Decorating pore environment via cationic units of covalent organic frameworks for enhancing CO2 reduction reaction
Abstract
The conversion performance for electrocatalytic CO2 reduction reaction (CO2RR) relies on the affinity of CO2 molecules. Ionic covalent organic frameworks (COFs) are promising platforms for CO2RR due to the accessible catalytic sites in the skeleton, high CO2 combination ability and the electronic conductivity. However, most ionic COFs are constructed via pre-functionalization of the monomers or post-modification of the skeleton, encountering incomplete loading or uneven distribution of the active sites. In this work, a cationic porphyrin-based COF using the (3-carboxypropyl)trimethylammonium and Co-porphyrin units is developed through the sub-stoichiometric bottom-up synthesis method to fine-tune the pore environment for modulating the binding ability of CO2. Compared to base COFs, the cationic COFs exhibit improved electronic conductivity, high CO2 adsorption uptakes and enhanced reducibility, further improving the electrocatalytic CO2RR performance. Notably, the cationic COF achieves a high CO selectivity of 93% and a partial current density of 24.6 mA·cm-2. This work not only offers considerable insights for improving the catalytic performance of COFs through the cationic groups but also provides a stoichiometry method to modulate the pore environment.
Keywords
INTRODUCTION
The electrocatalytic CO2 reduction reaction (CO2RR) is a crucial technology for reducing greenhouse gas concentrations and mitigating climate change[1-4]. Nowadays, the molecular catalysts, metallic nanoclusters and single atom catalysts (SACs) were employed to catalyse the CO2RR[5-7]. However, the high energy barrier required to activate inert CO2 and the weak binding interactions between CO2 and catalysts impede catalytic activity and selectivity[8-10]. Consequently, it is imperative to enhance the interaction between CO2 and reactants by modulating CO2 adsorption behavior, which holds great significance for optimizing catalytic activity in CO2RR.
Covalent organic frameworks (COFs), as emerging porous materials due to their high crystallinity, periodic channels, designable structures and robust stability, have attracted considerable attention for molecular adsorption, photo-/electro-catalysis, luminescent sensors and proton transport[11-20]. The porous materials including COFs and metal-organic frameworks (MOFs), constructed with catalytic building blocks, functional units and catalytic sites, have been employed as electrocatalysts towards oxygen reduction reaction (ORR) and oxygen evolution reaction (OER)[21-26]. In 2015, Lin et al. exhibited the porphyrin-COFs for CO2RR using the heterophase strategy[27]. After that, the different dimensional topologies, functional groups, catalytic sites and linkages have been employed to improve the activity and selectivity towards CO2RR. For example, Han et al. reported three-dimensional COFs with abundant exposure active sites for CO2RR[28]. Furthermore, two dioxin-linked COFs were synthesized for improving CO2RR activity through the tuneable electron transfer capacity[29]. However, these catalytic COFs mainly focused on the charge-neutral framework, lacking the exploration of ionic frameworks. The charge-neutral frameworks hinder the electron transferring to the catalytic sites, thus reducing the utilizing efficiency of catalytic sites. Ionic COFs as electrocatalysts possessed the enhanced electronic conductivity and improved electronic transport ability which can facilitate the intermediates transfer behavior. In addition, the abundant active sites promote the adsorption and activation of reactants in the CO2RR[30].
In this work, we incorporated catalytic sites and cationic functional groups into 2D COFs for enhancing the CO2RR selectivity and activity via the bottom-up synthesis method. The optimized COF [a cationic porphyrin-based COF using the (3-carboxypropyl)trimethylammonium (TMA-COF)] was built by adjusting stoichiometric ratios of the monomers using 4,4’,4’’,4’’’-[1,4-phenylenebis(azanetriyl)]- tetrabenzaldehyde (PATA) unit as linkers, [5,10,15,20-tetrakis(4-aminophenyl)porphinato]-cobalt (CoTAPP) as catalytic sites and (3-carboxypropyl)trimethylammonium (TMA) as decorate units. Compared to Co-COF without TMA groups, the optimized COF exhibited higher selectivity and activity due to the enhanced CO2 uptakes. Thus, this work provided not only a modification method for functionalizing COFs but also new insights for the energy conversion.
