electronic structure and coordination structures of SACs [Figure 6A-C][56]. In general, the XAS technique can be divided into XANES and extended XAFS (EXAFS) spectroscopy based on the differences of obtained information[93]. The XANES technique determines the electronic state and the valence of active centers, while EXAFS provides abundant information for coordination elements, coordination number, bond length, debye-waller factor, etc. Wu et al. performed XAS analysis on the Fe SACs during the NITRR process[56]. Through XANES measurements, they found the oxidation state of Fe sites is between 0 and +3 valence. Additionally, the EXAFS results illustrate the existence of Fe-N coordination and the absence of Fe-Fe coordination. As a further extension of XAS technology, the operando XAS strategy provides an opportunity to monitor the dynamic behavior of geometric structure of SACs and electronic environment during the catalysis process[93]. At the atomic scale, the structural evolution of metal-Nx sites can be monitored according to the peak shifts of the EXAFS spectrum.
Figure 6. (A) The XANE spectra of Fe SAC, Fe foil and Fe2O3 at Fe K-edge; (B) FT k3-weighted χ(k)-function of the EXAFS spectra at
Operando synchrotron radiation (SR)-FTIR spectroscopy is another powerful tool that reveals the reaction pathway by observing key intermediates during the reaction. Typically, NO3- shows a negative band at around 1,360 cm-1, while the *NO, *NO2, NH2, *NH2OH, and the produced NH4+ correspond to the positive bands at around 1,580, 1,230, 1,270, 1,150, and 1,460 cm-1, respectively[94]. For instance, Xu et al. utilized operando SR-FTIR to monitor the intermediates of NITRR when using Fe-N/P-C SAC as the catalyst. By varying operation potentials from 0 to -0.8 V vs. RHE, an increased peak intensity of NO3- was observed at 1,390 cm-1, which can be attributed to the continuous consumption of NO3- on the active sites. Furthermore, the NO2- stretching mode peak at 1,290 cm-1 indicates a deoxygenation step occurred during the NO3- reduction, providing strong evidence for their proposed reaction pathways [Figure 6D and E][59].
Additionally, the online DEMS efficiently monitors the intermediates during the NITRR process
MECHANISMS OF SACS-BASED NITRR
Electrochemical NO3- remediation requires complex steps to obtain the target products (NH3 and N2). Accordingly, various by-products (NO2-, N2O, NO, NH2OH, and N2H4) will be generated to adverse the performance of NITRR[95]. Based on the existing studies, remediating NO3- to N2 undergoes a 12 protons and ten electrons transfer process, while NO3- to NH3 involves nine protons and eight electrons transfer[96].
In the initial steps of NITRR, the adsorption of NO3- plays a vital role in influencing its activity and selectivity. Since NO3- can adsorb to active sites via chemisorption (2-O and 1-O) and physisorption,
Figure 7. (A) Possible mechanisms for the adsorption of NO3- on Cu (111); (B) Calculated ΔG(*NO3) and ΔG(*H). This figure is quoted with permission from Wang et al.[97]; (C) Pourbaix diagram of nitrogen species (left), detailed O-end, O-side, N-end, and N-side pathways to form NH3, and the NO-dimer pathway to form N2 (right); (D) Atomic structure of TM/g-CN (left), TM/g-CN limiting potentials for NITRR via the N-end pathway (right). This figure is quoted with permission from Niu et al.[98]; (E) The atomic structure of TM/g-C2N (left), limiting potentials of TM/g-C2N (right). This figure is quoted with permission from Zhu et al.[99].
Unlike the less selective N-N coupling for N2 formation, forming NH3 involves four varied mechanisms
CONCLUSION AND OUTLOOK
Electrochemical NITRR process has been proven to be a green and sustainable approach for NO3- remediation and NH3 production. Designing catalysts with fine electron structure, stability, high atomic utilization, and activity are the primary tasks to improve the performance of NITRR. This review systematically generalizes the recent advances in the field of NITRR dependent on SACs. Apparently, SACs have exhibited great potential in NITRR due to their maximum atom-utilization efficiency, fully exposed active sites, and unique electronic properties. Currently, it remains challenging to achieve large-scale production and commercial applications of SACs. Given that most fundamental research ultimately aims to serve social production, the practical application of SACs-based NITRR still has a long way to meet the standards since current research remains at the laboratory scale.
