Post-modified porous aromatic frameworks for carbon dioxide capture
Abstract
A new method was developed for post-modification of porous aromatic framework-1 (PAF-1) with chloride and then amine groups confirmed by different characterizations such as nuclear magnetic resonance (NMR), X-ray photoelectron spectroscopy (XPS), and Fourier transform infrared spectroscopy (FTIR). Compared to PAF-1, the amine-functionalized PAF-1 (PAF-1-NH2) exhibits a 50% improvement in carbon dioxide (CO2) adsorption capacity reaching 42 cm3·g-1 at room temperature and 1 bar, and the uptakes under CO2 concentration in air (4 kPa) and flue gas (150 kPa) also greatly increase. The column breakthrough experiments showed that PAF-1-NH2 can separate CO2 from a simulated flue gas of CO2/N2 (15:85, v/v) indicating its potential applications in post-combustion systems.
Keywords
INTRODUCTION
It has been an international consensus that global warming threatens the sustainable development of human society. The main means to retard the trend is by reducing carbon dioxide (CO2) concentration in the atmosphere[1]. A lot of global authorities have set CO2 emissions reduction plans, and the Chinese government has set the goals of carbon peaking by 2030 and carbon neutrality by 2060. To realize these goals, CO2 capture and sequestration technologies are urgently needed. According to the literature, more than 80% of the CO2 emission comes from the combustion of fossil fuels, and 60% originates from the coal and natural gas-fired power plants[2]. Currently, aqueous amine solutions are mainly used as the adsorbents for CO2 capture in the industry; however, this process suffers from strong equipment corrosion, high regeneration energy input and evaporation pollution/loss of the liquid amines[3].
Gas capture techniques may address excess CO2 emissions. For large-scale use industries, CO2 capture methods include amine scrubbing, calcium cycling from post-combustion, and direct air capture[4]. Amine scrubbing has a higher adsorption capacity, but the loss of adsorption capacity during cycling is greater and more energy is usually required to desorb CO2 due to its higher enthalpy of adsorption. Also, calcium cycling is widely used but faces high extra energy input[5]. Porous materials can overcome the problem of amine scrubbing and calcium cycling, with low energy input and high capacity[6]. At the present stage, research on first-generation capture technologies in China has made significant progress. The physical adsorption method has been successfully and commercially available in 2021[7].
Metal–organic frameworks (MOFs)[8-10] and porous organic frameworks (POFs)[11,12] have been found effective in CO2 capture. The large surface area of porous materials provides various adsorption sites, facilitating effective interaction with CO2 molecules. Furthermore, the reversible adsorption/desorption properties of porous materials allow for multiple cycles of use. Their unique structure and renewable characteristics offer an effective carbon capture and storage (CCS) adsorbent to address challenges associated with greenhouse gas emissions and the climate crisis[13]. Thus, MOFs and POFs are considered alternatives to replace the current liquid amine adsorbents.
Compared with relatively weak coordinate bond-connected MOFs, POFs are combined by stable covalent bonds with improved chemical stability making them available candidates for CO2 capture under harsh conditions[14]. Porous aromatic framework-1 (PAF-1), a star material among the POFs, has an ultra-high Brunauer–Emmett–Teller (BET) surface area and stable structural properties[12]. Thanks to the high chemical stability, different functional groups have been decorated on the PAF-1 and gifting PAF-1 extra properties such as gas storage, separation, uranium capture, and so on[10]. As we know, phenylchloride, which has the potential to be converted to other different functional groups, has yet to be modified in PAF-1. Herein, we developed a previously unreported method to first post-modify phenylchloride groups and then convert them to amine groups on the skeleton of PAF-1, which shows impressive CO2 capture properties. Various approaches were applied to demonstrate the success of the post-modifications, and our research could provide a new way for PAF chlorination and amine functionalization.
EXPERIMENTAL
Materials
All starting materials and solvents, unless otherwise specified, were obtained from Innochem Co. and used without further purification. Tetra(4-bromophenyl)methane (99%) was purchased from Jilin Chinese Academy of Sciences - Yanshen Technology Co., Ltd., and AlCl3 (99+%) was provided by Aladdin.