EXPERIMENTAL
The synthesis scheme and chemical structure of Co-COF and TMA-COF are shown in Scheme 1 and Supplementary Figures 1 and 2. More detailed information, including synthesis methods and materials, is presented in the Supplementary Materials.
RESULTS AND DISCUSSION
To identify the structure of two COFs, the Fourier transform infrared spectroscopy (FTIR) measurement was conducted. Specifically, the peaks at 1,620 cm-1 originated from C=N bonds of the as-synthesized COFs, suggesting the condensation of Schiff reactions [Supplementary Figure 3][31]. The 13C nuclear magnetic resonance (NMR) also exhibited the structure of the prepared COFs. Specifically, the peaks at around
Figure 1. (A) The PXRD profile of the experimentally observed (black), Pawley refined (red), difference (orange), simulated using the AA (blue) and staggered AB (green) stacking modes; (B) Top view of theoretically modeled eclipsed-AA stacking model for the Co-COF
In addition, the simulated PXRD patterns of the TMA-COF demonstrated the diffraction peaks at 5.61°, 9.55°, 10.84° and 20.84°, contributing to the (011), (202), (301) and (001) facets, respectively [Figure 1C]. Notably, the larger layer distance of (001) from TMA-COF than that of Co-COF manifested the electrostatic repulsion from the TMA groups. The Pawly refinement confirmed the AA stacking models of TMA-COF with the Rp = 2.53% and Rwp = 3.41% rather than AB stacking models [Figure 1D and Supplementary
The morphologies of obtained COFs were confirmed through the transmission electron microscopy (TEM) and field-emission scanning electron microscopy (FE-SEM). Specifically, the high-resolution TEM (HRTEM) images of Co-COF and TMA-COF illustrated that the dominant lattices with a d-space of 1.6 and 0.4 nm from (011) and (001) facet, respectively, further indicating the high crystallinity [Figure 1E and F]. In addition, the FE-SEM images showed that the Co-COF illustrated a bar shape that was composed of nanoparticles [Figure 1G]. The TMA-COF also exhibited a bar shape consisting of nanoparticles [Figure 1H]. Moreover, the energy dispersive X-ray spectroscopy (EDX) mappings confirmed the uniform distribution of these elements in COF fragments [Supplementary Figures 7 and 8]. Thermogravimetric analysis (TGA) manifested that the decompositions of Co-COF and TMA-COF reached only 8% and 11% at 550 °C, respectively [Supplementary Figure 9].
The inherent porosities of Co-COF and TMA-COF were analyzed by the N2 sorption behavior. These two obtained COFs exhibited the type-I sorption curve, demonstrating microporous structures [Figure 2A]. The Brunner-Emmet-Teller specific surface areas of Co-COF and TMA-COF were 271.70 and 289.81 m2·g-1 with pore volumes of 0.38 and 0.47 cm3·g-1, respectively. Furthermore, the Co-COF and TMA-COF showed pore size distributions of 1.2 and 1.3 nm, respectively [Figure 2B and C]. Moreover, CO2 adsorption isotherms were conducted to evaluate the CO2 behavior of as-synthesized COFs. The TMA-COF illustrated the CO2 uptakes of 21.33 cm3·g-1 at 298 K, respectively, which was higher than that of Co-COF (18.75 cm3·g-1, Figure 2D). These results indicated that introducing TMA units, which possessed strong CO2 affinity capacity, was beneficial for the CO2RR.