Inhibiting the aggregation and migration of single atoms
Despite great efforts to enhance the NITRR performances in recent SACs-based strategies, the yield rate of NH3 remains lower due to the limited number of active sites [Table 1]. From an application viewpoint, ensuring a high dispersion of isolated single atoms on SACs is urgent to maximize catalytic activity. However, the atomic scale sites imply the greatly increased free energy of the single atoms. The mass loading of single atoms may induce inevitable migration and aggregation and generate unfavored clusters/nanoparticles under large overpotentials[100]. Once the advantage structure was destroyed, the production of unwanted N-byproducts and H2 would dominate the NITRR process, adversely affecting the stability of SACs. Since the stability of SACs is vital for determining their large-scale applicability and commercial viability, it should be considered as an important indicator during the electrocatalytic process. To improve it, strong binding energy between single-atom sites and substrates is beneficial to prevent the migration or aggregation of single atoms[101]. By optimizing the binding energy through surface modification and changing suitable support materials, migration and aggregation are expected to be effectively suppressed[19,102]. In addition, it is urgent to propose a unified standard to guide the long-term performance testing of SACs. Continuously monitoring catalytic activity, selectivity, energy efficiency, and structural integrity over extended periods is needed to judge the comprehensive performance of SACs-based electrocatalytic systems. Therefore, the structure evolution of SACs is suggested to be investigated during the NITRR process in future studies. The rational use of operando techniques can help us to track the actual active sites and intermediates, which is beneficial to understanding the structure-activity relationship and the in-depth mechanisms to fabricate more efficient SACs.
Summary of catalytic performance of SACs in NITRR
SACs | Operation voltage | FE % | Electrolyte | NH3 yield rate | Stability (cycle) | Durability (h) | Ref. |
BCN@Cu | -0.6 V vs. RHE | 97.37 | KOH + NO3- | 83.95 mg h-1 mgcat-1 | 10 | 16 | [45] |
BCN@Cu/CNT | -0.6V vs. RHE | 95.32 | KOH + NO3- | 172.227 mg h-1 mgcat-1 | 10 | N/A | [46] |
Cu-N-C | -1.5 V vs. SCE | 94 | Na2SO4 + NO3- | 0.25 mg h-1 mgcat-1 | 8 | N/A | [41] |
CuSANPC | -1.1 V vs. RHE | 87.2 | PBS + NaNO3 | 5.204 mg h-1 mgcat-1 | 10 | N/A | [48] |
Cu MNC | -0.64 V vs. RHE | 52.0 | Na2SO4 + NO3- | 92.922 mg h-1 mgcat-1 | 14 | 84 | [49] |
Cu-N3 SACs/NCNT | -0.8 V vs. RHE | 89.64 | KOH + KNO3 | 30.09 mg h-1 mgcat-1 | 10 | N/A | [50] |
Cu-N4/P | -0.6 V vs. RHE | 95.89 | Na2SO4 + NaNO3 | 2.01 mg h-1 mgcat-1 | 6 | N/A | [51] |
Cu SAA | -0.9 V vs. RHE | 87.0 | Na2SO4 + NO3- | 0.3 mg h-1 cm-2 | 20 | ~300 | [52] |
FeN4 SAC | -0.66 V vs. RHE | ~75 | KNO3 + K2SO4 | 20 mg h-1 mgcat-1 | 20 | N/A | [56] |
Fe/g-C3N4 | -0.65 V vs. RHE | 77.3 | Na2SO4 + NO3- | N/A | 8 | N/A | [57] |
FeN2O2-SAC | -0.66 V vs. RHE | ~92 | KNO3 + K2SO4 | 46 mg h-1 mgcat-1 | 15 | N/A | [58] |
Fe-N/P-C | -0.8 V vs. RHE | 90.3 | KOH + KNO3 | 18 mg h-1 mgcat-1 | 20 | N/A | [59] |
S-doped Fe SAC | -0.47 V vs. RHE | 93.9 | Na2SO4 + NaNO3 | N/A | 6 | N/A | [60] |
Fe-TiO2 | -1.4 V vs. RHE | 92.3 | KNO3 + K2SO4 | 137.3 mg h-1 mgcat-1 | 5 | N/A | [61] |
Zn-SAC | -1.0 V vs. RHE | 94.8 | Na2SO4 + NaNO3 | 39.27 mg h-1 mgcat-1 | 20 | N/A | [62] |
Zr-TiON | N/A | 94.8 | Na2SO4 + KNO3 | 11.28 mg h-1 mgcat-1 | 7 | 14 | [63] |
NiSA-BNG | -0.4 V vs. RHE | 95 | Na2SO4 + NaNO3 | 0.168 mg h-1 cm-2 | 5 | N/A | [64] |
Co-SAC | -0.69 V vs. RHE | 92.0 | Na2SO4 + NO3- | 0.4333 mg h-1 mgcat-1 | 5 | N/A | [65] |
PdCu-SAA | -0.6 V vs. RHE | 97.1 | Na2SO4 | 0.26 mg h-1 mgcat-1 | N/A | 10 | [71] |
Rh@Cu | -0.2 V vs. RHE | 93 | Na2SO4 + KNO3 | 21.59 mg h-1 cm-2 | N/A | 30 | [72] |
Ni1Cu-SAA | -0.55 V vs. RHE | ~100 | K2SO4 + NO3- | 5.554 mg h-1 cm-2 | N/A | N/A | [73] |
Au/Cu SAAs | -0.8 V vs. RHE | 99.69 | NaNO3 + Na2SO4 | 3.281 mg h-1 cm-2 | 20 | N/A | [74] |
VCu-Au1Cu SAAs | -0.2 V vs. RHE | 98.7 | KOH + KNO3 | 0.555 mg h-1 cm-2 | 5 | 15 | [75] |
Bi1Pd SAAs | -0.6 V vs. RHE | 99.6 | KOH + NO3− | 33.8 mg h-1 cm-2 | 10 | 20 | [76] |
ISAA In−Pd | -0.6 V vs. RHE | 87.2 | NaNO3 + Na2SO4 | 28.06 mg h-1 mgcat-1 | 20 | 100 | [77] |
Fabrication of SACs with asymmetry active centers
For most inorganic carrier-supported SACs, metal centers are coordinated with four N atoms to form a C4V coordination symmetry. However, recent studies indicate that the symmetrical electronic density distribution shows a weak polar active site, leading to a weak adsorption energy of NO3- and intermediates. As a result, the unwanted intermediates are accumulated in the NITRR system and are adverse to the N-pollutant treatment and NH3 production. The breaking of single-atom symmetry provides a promising approach to adjusting the electronic density of active sites, reducing the energy barrier for forming key intermediates, and strengthening the adsorption energies between actives and N-intermediates. Therefore, the efficient methodologies and in-depth mechanisms for constructing asymmetry active sites urgently need to be investigated based on substrate engineering, heteroatom doping, and defect engineering approaches.