Synthesis method
Synthesis of PAF-1
Synthesis of PAF-1 as reported[12]: In a glovebox, 1,5-cyclooctadiene (cod, 0.67 mL, 5.3 mmol), bis(1,5-cyclooctadiene)nickel(0) [Ni(cod)2, 1.44 g, 5.2 mmol], 2,2’-bipyridine (2,2’-Bpy, 0.81 g, 5.2 mmol), and anhydrous N,N’-dimethylformamide (DMF) (76 mL) were added to a 250 mL double-neck flask. In another double-neck flask, a solution of tetra(4-bromophenyl)methane (0.63 g, 1 mmol) in DMF (19 mL) was prepared. Both flasks were activated simultaneously at 80 °C for one hour. The activated monomer solution was then slowly transferred into the activated catalyst flask using a syringe. The reaction proceeded at 80 °C for 48 h. After cooling to room temperature, 6 M hydrochloric acid was added, and the solution was stirred for one hour until it turned green with a snowflake-like solid formation. The mixture was filtered, and the white solid obtained was thoroughly washed with water, methanol, and chloroform. The resulting white powder was dried under vacuum at 120 °C to yield a white powder (230 mg, 78% yield).
Synthesis of PAF-1-Cl
The introduction of chlorine into the framework draws on the method of insoluble molecular chlorination[15].
Under an argon atmosphere, PAF-1 (200 mg), AlCl3 (0.2 g, 1.5 mmol), ICl (9.7 g, 60 mmol), and 30 mL of CCl4 were added to a 100 mL flask. The mixture was vigorously stirred and heated to 80 °C, and the reaction was allowed to proceed for two days. After quenching with 50 mL of methanol, the mixture was filtered, and the resulting solid was thoroughly washed with hydrochloric acid, water, methanol, and chloroform. After vacuum drying, a white-gray solid was obtained (277 mg, 96% yield).
Synthesis of PAF-1-CN
Under an inert atmosphere, PAF-1-Cl (60 mg), anhydrous cesium carbonate (1g, 3 mmol), 3-Mercaptopropiononitrile (88 mg, 1 mmol) in anhydrous DMF (5 mL) were heated in a 20 mL single-neck glass bottle at 100 °C for 48 h. After cooling to room temperature, the solid was collected and washed with water, methanol and acetone several times. After drying, 80 mg of light yellow powder with a 87% yield was obtained[16].
Synthesis of PAF-1-COOH
Under an inert atmosphere, PAF-1-Cl (60 mg), anhydrous cesium carbonate (1g, 3 mmol), and thioglycolic acid (93 mg, 1 mmol) in anhydrous DMF (5 mL) were heated in a 20 mL single-neck glass bottle at 100 °C for 48 h. After cooling to room temperature, the solid was collected and washed with water, methanol and acetone several times. After drying, 86 mg of light yellow powder with a 91% yield was obtained[16].
Synthesis of PAF-1-OH
Under an inert atmosphere, PAF-1-Cl (60 mg), anhydrous cesium carbonate (1g, 3 mmol), and 3-Mercapto-1-propanol (93 mg, 1 mmol) in anhydrous DMF (5 mL) were heated in a 20 mL single-neck glass bottle at 100 °C for 48 h. After cooling to room temperature, the solid was collected and washed with water, methanol and acetone several times. After drying, 87 mg of light yellow powder with a 91% yield was obtained[16].
Synthesis of PAF-1-NH-Boc
Under an inert atmosphere, PAF-1-Cl (210 mg), anhydrous cesium carbonate (1g, 3 mmol), and 2-(Boc-amino)ethanethiol (53 mg, 3 mmol) in anhydrous DMF (5 mL) were heated in a 20 mL single-neck glass bottle at 100 °C for 48 h. After cooling to room temperature, the solid was collected and washed with water, methanol and acetone several times. After drying, 336 mg of light yellow powder with a 77% yield was obtained[16].
Synthesis of PAF-1-NH2
Add PAF-1-NH-Boc (230 mg) into a single-neck flask containing 10 mL of concentrated hydrochloric acid and 10 mL of ethyl acetate under an inert gas atmosphere. Stir and heat to reflux for 48 h. Afterward, filter, collect the solid, and thoroughly wash it with water, methanol, and acetone. Subsequently, the collected solid was added into a methanol solution containing 10 wt% sodium hydroxide and vigorously stirred at room temperature for 24 h. Filter and collect the solid, rinse thoroughly with water, and then subject it to Soxhlet extraction using methanol and chloroform as the solvent, respectively, for 6 h each. After drying, 106.8 mg pale yellow solid powder with a 73% yield was obtained[16].