Figure 2. (A) The nitrogen-sorption isotherms at 77 K of Co-COF (black) and TMA-COF (red); The pore distribution curves of (B)
We adopted X-ray photoelectron spectroscopy (XPS) to study the atom and electron states. In particular, the XPS spectra showed peaks of all elements in orbitals (C, N, O and Co, Supplementary Figure 10). The high-resolution Co 2p spectra of these two COFs displayed the peaks at ~781.38 eV assigned to the Co-N coordination from CoTAPP units [Figure 3A]. In addition, the N 1s spectra of the Co-COF showed three peaks at 398.7, 399.8 and 401.5 eV, respectively, originating from iminic N, C=N, and C−N bonds[32-34]. Correspondingly, the N 1s spectra of the TMA-COF demonstrated a new peak at ~402.1 eV attributed to the C−N+ bonds from TMA groups [Figure 3B][33]. Moreover, we adopted the ultraviolet-visible (UV-Vis) spectroscopy to calculate band gaps [Supplementary Figure 11]. In detail, the Tauc plot illustrated the band gaps for Co-COF and TMA-COF were 1.91 and 1.73 eV, respectively, and the narrower bandgap for
Figure 3. The XPS spectra of (A) Co 2p and (B) N 1s for Co-COF and TMA-COF. XPS: X-ray photoelectron spectroscopy; TMA-COF:
Considering these features of COFs, we evaluate the CO2RR performance in 0.5 M KHCO3 under CO2 atmosphere within the applied potential range of -0.5 to -1.0 V vs. reversible hydrogen electrode (RHE). Specifically, the linear sweep voltammetry (LSV) curves suggested that the Co-COF and TMA-COF possessed higher current density in CO2 atmosphere than in Ar atmosphere, revealing their efficient electrocatalytic CO2RR activity [Figure 4A]. Meanwhile, the TMA-COF exhibited higher current density in the range of potentials than that of Co-COF, illustrating the high activity and electronic conductivity. Correspondingly, the Tafel slope of the Co-COF was 238 mV·dec-1, which declined to 189 mV·dec-1 for TMA-COF, demonstrating the high kinetics [Figure 4B]. In addition, we used the gas and liquid chromatography to detect the products in the process of CO2RR. The CO Faradaic efficiencies (FECO) of the Co-COF were 69%, 75%, 78%, 82%, 76%, and 70% with a floating value of ~4% from -0.5 to -1.0 V vs. RHE, respectively [Figure 4C and Supplementary Figure 15]. Additionally, the higher FECO values were observed on the TMA-COF with the increase of CO2 uptakes. Specifically, it delivered FECO of 81%, 86%, 90%, 93%, 86% and 83% with a floating value of ~5%, in the same potential ranges, which are higher than that of other reported COF-based materials [Supplementary Figure 16 and Table 1]. Furthermore, the FECO of carbon nanotubes (CNTs) were below 0.22% from -0.5 to -1.0 V vs. RHE, respectively [Supplementary Figure 17]. In addition to the selectivity, we calculated the partial CO current density (jCO) to investigate the activity of two COFs. The Co-COF and TMA-COF exhibited the highest jCO of 17.9 and 28.5 mA·cm-2 at -1.0 V vs. RHE [Figure 4D]. To confirm the internal catalytic activity, the turnover frequency (TOF) was calculated
Figure 4. (A) LSV curves from -0.5 to -1.0 V in 0.5 M KHCO3 under CO2 atmosphere (line) and Ar atmosphere (dot); (B) Tafel slopes; (C) CO faradaic efficiency; (D) The partial CO current density; (E) The ECSA slopes; (F) The CO current density by the normalized ECSA for Co-COF (black) and TMA-COF (red). LSV: Linear sweep voltammetry; ECSA: electrochemically active surface area; COF: covalent organic framework; TMA-COF: (3-carboxypropyl)trimethylammonium covalent organic framework.
The electrochemical double layer capacitances (Cdl) investigated the catalytic density of active sites through the cyclic voltammetry (CV) measurement [Supplementary Figure 19]. The Cdl values for the Co-COF and TMA-COF were 12.0 and 16.7 mF·cm-2, respectively, indicating the TMA-COF possessed more exposure active sites than Co-COF [Figure 4E]. Furthermore, the electrochemically active surface areas (ECSAs) of obtained COFs were calculated by the ratio of Cdl and Cs, in which the Cs is 0.04 mF cm-2[38]. We normalized CO current densities using jCO per ECSA. Specifically, TMA-COF exhibited higher jCO/ECSA in the range of
The stability of TMA-COF was measured in 0.5 M KHCO3. The activity for CO2RR was well preserved for 30 h [Supplementary Figure 20]. The FECO and the value of j/j0 were over 90%. In addition, the PXRD profiles confirmed the maintained crystallinity [Supplementary Figure 21]. Meanwhile, the XPS spectra of Co 2p and N 1s also proved that the atomic states maintained the coordination after the stability measurement, confirming the reliable stability [Supplementary Figures 22 and 23][39].