Optimization of costs
It can be stated that the effective dispersion of single atoms on supports brings cost advantages for synthesizing SACs. However, it is theoretically feasible and faces many difficulties in practical operations. Firstly, maintaining the high performance and uniformity of active sites is a great challenge when performing large-scale synthesis of SACs. The surface conditions of employed supports are generally more complex than expected. Since the coordination atoms will greatly affect the electronic structure of the active sites, it is essential to fully consider the impacts of the amplified system on the coordination atoms before large-scale synthesis of SACs, thus avoiding the incorrect expression of active sites. Therefore, scalable technologies are urgently needed to precisely regulate the microenvironment of the catalyst at the atomic level. On the other hand, most SAC synthesis strategies involve support/precursor preparation, pyrolysis, and further washing/pickling steps. The cost issues caused by these complex synthesis processes will be further magnified in the scale-up SAC synthesis process.
Technical aspects for SACs-based NITRR
A deeper understanding of detailed technical aspects can help us achieve a more efficient ammonia synthesis. In addition to the stability of isolated active sites and cost issues, the atom selection, atom loading, and reaction environments (pH values, temperature, local pressure, nitrate concentrations, alkaline cations) are also important in designing efficient SACs. To verify the validity of SACs for further water cleaning applications, it is recommended that tests be conducted under conditions that closely mimic practical applications. Generally, heavy metal ions and organic molecules commonly coexist with nitrates in wastewater, which will induce devastating effects on the single-atom active sites by forming strong bonds with active sites. However, current studies generally focus on improving the performance of SACs, little attention was paid to the influences of these unfavorable factors. In addition to the common indicators, the resistant capability of SACs to heavy metal ions and organic macromolecules is recommended as a benchmark in future studies. Furthermore, after obtaining an optimal SAC, the coating of catalysts on electrodes is another key step to ensure the performance of NITRR. Currently, the most widely employed strategy is to prepare a homogeneous catalyst ink and drop the ink onto the electrode. To ensure the uniformity of coated SACs, it is suggested that an airbrush be employed to disperse the homogeneous catalyst ink on the electrode. Additionally, when considering the scale-up application of SACs-based NITRR systems, energy efficiency is another crucial factor. Current strategies majorly focus on the faradaic efficiency and the yield (corresponding to the current density) of target products, while the working potential of proposed systems has always been overlooked. Since the overpotentials in scale-up applications are often larger than those in laboratory-grade cells, it is necessary to optimize reaction conditions and catalyst design to obtain improved energy efficiencies of catalysts.
Theoretical screening for effective SACs configuration
In addition to the experimental investigations, theoretical prescreening is also a powerful route to optimize the fine structures of SACs. Through the modularized DFT simulations, the adsorption of NO3-, reaction pathways, thermodynamic Gibbs free energy changes, kinetic activation energy and limiting potentials of different active sites can be estimated. On the other hand, identifying the rate-determining step helps clarify the physicochemical origin of NITRR performances. The theoretical prescreening can drastically reduce the expended efforts of time-consuming experimental screenings. Typically, solving the Schrödinger equation can accurately predict various properties of relevant systems, while the DFT simulation can only provide an approximate result in larger models that contain more atoms[103]. The results of DFT simulations strongly depend on the expression of the employed exchange-correlation (ec) functional[104]. In current SACs-based studies, Perdew-Burke-Ernzerhof (PBE) ec functional is the most widely used method within the generalized gradient approximation (GGA)[56,61,62,71]. However, the accuracy of DFT simulations in most SACs-based studies may deviate from reality since they barely considered the effects of solvent environments (solvation and electrolyte), potentials, and local pH values. In water solvent-based electrochemical processes, the hydrogen bonds, dynamic effects, and secondary interactions with specific directionality cannot be ignored. For the potential and local pH values, it is necessary to consider the extra charges caused by protons in water solvent and electrons on the electrode when constructing models. In the NITRR process, the methodology development of DFT simulations should focus on several critical steps and considerations, including functional, basis set, pseudopotentials, integration grids, spin polarization, validation and benchmark, convergence criteria, and error analysis. The DFT simulation of SACs-based NITRR systems can reference the experiences from other known systems. For instance, the explicit water layer and implicit solvation can be an effective strategy for treating solvation in the modeling system.