Materials and physical measurements
13C solid nuclear magnetic resonance (NMR) spectra were attained using a Bruker Avance III model
N2 isotherms at 77 K and some other gas isotherms at different temperatures were acquired using an Autosorb absorptiometer (Quantachrome, iQ2). Thermogravimetric analysis (TGA) was performed with a TGA/DSC 1 STARe system at a heating rate of 5 °C/min under an air atmosphere.
Breakthrough experiments
Breakthrough experiments were performed on a mixSorb S (3P instrument), a dynamic gas breakthrough device. A 0.189 g sample of PAF-1-NH2 was loaded onto a stainless-steel column (1 mL volume, 0.45 cm inner diameter). Heat it under a vacuum at 120 °C for 8 h to obtain an activated sample. A CO2/N2 (15:85,
RESULTS AND DISCUSSION
First, a new method that introduced chlorine into a framework is demonstrated [Figure 1A]. The 13C cross-polarization/magic angle spinning NMR (13C CP/MAS NMR) spectrum and XPS analysis confirmed the successful introduction of chlorine elements onto the framework of PAF-1. The solid-state NMR spectrum peaks of PAF-1 were assigned according to the reported literature [Figure 1][12]. 13C NMR spectra of
Figure 1. Schematic diagram of the post-modification process. (A) The new post-modified method for PAF-1; (B) 13C CP/MAS NMR spectrum of PAF-1 and PAF-1-Cl; (C and D) XPS C 1s and Cl 2p spectrum of PAF-1 and PAF-1-Cl. PAF-1: Porous aromatic framework-1; CP/MAS: cross-polarization/magic angle spinning; NMR: nuclear magnetic resonance; XPS: X-ray photoelectron spectroscopy.
In this study, different functional groups are modified by PAF-1-Cl; comprehensive validation of the successful progression of the entire process is evidenced through FTIR spectra. This research involved a series of functional modifications of PAF-1, including PAF-1-CN, PAF-1-COOH and PAF-1-OH [Figure 2]. The characteristic vibrational peaks corresponding to these three materials are displayed in Supplementary Figures 2-4. Their physical adsorption characterizations are shown in Supplementary Figures 5 and 6. In order to solve the problem of CO2 capture, we designed and synthesized post-modified PAF-1 containing amino groups that were commonly known in this application. As depicted in Figure 3A, PAF-1-Cl exhibits a distinctive new peak at 745 cm-1, characteristic of C–Cl bonds, which diminishes in the next post-synthetic step where -Cl and subsequently engages in a continuous reaction with 2-(Boc-amino)ethanethiol. At the same time, PAF-1-NH-Boc displays new peaks at 1,721 and 1,170 cm-1, which were assigned to the C=O and C–N stretch in the Boc group. After the deprotection, the resulting PAF-1-NH2 shows the disappearance of the peaks at 1,721 and 1,170 cm-1. Meanwhile, the -NH2 peak shows at ~3,400 nm-1, which is direct evidence of the successful modification of PAF-1-NH2. Furthermore, the TGA results [Supplementary Figure 5] indicate that all these compounds showed high thermal stability. However, the weight of PAF-1-NH-Boc decreases by 22% at around 250 °C due to the decomposition of the Boc group[18]. This means that PAF-1-Cl contains one -NH-Boc group in each unit on average. Supplementary Figure 6 shows the scanning electron microscope (SEM) images with no marked change after modification. A transmission electron microscopy (TEM) image and the energy-dispersive X-ray spectroscopy (EDS) mapping images of PAF-1-NH2 [Figure 3B-E] exhibited the uniform distribution of S and N elements, implying the success of the post-modification.
Figure 2. Different functional groups modified methods for PAF-1-Cl. PAF-1: Porous aromatic framework-1.
Figure 3. (A) FTIR spectra of PAF-1 (black), PAF-1-Cl (red), PAF-1-NH-Boc (blue) and PAF-1-NH2 (green); (B) The TEM image of PAF-1-NH2; (C-E) EDS maps of S, N and corresponding to (C). FTIR: Fourier transform infrared spectroscopy; PAF-1: porous aromatic framework-1; TEM: transmission electron microscopy; EDS: energy-dispersive X-ray spectroscopy.