CONCLUSIONS
In summary, a catalytic COF with cationic functional groups was developed using the sub-stoichiometric bottom-up synthesis method. The experiments suggested that the combination of catalytic sites and cationic groups can modulate the band structure and CO2 adsorption behavior. This cationic COF illustrated the high CO2RR performance with FECO of 93% at -0.8 V vs. RHE. Thus, constructing ionic structures which can modulate the interaction with guests and regulate the location of charge groups is a prospective way for understanding the catalytic mechanisms, further providing new insights for the energy conversion.
DECLARATIONS
Acknowledgments
Authors acknowledged the support from the PhD thesis from the University of Nottingham Ningbo China.
Authors’ contributions
Made substantial contributions to conception and design of the study and performed data analysis and interpretation: Liu M, Liu G, Xu Q, Zeng G
Performed data acquisition and provided administrative, technical, and material support: Liu M, Liu G,
Availability of data and materials
The raw data supporting the findings of this study are available within this Article and its Supplementary Materials. Further data is available from the corresponding authors upon request.
Financial support and sponsorship
The National Natural Science Foundation of China (52303288, 22075309, 22378413), Science and Technology Innovation Plan of the Science and Technology Commission of Shanghai Municipality (22ZR1470100, 23DZ1202600, 23DZ1201804), the Youth Innovation Promotion Association of Chinese Academy of Sciences (E324441401), Sichuan Province Engineering Technology Research Center of Novel CN Polymeric Materials (CNP-C-240201), and Biomaterials and Regenerative Medicine Institute Cooperative Research Project Shanghai Jiao Tong University School of Medicine (2022LHA09).
Conflicts of interest
All authors declared that there are no conflicts of interest.
Ethical approval and consent to participate
Not applicable.
Consent for publication
Not applicable.
Copyright
© The Author(s) 2025.
Supplementary Materials
REFERENCES
1. Chen, Q.; Wang, X.; Zhou, Y.; et al. Electrocatalytic CO2 reduction to C2+ products in flow cells. Adv. Mater. 2024, 36, e2303902.
2. Lai, W.; Qiao, Y.; Zhang, J.; Lin, Z.; Huang, H. Design strategies for markedly enhancing energy efficiency in the electrocatalytic CO2 reduction reaction. Energy. Environ. Sci. 2022, 15, 3603-29.
3. Li, X.; Yu, J.; Jaroniec, M.; Chen, X. Cocatalysts for selective photoreduction of CO2 into solar fuels. Chem. Rev. 2019, 119, 3962-4179.
4. Lu, M.; Zhang, M.; Liu, J.; et al. Covalent organic framework based functional materials: important catalysts for efficient CO2 utilization. Angew. Chem. Int. Ed. Engl. 2022, 61, e202200003.
5. Ma, W.; He, X.; Wang, W.; Xie, S.; Zhang, Q.; Wang, Y. Electrocatalytic reduction of CO2 and CO to multi-carbon compounds over
6. Xiao, C.; Zhang, J. Architectural design for enhanced C2 product selectivity in electrochemical CO2 reduction using Cu-based catalysts: a review. ACS. Nano. 2021, 15, 7975-8000.
7. Xie, C.; Niu, Z.; Kim, D.; Li, M.; Yang, P. Surface and interface control in nanoparticle catalysis. Chem. Rev. 2020, 120, 1184-249.
8. Yang, P. P.; Gao, M. R. Enrichment of reactants and intermediates for electrocatalytic CO2 reduction. Chem. Soc. Rev. 2023, 52, 4343-80.
9. Zhang, X.; Zhang, Z.; Li, H.; et al. Insight into heterogeneous electrocatalyst design understanding for the reduction of carbon dioxide. Adv. Energy. Mater. 2022, 12, 2201461.
10. Zhang, Z.; Huang, X.; Chen, Z.; et al. Membrane electrode assembly for electrocatalytic CO2 reduction: principle and application. Angew. Chem. Int. Ed. Engl. 2023, 62, e202302789.
11. Guo, L.; Yang, L.; Li, M.; Kuang, L.; Song, Y.; Wang, L. Covalent organic frameworks for fluorescent sensing: recent developments and future challenges. Coord. Chem. Rev. 2021, 440, 213957.