DECLARATIONS
Authors’ contributions
Proposed the topic of this review: Li Z
Performed literature survey and prepared the manuscript: Li Z, Yang C, Yao L
Collectively discussed and revised the manuscript: Li Z, Xu B, Zhu W
Review, conceptualization, and supervision: Zhu W, Cui Y
Availability of data and materials
Not applicable.
Financial support and sponsorship
This work was supported by the National Natural Science Foundation of China (22176086), National Key R&D Program of China (2022YFC3204004), Natural Science Foundation of Jiangsu Province (BK20210189), State Key Laboratory of Pollution Control and Resource Reuse, the Fundamental Research Funds for the Central Universities (021114380183, 021114380189, 021114380199), the Research Funds from Frontiers Science Center for Critical Earth Material Cycling of Nanjing University, Research Funds for Jiangsu Distinguished Professor, and Carbon Peaking and Carbon Neutrality Technological Innovation Foundation of Jiangsu Province (BE2022861), the Special Basic Research Service for the Central Level Public Welfare Research Institute (GYZX240503), and the Foundation of Key Laboratory of Pulp and Paper Science & Technology of Ministry of Education (No. KF 202206), Qilu University of Technology.
Conflicts of interest
All authors declared that there are no conflicts of interest.
Ethical approval and consent to participate
Not applicable.
Consent for publication
Not applicable.
Copyright
© The Author(s) 2024.
REFERENCES
1. Suryanto BHR, Matuszek K, Choi J, et al. Nitrogen reduction to ammonia at high efficiency and rates based on a phosphonium proton shuttle. Science 2021;372:1187-91.
2. Wang S, Ichihara F, Pang H, Chen H, Ye J. Nitrogen fixation reaction derived from nanostructured catalytic materials. Adv Funct Mater 2018;28:1803309.
3. Chen Z, Dolfing J, Zhuang S, Wu Y. Periphytic biofilms-mediated microbial interactions and their impact on the nitrogen cycle in rice paddies. Eco Environ Health 2022;1:172-80.
4. Wang Y, Zhou W, Jia R, Yu Y, Zhang B. Unveiling the activity origin of a copper-based electrocatalyst for selective nitrate reduction to ammonia. Angew Chem Int Ed 2020;59:5350-4.
5. Rosca V, Duca M, de Groot MT, Koper MT. Nitrogen cycle electrocatalysis. Chem Rev 2009;109:2209-44.
6. Penuelas J, Sardans J. Human-driven global nutrient imbalances increase risks to health. Eco Environ Health 2023;2:246-51.
7. Duca M, Koper MTM. Powering denitrification: the perspectives of electrocatalytic nitrate reduction. Energy Environ Sci 2012;5:9726-42.
8. Du R, Cao S, Peng Y, Zhang H, Wang S. Combined partial denitrification (PD)-Anammox: A method for high nitrate wastewater treatment. Environ Int 2019;126:707-16.
9. Shen J, He R, Han W, Sun X, Li J, Wang L. Biological denitrification of high-nitrate wastewater in a modified anoxic/oxic-membrane bioreactor (A/O-MBR). J Hazard Mater 2009;172:595-600.
10. Zheng W, Zhu L, Yan Z, et al. Self-Activated Ni Cathode for Electrocatalytic Nitrate Reduction to Ammonia: From Fundamentals to Scale-Up for Treatment of Industrial Wastewater. Environ Sci Technol 2021;55:13231-43.
11. Zhang C, Zhang Y, Deng R, et al. Enabling logistics automation in nanofactory: cobalt phosphide embedded metal-organic frameworks for efficient electrocatalytic nitrate reduction to ammonia. Adv Mater 2024:e2313844.
12. Li J, Zhan G, Yang J, et al. Efficient ammonia electrosynthesis from nitrate on strained ruthenium nanoclusters. J Am Chem Soc 2020;142:7036-46.
13. Jia R, Wang Y, Wang C, Ling Y, Yu Y, Zhang B. Boosting selective nitrate electroreduction to ammonium by constructing oxygen vacancies in TiO2. ACS Catal 2020;10:3533-40.
14. Gao Z, Lai Y, Tao Y, Xiao L, Zhang L, Luo F. Constructing well-defined and robust Th-MOF-supported single-site copper for production and storage of ammonia from electroreduction of nitrate. ACS Cent Sci 2021;7:1066-72.