The N2 and CO2 adsorption isotherm of PAF-1-CN, PAF-1-COOH and PAF-1-OH were shown in Supplementary Figures 7 and 8. Apart from that, the N2 sorption isotherm measurements were conducted on PAF-1, PAF-1-Cl, PAF-1-NH-Boc, and PAF-1-NH2 at 77 K, revealing BET surface areas of 4,580, 1,077, 139, and 1,002 m2·g-1, respectively [Figure 4A and Supplementary Figure 9]. Simultaneously, the pore width decreases from 1.42 nm (PAF-1) to 1.08 nm (PAF-1-NH2) [Supplementary Figure 10]. The nitrogen adsorption data and the change in the specific surface area suggest that the specific surface area of chlorinated PAF-1 decreases due to not only the larger chlorine atoms occupying the pores but also the increased weight of the chlorine atoms, leading to a drastic reduction in adsorption per unit mass (calculation details are in Supplementary Materials). Post-modification of amino groups with protecting Boc groups further decreases the specific surface area to about 100 m2·g-1, indicating nearly complete pore blockage to nitrogen molecules due to the large molecular size of the Boc. This means that it is difficult for N2 to enter the pores of PAF-1-NH-Boc. In this case, the BET surface area of PAF-1-NH-Boc is much less than that of PAF-1-Cl. Subsequent removal of the amino groups restores the specific surface area to about 1,000 m2·g-1, confirming the successful removal of protective groups and comparable specific surface area to PAF-1-Cl. These changes in specific surface area affirm the success of this post-modified PAF. Meanwhile, the N2 adsorption capacities of PAF-1 and PAF-1-NH2 at 273 and 298 K are shown in Supplementary Figure 12.
Figure 4. Gas absorption and desorption of PAF-1 and its derivations. (A) N2 uptake at 77 K; (B) CO2 uptake of PAF-1 and its derivations at 273 K; (C) CO2 uptake of PAF-1 and its derivations at 298 K; (D) CO2 Qst of PAF-1 and PAF-1-NH2. PAF-1: Porous aromatic
Figure 4B and C depicts the single-component CO2 adsorption isotherms of PAF-1 and its modified derivatives. PAF-1 and PAF-1-Cl both have relatively low uptakes of 1.1 and 0.82 mmol·g-1 at 1 bar and
Specifically, at 273 K, PAF-1-NH2 exhibits a CO2 adsorption capacity of 3.09 mmol·g-1, slightly decreasing to 1.91 mmol at 298 K. This represents a 50% enhancement in CO2 adsorption compared to PAF-1. Importantly, at 4 kPa (relevant to post-combustion capture from natural gas flue), 298 K[18], PAF-1-NH2 demonstrates a substantial increase in CO2 adsorption at 5.824 cm3·g-1 (0.26 mmol·g-1), marking a 6.5-fold enhancement over PAF-1 (0.89 cm3·g-1, 0.04 mmol·g-1). At 15 kPa (pertinent to post-combustion capture from coal flue gas)[20], PAF-1-NH2 shows a significant increase in CO2 uptake at 13.4 cm3·g-1 (0.6 mmol·g-1), representing a 3.3-fold improvement compared to PAF-1 (3.98 cm3·g-1, 0.18 mmol·g-1). These substantial enhancements in CO2 uptake provide compelling evidence for the efficacy of incorporating aliphatic amines into PAFs, suggesting their promising potential as sorbents for efficient post-combustion CO2 capture. Additionally, the CO2 isothermal desorption curve for PAF-1-NH2 exhibits no obvious hysteresis loop. The calculated CO2 adsorption calorific values of PAF-1 and PAF-1-NH2 are 16 and 44 kJ·mol-1, respectively
Based on the CO2 and N2 adsorption isotherms at 273 and 298 K of PAF-1 and PAF-1-NH2, the CO2/N2 selectivity of porous materials could be calculated using the ideal adsorption solution theory (IAST) method. As shown in Figure 5A, The CO2/N2 selectivity of PAF-1 was only 5. With the addition of the amine, the selectivity of PAF-1-NH2 for CO2/N2 was about 163, 33 times higher than that of the original material. Compared with other porous organic polymers in Figure 5B, PAF-1-NH2 possesses a high enthalpy of CO2 adsorption and an impressive separation selectivity [Supplementary Table 1]. The adsorption-desorption cycle of the material is critical for the use of industrial capture, and after five cycles, there was little change in CO2 capacity [Figure 5C]. The results show that PAF-1-NH2 is a promising adsorptive separation material for CO2 separation from industrial flue gas. To examine the practical separation potential of PAF-1-NH2, the transient breakthrough experiments were conducted, in which a CO2/N2 (15:85, v/v) gas mixture flow over a fixed-bed column tightly filled with PAF-1-NH2 samples by a flow of 10 mL·min-1 at 298 K and 1 bar. As shown in Figure 5D, N2 elutes first without detectable CO2, whereas CO2 breaks out after a remarkably long retention time (412 s·g-1), unveiling outstanding CO2 separation performance at 298 K and 1 bar. The calculated CO2 capture capacity based on a single breakthrough experiment is 0.461 mmol·g-1 in accord with the CO2 isotherm.