12. Haug, W. K.; Moscarello, E. M.; Wolfson, E. R.; McGrier, P. L. The luminescent and photophysical properties of covalent organic frameworks. Chem. Soc. Rev. 2020, 49, 839-64.
13. Jin, E.; Asada, M.; Xu, Q.; et al. Two-dimensional sp2 carbon-conjugated covalent organic frameworks. Science 2017, 357, 673-6.
14. Li, Z.; Sheng, L.; Wang, H.; et al. Three-dimensional covalent organic framework with ceq topology. J. Am. Chem. Soc. 2021, 143, 92-6.
15. Weng, W.; Guo, J. Chiral covalent organic framework films with enhanced photoelectrical performances. J. Am. Chem. Soc. 2024, 146, 13201-9.
16. Liu, L.; Gong, Y.; Tong, Y.; et al. Imidazole-linked fully conjugated covalent organic framework for high-performance sodium-ion battery. CCS. Chem. 2024, 6, 1255-63.
17. Sun, C.; Sheng, D.; Wang, B.; Feng, X. Covalent organic frameworks for extracting water from air. Angew. Chem. Int. Ed. Engl. 2023, 62, e202303378.
18. Xu, X.; Wu, X.; Xu, K.; Xu, H.; Chen, H.; Huang, N. Pore partition in two-dimensional covalent organic frameworks. Nat. Commun. 2023, 14, 3360.
20. Zhou, T.; Wang, L.; Huang, X.; et al. PEG-stabilized coaxial stacking of two-dimensional covalent organic frameworks for enhanced photocatalytic hydrogen evolution. Nat. Commun. 2021, 12, 3934.
21. Li, Z.; Deng, T.; Ma, S.; et al. Three-component donor-π-acceptor covalent-organic frameworks for boosting photocatalytic hydrogen evolution. J. Am. Chem. Soc. , 2023, 8364-74.
22. Lin, Z.; Liu, S.; Weng, W.; Wang, C.; Guo, J. Photostimulated covalent linkage transformation isomerizing covalent organic frameworks for improved photocatalytic performances. Small 2024, 20, e2307138.
23. Liu, M.; Fu, Y.; Bi, S.; et al. Dimensionally-controlled interlayer spaces of covalent organic frameworks for the oxygen evolution reaction. Chem. Eng. J. 2024, 479, 147682.
24. Zhang, Y.; Zhang, X.; Jiao, L.; Meng, Z.; Jiang, H. L. Conductive covalent organic frameworks of polymetallophthalocyanines as a tunable platform for electrocatalysis. J. Am. Chem. Soc. 2023, 145, 24230-9.
25. Zhu, H. J.; Lu, M.; Wang, Y. R.; et al. Efficient electron transmission in covalent organic framework nanosheets for highly active electrocatalytic carbon dioxide reduction. Nat. Commun. 2020, 11, 497.
26. Pan, Y.; Li, Y.; Nairan, A.; et al. Constructing FeNiPt@C trifunctional catalyst by high spin-induced water oxidation activity for Zn-Air battery and anion exchange membrane water electrolyzer. Adv. Sci. 2024, 11, e2308205.
27. Lin, S.; Diercks, C. S.; Zhang, Y. B.; et al. Covalent organic frameworks comprising cobalt porphyrins for catalytic CO2 reduction in water. Science 2015, 349, 1208-13.
28. Han, B.; Jin, Y.; Chen, B.; et al. Maximizing electroactive sites in a three-dimensional covalent organic framework for significantly improved carbon dioxide reduction electrocatalysis. Angew. Chem. Int. Ed. Engl. 2022, 61, e202114244.
29. Yue, Y.; Cai, P.; Xu, K.; et al. Stable bimetallic polyphthalocyanine covalent organic frameworks as superior electrocatalysts. J. Am. Chem. Soc. 2021, 143, 18052-60.
30. Liu, M.; Xu, Q.; Zeng, G. Ionic covalent organic frameworks in adsorption and catalysis. Angew. Chem. Int. Ed. Engl. 2024, 63, e202404886.
31. Zhang, Q.; Dong, S.; Shao, P.; et al. Covalent organic framework-based porous ionomers for high-performance fuel cells. Science 2022, 378, 181-6.