15. Chen G, Yuan Y, Jiang H, et al. Electrochemical reduction of nitrate to ammonia via direct eight-electron transfer using a copper-molecular solid catalyst. Nat Energy 2020;5:605-13.
16. Sun WJ, Ji HQ, Li LX, et al. Built-in electric field triggered interfacial accumulation effect for efficient nitrate removal at ultra-low concentration and electroreduction to ammonia. Angew Chem Int Ed 2021;60:22933-9.
17. Carvalho OQ, Marks R, Nguyen HKK, et al. Role of electronic structure on nitrate reduction to ammonium: a periodic journey. J Am Chem Soc 2022;144:14809-18.
18. Fan X, Liang J, Zhang L, et al. Enhanced electrocatalytic nitrate reduction to ammonia using plasma-induced oxygen vacancies in CoTiO3-x nanofiber. Carbon Neutral 2022;1:6-13.
19. Chen Y, Ji S, Chen C, Peng Q, Wang D, Li Y. Single-atom catalysts: synthetic strategies and electrochemical applications. Joule 2018;2:1242-64.
20. Ding S, Yin L, Lyu Z, et al. Wearable microgrids empowered by single-atom materials. Innova Mater 2023;2:100023.
21. Chakraborty R, K V, Pradhan M, Nayak AK. Recent advancement of biomass-derived porous carbon based materials for energy and environmental remediation applications. J Mater Chem A 2022;10:6965-7005.
22. Wang Y, Su H, He Y, et al. Advanced electrocatalysts with single-metal-atom active sites. Chem Rev 2020;120:12217-314.
23. Zhao J, Xue S, Barber J, Zhou Y, Meng J, Ke X. An overview of Cu-based heterogeneous electrocatalysts for CO2 reduction. J Mater Chem A 2020;8:4700-34.
24. Wei Y, Xia H, Yan W, Zhang J. Recent processing of interaction mechanisms of single metallic atom/clusters in energy electrocatalysis. Energy Mater 2023;3:300033.
25. Gong X, Song P, Han C, Xiao Y, Mei X, Xu W. Heterogeneous single-atom catalysts for energy process: recent progress, applications and challenges. Energy Mater 2023;3:300016.
26. Cheng XF, He JH, Ji HQ, et al. Coordination symmetry breaking of single-atom catalysts for robust and efficient nitrate electroreduction to ammonia. Adv Mater 2022;34:e2205767.
27. Zhu C, Shi Q, Feng S, Du D, Lin Y. Single-atom catalysts for electrochemical water splitting. ACS Energy Lett 2018;3:1713-21.
28. Shang W, Liu W, Cai X, et al. Insights into atomically dispersed reactive centers on g-C3N4 photocatalysts for water splitting. Adv Powder Mater 2023;2:100094.
29. Jiang F, Li Y, Pan Y. Design principles of single-atom catalysts for oxygen evolution reaction: from targeted structures to active sites. Adv Mater 2024;36:e2306309.
30. Zhang J, Tang X, Hong Y, et al. Carbon-based single-atom catalysts in advanced oxidation reactions for water remediation: from materials to reaction pathways. Eco Environ Health 2023;2:47-60.
31. Wan C, Duan X, Huang Y. Molecular design of single-atom catalysts for oxygen reduction reaction. Adv Energy Mater 2020;10:1903815.
32. Li Z, Ma R, Ju Q, et al. Spin engineering of single-site metal catalysts. Innovation 2022;3:100268.
33. Han J, Bian J, Sun C. Recent advances in single-atom electrocatalysts for oxygen reduction reaction. Research 2020;2020:9512763.
34. Lu B, Liu Q, Nichols F, et al. Oxygen reduction reaction catalyzed by carbon-supported platinum few-atom clusters: significant enhancement by doping of atomic cobalt. Research 2020;2020:9167829.
35. Shao Y, Yuan Q, Zhou J. Single-atom catalysts and dual-atom catalysts for CO2 electroreduction: competition or cooperation? Small 2023;19:e2303446.
36. Tang T, Wang Z, Guan J. Achievements and challenges of copper-based single-atom catalysts for the reduction of carbon dioxide to C2+ products. Exploration 2023;3:20230011.
37. Li M, Wang H, Luo W, Sherrell PC, Chen J, Yang J. Heterogeneous single-atom catalysts for electrochemical CO2 reduction reaction. Adv Mater 2020;32:e2001848.
38. Li X, Chen Y, Zhan X, et al. Strategies for enhancing electrochemical CO2 reduction to multi-carbon fuels on copper. Innov Mater 2023;1:100014.
39. Li J, Chen S, Quan F, et al. Accelerated dinitrogen electroreduction to ammonia via interfacial polarization triggered by single-atom protrusions. Chem 2020;6:885-901.