Figure 5. Separate ability of PAF-1-NH2. (A) CO2/N2 selectivity of PAF-1 and PAF-1-NH2; (B) CO2/N2 selectivity and CO2 Qst comparison with other POPs; (C) CO2 cycles adsorption of PAF-1-NH2; (D) Breakthrough curves of PAF-1-NH2 for a CO2/N2 (15:85, v/v) gas mixture with a total flow of 10 mL·min-1 at 298 K and 1 bar. PAF-1: Porous aromatic framework-1; POPs: porous organic polymers.
CONCLUSIONS
We reported a new approach for functionalizing PAF materials, i.e., chloro-substituted PAF-1 and further converting to amino-functionalized PAF-1. XPS, 13C NMR and FTIR confirm the successful post-modification of different groups such as -Cl and -NH2. This method can also modify PAFs with various functional groups such as -SH and -CHO, thus adapting PAFs to various application scenarios. The CO2 adsorption-desorption isotherms and CO2/N2 dynamic breakthrough of PAF-1-NH2 were characterized, and we also observed that PAF-1-NH2 owns excellent performance in CO2 capture for dealing with post-combustion gases.
DECLARATIONS
Authors’ contributions
Conception: Jia J, Zhu G
Planned the study, analyzed the data, and wrote the manuscript: Wang Z, Jia J
Synthesis and analytical discussions: Jiang L, Zhang Y
SEM and TEM characterization: Han Q, Wang Q
Availability of data and materials
The data is available in the supplementary information section of the submitted manuscript.
Financial support and sponsorship
The authors are grateful for financial support from the National Natural Science Foundation of China (Grant Nos. 22131004 and U21A20330) and the ‘‘111’’ project (No. B18012).
Conflicts of interest
Zhu G served as an editorial member of Chemical Synthesis but was not involved in the editorial process of the work, while the other authors have declared that they have no conflicts of interest.
Ethical approval and consent to participate
Not applicable.
Consent for publication
Not applicable.
Copyright
© The Author(s) 2024.
Supplementary Materials
REFERENCES
1. Yin Y, Kang X, Han B. Two-dimensional materials: synthesis and applications in the electro-reduction of carbon dioxide. Chem Synth 2022;2:19.
2. Choi S, Drese JH, Jones CW. Adsorbent materials for carbon dioxide capture from large anthropogenic point sources. ChemSusChem 2009;2:796-854.
3. Hack J, Maeda N, Meier DM. Review on CO2 capture using amine-functionalized materials. ACS Omega 2022;7:39520-30.
4. Zhai R, Zhang L, Gu M, et al. A review of phosphorus structures as CO2 reduction photocatalysts. Small 2023;19:e2207840.
5. Hanifa M, Agarwal R, Sharma U, Thapliyal P, Singh L. A review on CO2 capture and sequestration in the construction industry: emerging approaches and commercialised technologies. J CO2 Util 2023;67:102292.
6. Abdelhamid HN. Removal of carbon dioxide using zeolitic imidazolate frameworks: adsorption and conversion via catalysis. Appl Organomet Chem 2022;36:e6753.
7. Zhang X, Li Y, Ma Q, Liu L. Development of carbon capture, utilization and storage technology in china. Chin J Eng Sci 2021;23:70.
8. Li H, Eddaoudi M, O’Keeffe M, Yaghi OM. Design and synthesis of an exceptionally stable and highly porous metal-organic framework. Nature 1999;402:276-9.
9. Jiang L, Jia J, Ma Y, Tian Y, Zou X, Zhu G. Metal–carbon bond metal-organic frameworks with permanent porosity. Chem 2024;10:557-66.
10. Jia J, Sun F, Fang Q, et al. A novel low density metal-organic framework with pcu topology by dendritic ligand. Chem Commun 2011;47:9167-9.
11. Côté AP, Benin AI, Ockwig NW, O’Keeffe M, Matzger AJ, Yaghi OM. Porous, crystalline, covalent organic frameworks. Science 2005;310:1166-70.