32. Zhang, M.; Liao, J. P.; Li, R. H.; et al. Green synthesis of bifunctional phthalocyanine-porphyrin cofs in water for efficient electrocatalytic CO2 reduction coupled with methanol oxidation. Natl. Sci. Rev. 2023, 10, nwad226.
33. Song, Y.; Zhang, J. J.; Dou, Y.; et al. Atomically thin, ionic-covalent organic nanosheets for stable, high-performance carbon dioxide electroreduction. Adv. Mater. 2022, 34, e2110496.
34. Baker, C. B. D.; Enache, M.; Küster, K.; et al. Structural transformation of surface-confined porphyrin networks by addition of Co atoms. Chemistry 2021, 27, 12430-6.
35. Yao, N.; Wang, G.; Jia, H.; et al. Intermolecular energy gap-induced formation of high-valent cobalt species in CoOOH surface layer on cobalt sulfides for efficient water oxidation. Angew. Chem. Int. Ed. Engl. 2022, 61, e202117178.
36. Yang, D.; Yu, H.; He, T.; et al. Visible-light-switched electron transfer over single porphyrin-metal atom center for highly selective electroreduction of carbon dioxide. Nat. Commun. 2019, 10, 3844.
37. Huang, Q.; Niu, Q.; Li, X. F.; et al. Demystifying the roles of single metal site and cluster in CO2 reduction via light and electric dual-responsive polyoxometalate-based metal-organic frameworks. Sci. Adv. 2022, 8, eadd5598.
38. Li, N.; Si, D. H.; Wu, Q. J.; Wu, Q.; Huang, Y. B.; Cao, R. Boosting electrocatalytic CO2 reduction with conjugated bimetallic Co/Zn polyphthalocyanine frameworks. CCS. Chem. 2023, 5, 1130-43.
39. Liu, M. Rational design of functional covalent organic frameworks for the oxygen and Carbon Dioxide reduction reactions. PhD thesis, University of Nottingham Ningbo China (UNNC), 2024. Available from: https://research.nottingham.edu.cn/en/studentTheses/rational-design-of-functional-covalent-organic-frameworks-for-the. [Last accessed on 9 Aug 2024]
Cite This Article
How to Cite
Download Citation
Export Citation File:
Type of Import
Tips on Downloading Citation
Citation Manager File Format
Type of Import
Direct Import: When the Direct Import option is selected (the default state), a dialogue box will give you the option to Save or Open the downloaded citation data. Choosing Open will either launch your citation manager or give you a choice of applications with which to use the metadata. The Save option saves the file locally for later use.
Indirect Import: When the Indirect Import option is selected, the metadata is displayed and may be copied and pasted as needed.
About This Article
Special Issue
Copyright
Author Biographies
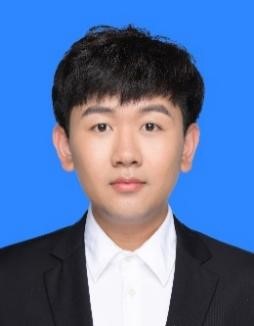
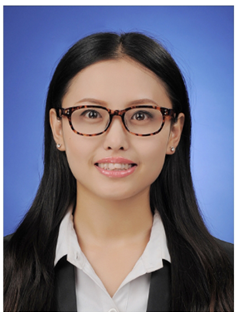
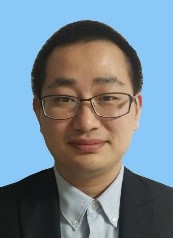
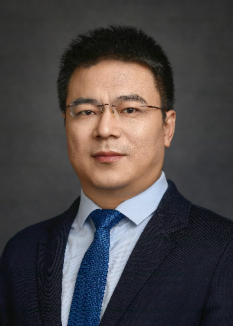
Data & Comments
Data
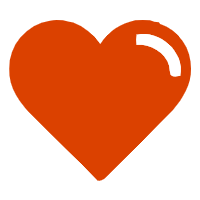
Comments
Comments must be written in English. Spam, offensive content, impersonation, and private information will not be permitted. If any comment is reported and identified as inappropriate content by OAE staff, the comment will be removed without notice. If you have any queries or need any help, please contact us at [email protected].