40. Wei X, Liu Y, Zhu X, et al. Dynamic reconstitution between copper single atoms and clusters for electrocatalytic urea synthesis. Adv Mater 2023;35:e2300020.
41. Chen H, Zhang C, Sheng L, et al. Copper single-atom catalyst as a high-performance electrocatalyst for nitrate-ammonium conversion. J Hazard Mater 2022;434:128892.
42. Wang L, Wang D, Li Y. Single-atom catalysis for carbon neutrality. Carbon Energy 2022;4:1021-79.
43. Wang Z, Liu S, Wang M, et al. In situ construction of metal-organic frameworks as smart channels for the effective electrocatalytic reduction of nitrate at ultralow concentrations to ammonia. ACS Catal 2023;13:9125-35.
44. Hu T, Wang C, Wang M, Li CM, Guo C. Theoretical insights into superior nitrate reduction to ammonia performance of copper catalysts. ACS Catal 2021;11:14417-27.
45. Zhao X, Jia X, He Y, et al. Two-dimensional BCN matrix inlaid with single-atom-Cu driven electrochemical nitrate reduction reaction to achieve sustainable industrial-grade production of ammonia. Appl Mater Today 2021;25:101206.
46. Zhao X, Li X, Zhang H, et al. Atomic-dispersed copper simultaneously achieve high-efficiency removal and high-value-added conversion to ammonia of nitrate in sewage. J Hazard Mater 2022;424:127319.
47. Zhu T, Chen Q, Liao P, et al. Single-atom Cu catalysts for enhanced electrocatalytic nitrate reduction with significant alleviation of nitrite production. Small 2020;16:e2004526.
48. Zhao X, Geng Q, Dong F, et al. Boosting the selectivity and efficiency of nitrate reduction to ammonia with a single-atom Cu electrocatalyst. Chem Eng J 2023;466:143314.
49. Xue Y, Yu Q, Ma Q, et al. Electrocatalytic hydrogenation boosts reduction of nitrate to ammonia over single-atom Cu with Cu(I)-N3C1 sites. Environ Sci Technol 2022;56:14797-807.
50. Wang Y, Zhang W, Wen W, et al. Atomically dispersed unsaturated Cu-N3 sites on high-curvature hierarchically porous carbon nanotube for synergetic enhanced nitrate electroreduction to ammonia. Adv Funct Mater 2023;33:2302651.
51. Wang H, Yao Y, Zhan J, et al. P-modified single-atom Cu catalyst boosting electrocatalytic performance of NO3- reduction to NH3. ChemCatChem 2023;15:e202201633.
52. Li P, Liao L, Fang Z, Su G, Jin Z, Yu G. A multifunctional copper single-atom electrocatalyst aerogel for smart sensing and producing ammonia from nitrate. Proc Natl Acad Sci USA 2023;120:e2305489120.
53. Yang J, Qi H, Li A, et al. Potential-driven restructuring of Cu single atoms to nanoparticles for boosting the electrochemical reduction of nitrate to ammonia. J Am Chem Soc 2022;144:12062-71.
54. Nielsen SE. Ammonia synthesis: catalyst and technologies. In: Flank WH, Abraham MA, Matthews MA, editors. Innovations in industrial and engineering chemistry. Washington: American Chemical Society; 2008. pp. 15-39.
55. Yoshino H, Kawase Y. Kinetic modeling and simulation of zero-valent iron wastewater treatment process: simultaneous reduction of nitrate, hydrogen peroxide, and phosphate in semiconductor acidic wastewater. Ind Eng Chem Res 2013;52:17829-40.
56. Wu ZY, Karamad M, Yong X, et al. Electrochemical ammonia synthesis via nitrate reduction on Fe single atom catalyst. Nat Commun 2021;12:2870.
57. Song Q, Li M, Hou X, et al. Anchored Fe atoms for N=O bond activation to boost electrocatalytic nitrate reduction at low concentrations. Appl Catal B Environ 2022;317:121721.
58. Zhang W, Dong H, Zhou L, et al. Fe single-atom catalysts with pre-organized coordination structure for efficient electrochemical nitrate reduction to ammonia. Appl Catal B Environ 2022;317:121750.
59. Xu J, Zhang S, Liu H, et al. Breaking local charge symmetry of iron single atoms for efficient electrocatalytic nitrate reduction to ammonia. Angew Chem Int Ed 2023;62:e202308044.
60. Liu F, Li J, An N, Huang J, Liu X, Li M. Highly active electroreduction of nitrates to ammonia over a zeolitic imidazolium framework-derived Fe single-atom catalyst with sulfur-modified asymmetric active centers. J Hazard Mater 2024;465:133484.
61. Wang Z, Liu S, Zhao X, et al. Interfacial defect engineering triggered by single atom doping for highly efficient electrocatalytic nitrate reduction to ammonia. ACS Mater Lett 2023;5:1018-26.
62. Zhao J, Ren X, Liu X, et al. Zn single atom on N-doped carbon: highly active and selective catalyst for electrochemical reduction of nitrate to ammonia. Chem Eng J 2023;452:139533.