12. Ben T, Ren H, Ma S, et al. Targeted synthesis of a porous aromatic framework with high stability and exceptionally high surface area. Angew Chem Int Ed Engl 2009;48:9457-60.
13. Siegelman RL, Kim EJ, Long JR. Porous materials for carbon dioxide separations. Nat Mater 2021;20:1060-72.
15. Dong R, Pfeffermann M, Skidin D, et al. Persulfurated coronene: a new generation of “sulflower”. J Am Chem Soc 2017;139:2168-71.
16. Legru A, Verdirosa F, Hernandez JF, et al. 1,2,4-Triazole-3-thione compounds with a 4-ethyl alkyl/aryl sulfide substituent are broad-spectrum metallo-β-lactamase inhibitors with re-sensitization activity. Eur J Med Chem 2021;226:113873.
17. Pełech I, Narkiewicz U, Moszyński D, Pełech R. Simultaneous purification and functionalization of carbon nanotubes using chlorination. J Mater Res 2012;27:2368-74.
18. Han X, Zhou Z, Wang K, et al. Crystalline polyphenylene covalent organic frameworks. J Am Chem Soc 2024;146:89-94.
19. Jiang W, Duan L, Qiao J, et al. Novel star-shaped host materials for highly efficient solution-processed phosphorescent organic light-emitting diodes. J Mater Chem 2010;20:6131-7.
20. Raganati F, Miccio F, Ammendola P. Adsorption of carbon dioxide for post-combustion capture: a review. Energy Fuels 2021;35:12845-68.
Cite This Article

How to Cite
Download Citation
Export Citation File:
Type of Import
Tips on Downloading Citation
Citation Manager File Format
Type of Import
Direct Import: When the Direct Import option is selected (the default state), a dialogue box will give you the option to Save or Open the downloaded citation data. Choosing Open will either launch your citation manager or give you a choice of applications with which to use the metadata. The Save option saves the file locally for later use.
Indirect Import: When the Indirect Import option is selected, the metadata is displayed and may be copied and pasted as needed.
About This Article
Special Issue
Copyright
Author Biographies
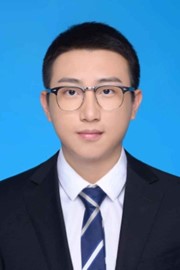
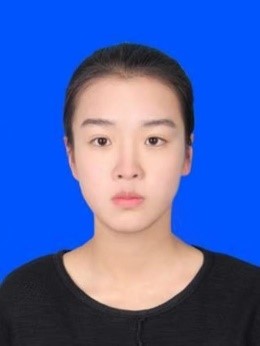
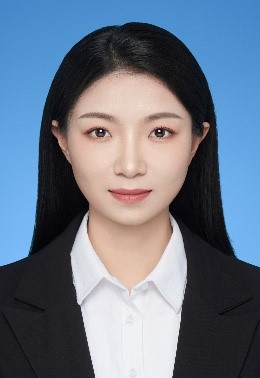
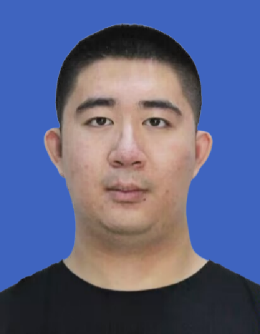
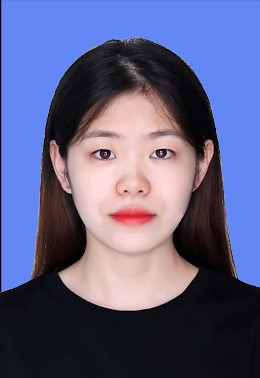
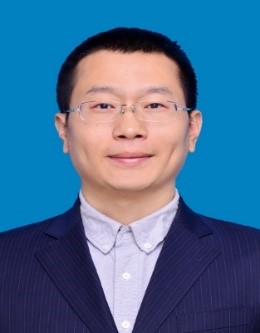
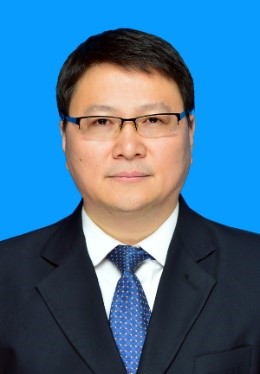
Comments
Comments must be written in English. Spam, offensive content, impersonation, and private information will not be permitted. If any comment is reported and identified as inappropriate content by OAE staff, the comment will be removed without notice. If you have any queries or need any help, please contact us at [email protected].