63. Yang L, Wang C, Li Y, et al. Frustrated lewis pairs on Zr single atoms supported N-doped TiO2-x catalysts for electrochemical nitrate reduction to ammonia. Adv Funct Mater 2024:2401094.
64. Ajmal S, Kumar A, Mushtaq MA, et al. Uniting synergistic effect of single-Ni site and electric field of B- bridged-N for boosted electrocatalytic nitrate reduction to ammonia. Small 2024:e2310082.
65. Li J, Li M, An N, et al. Boosted ammonium production by single cobalt atom catalysts with high Faradic efficiencies. Proc Natl Acad Sci USA 2022;119:e2123450119.
66. Wang C, Markovic NM, Stamenkovic VR. Advanced platinum alloy electrocatalysts for the oxygen reduction reaction. ACS Catal 2012;2:891-8.
67. Li S, Dong M, Peng M, et al. Crystal-phase engineering of PdCu nanoalloys facilitates selective hydrodeoxygenation at room temperature. Innovation 2022;3:100189.
68. Zhang L, Dang Y, Zhou X, et al. Direct conversion of CO2 to a jet fuel over CoFe alloy catalysts. Innovation 2021;2:100170.
69. Vandevyvere T, Sabbe MK, Mendes PS, Thybaut JW, Lauwaert J. NiCu-based catalysts for the low-temperature hydrodeoxygenation of anisole: Effect of the metal ratio on SiO2 and γ-Al2O3 supports. Green Carbon 2023;1:170-84.
70. Wang Y, Xu A, Wang Z, et al. Enhanced nitrate-to-ammonia activity on copper-nickel alloys via tuning of intermediate adsorption. J Am Chem Soc 2020;142:5702-8.
71. Du C, Lu S, Wang J, et al. Selectively reducing nitrate into NH3 in neutral media by PdCu single-atom alloy electrocatalysis. ACS Catal 2023;13:10560-9.
72. Liu H, Lang X, Zhu C, et al. Efficient electrochemical nitrate reduction to ammonia with copper-supported rhodium cluster and single-atom catalysts. Angew Chem Int Ed 2022;61:e202202556.
73. Cai J, Wei Y, Cao A, et al. Electrocatalytic nitrate-to-ammonia conversion with ~100% faradaic efficiency via single-atom alloying. Appl Catal B Environ 2022;316:121683.
74. Yin H, Peng Y, Li J. Electrocatalytic reduction of nitrate to ammonia via a Au/Cu single atom alloy catalyst. Environ Sci Technol 2023;57:3134-44.
75. Zhang Y, Chen X, Wang W, Yin L, Crittenden JC. Electrocatalytic nitrate reduction to ammonia on defective Au1Cu (111) single-atom alloys. Appl Catal B Environ 2022;310:121346.
76. Chen K, Ma Z, Li X, Kang J, Ma D, Chu K. Single-atom Bi alloyed Pd metallene for nitrate electroreduction to ammonia. Adv Funct Mater 2023;33:2209890.
77. Xie M, Tang S, Li Z, et al. Intermetallic single-atom alloy In-Pd bimetallene for neutral electrosynthesis of ammonia from nitrate. J Am Chem Soc 2023;145:13957-67.
78. Wu X, Nazemi M, Gupta S, et al. Contrasting capability of single atom palladium for thermocatalytic versus electrocatalytic nitrate reduction reaction. ACS Catal 2023;13:6804-12.
79. Ji S, Chen Y, Wang X, Zhang Z, Wang D, Li Y. Chemical synthesis of single atomic site catalysts. Chem Rev 2020;120:11900-55.
80. Fei H, Dong J, Wan C, et al. Microwave-assisted rapid synthesis of graphene-supported single atomic metals. Adv Mater 2018;30:e1802146.
81. Zhang L, Banis MN, Sun X. Single-atom catalysts by the atomic layer deposition technique. Natl Sci Rev 2018;5:628-30.
82. Qiu HJ, Ito Y, Cong W, et al. Nanoporous graphene with single-atom nickel dopants: an efficient and stable catalyst for electrochemical hydrogen production. Angew Chem Int Ed 2015;54:14031-5.
83. Ali H, Masar M, Guler AC, Urbanek M, Machovsky M, Kuritka I. Heterojunction-based photocatalytic nitrogen fixation: principles and current progress. Nanoscale Adv 2021;3:6358-72.
84. Zhao Y, Shi R, Bian X, et al. Ammonia detection methods in photocatalytic and electrocatalytic experiments: how to improve the reliability of NH3 production rates? Adv Sci 2019;6:1802109.
85. Hodgetts RY, Kiryutin AS, Nichols P, et al. Refining universal procedures for ammonium quantification via rapid 1H NMR analysis for dinitrogen reduction studies. ACS Energy Lett 2020;5:736-41.
86. Li Z, Wang L, Cai Y, Zhang J, Zhu W. Electrochemically reconstructed copper-polypyrrole nanofiber network for remediating nitrate-containing water at neutral pH. J Hazard Mater 2022;440:129828.
87. Wendimu G, Hussen A, Mohan BR. Wax screen-based fabrication of paper devices for the determination of iron in particulates of selected welding fumes in Addis Ababa, Ethiopia. Bull Chem Soc Eth 2024;38:563-76.
88. Sarma BB, Maurer F, Doronkin DE, Grunwaldt JD. Design of single-atom catalysts and tracking their fate using operando and advanced X-ray spectroscopic tools. Chem Rev 2023;123:379-444.
89. Wang X, Wu X, Ma W, et al. Free-standing membrane incorporating single-atom catalysts for ultrafast electroreduction of low-concentration nitrate. Proc Natl Acad Sci USA 2023;120:e2217703120.
90. Liu X, Chen S, Wang H, et al. Lattice confined Ru single sites in hollow Co9S8 polyhedron triggering Co-S-Ru catalytic centers for rechargeable Zn-air battery. Nano Res 2023;16:6701-9.
91. Sun H, Sun L, Liao Y, et al. Atomically imaging single atom catalysts and their behaviors by scanning tunneling microscopy. EES Catal 2023;1:794-809.
92. Zhang L, Li Y, Zhang L, et al. Direct visualization of the evolution of a single-atomic cobalt catalyst from melting nanoparticles with carbon dissolution. Adv Sci 2022;9:e2200592.
93. Li X, Yang X, Zhang J, Huang Y, Liu B. In situ/operando techniques for characterization of single-atom catalysts. ACS Catal 2019;9:2521-31.
94. Zhang N, Zhang G, Shen P, Zhang H, Ma D, Chu K. Lewis acid Fe-V pairs promote nitrate electroreduction to ammonia. Adv Funct Mater 2023;33:2211537.
95. Song W, Yue L, Fan X, et al. Recent progress and strategies on the design of catalysts for electrochemical ammonia synthesis from nitrate reduction. Inorg Chem Front 2023;10:3489-514.
96. Xiang T, Liang Y, Zeng Y, et al. Transition metal single-atom catalysts for the electrocatalytic nitrate reduction: mechanism, synthesis, characterization, application, and prospects. Small 2023;19:e2303732.
97. Wang S, Li L, Hui KS, et al. Non-noble single-atom alloy for electrocatalytic nitrate reduction using hierarchical high-throughput screening. Nano Energy 2023;113:108543.
98. Niu H, Zhang Z, Wang X, Wan X, Shao C, Guo Y. Theoretical insights into the mechanism of selective nitrate-to-ammonia electroreduction on single-atom catalysts. Adv Funct Mater 2021;31:2008533.
99. Zhu S, Qin M, Chen L, et al. Theoretical investigation of electrocatalytic reduction of nitrates to ammonia on highly efficient and selective g-C2N monolayer-supported single transition-metal atoms. J Phys Chem Lett 2023;14:4185-91.
100. Lai J, Zhang Z, Yang X, et al. Opening the black box: Insights into the restructuring mechanism to steer catalytic performance. Innov Mater 2023;1:100020.
101. Bai X, Zhao X, Zhang Y, et al. Dynamic stability of copper single-atom catalysts under working conditions. J Am Chem Soc 2022;144:17140-8.
102. Zhang H, Liu G, Shi L, Ye J. Single-atom catalysts: emerging multifunctional materials in heterogeneous catalysis. Adv Energy Mater 2018;8:1701343.
105. Wang Y, Qin X, Shao M. First-principles mechanistic study on nitrate reduction reactions on copper surfaces: effects of crystal facets and pH. J Catal 2021;400:62-70.
Cite This Article

How to Cite
Li, Z.; Yang C.; Xu B.; Yao L.; Zhu W.; Cui Y. Electrochemically nitrate remediation by single-atom catalysts: advances, mechanisms, and prospects. Energy Mater. 2024, 4, 400046. http://dx.doi.org/10.20517/energymater.2023.147
Download Citation
Export Citation File:
Type of Import
Tips on Downloading Citation
Citation Manager File Format
Type of Import
Direct Import: When the Direct Import option is selected (the default state), a dialogue box will give you the option to Save or Open the downloaded citation data. Choosing Open will either launch your citation manager or give you a choice of applications with which to use the metadata. The Save option saves the file locally for later use.
Indirect Import: When the Indirect Import option is selected, the metadata is displayed and may be copied and pasted as needed.
About This Article
Special Issue
Copyright
Data & Comments
Data
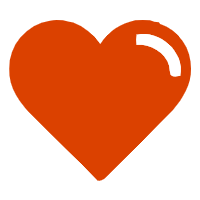
Comments
Comments must be written in English. Spam, offensive content, impersonation, and private information will not be permitted. If any comment is reported and identified as inappropriate content by OAE staff, the comment will be removed without notice. If you have any queries or need any help, please contact us at support@oaepublish.com.