A 3D ordered hierarchical crystalline porous organic salt for large-sized enzyme immobilization
Abstract
Crystalline porous organic salts (CPOSs) are an emerging class of promising materials with intrinsic highly polar nanoconfined microporosity. However, their single microporous structure greatly hinders their development in the field of catalysis and adsorption. Constructing a hierarchical porous structure can effectively reduce the mass transport resistance, thus expanding the scope of their applications. Herein, we report the synthesis of a three-dimensional (3D) ordered macro-/microporous hierarchical CPOS (HCPOS-1) using a template-assisted approach for the first time. The as-synthesized HCPOS-1 prepared from a polystyrene colloidal crystal template showcases a 3D ordered macroporous structure while also preserving the microporous structure. The 3D ordered macroporous structure in such a hierarchical structure, together with its hydrophilic surface, endows HCPOS-1 with the ability to immobilize large-sized enzymes through physical adsorption under mild conditions. The resulting catalase/HCPOS-1 showcases a high enzyme immobilization capacity and avoids undesired conformational changes of enzymes during the immobilization process, thus exhibiting excellent catalytic activity for the decomposition of hydrogen peroxide.
Keywords
INTRODUCTION
Crystalline porous organic salts (CPOSs), as a novel class of porous organic materials constructed from organic acids and organic bases via ionic bonding, have aroused significant attention and made notable advancements in diverse fields[1,2]. Although the micropores in their structure endow them with an impressive micropore surface area, the single microporous structure impedes mass transfer and severely restricts their further application in catalysis, adsorption, and various other fields[3-15]. Hierarchical porous structures composed of interconnecting pores with different sizes widely exist in nature, which maximize the efficiency of substance transport and reaction rate, and ensure efficient chemical reactions in organisms[16-18]. Introducing hierarchical porous structures into CPOSs is conducive to reducing mass transfer resistance and accommodating larger molecules than micropores, thus expanding the scope of application for CPOSs[2]. Nevertheless, synthesizing CPOSs with hierarchical porous structures remains an enormous challenge due to the rapid reaction rate and flexible bonding motifs of organic acids and bases.
The template-assisted technique represents a highly effective strategy for fabricating exquisite hierarchical porous materials[19]. By employing this method, the pore size of resulting hierarchical porous materials can be finely tuned over a wide range, depending on the dimensions of the chosen template. Furthermore, compared to alternative approaches such as elongated ligands, this technique offers a more streamlined and less challenging synthetic process for fabricating hierarchical porous materials[20]. Currently, the template-assisted method has gained significant traction in fabricating hierarchical porous materials and has been successfully applied to fabricating hierarchical crystalline porous materials such as metal- (MOFs) and covalent organic frameworks (COFs)[21-26]. In 2018, Shen et al. first prepared three-dimensional (3D) ordered micro-/microporous MOF single crystals utilizing polystyrene (PS) colloidal crystal as the sacrifice template[21]. More recently, Liu et al. successfully employed this methodology to prepare ordered macro-/microporous single crystals of COFs under solvothermal conditions with aniline as the modulator[26]. Notably, selecting the synthesis conditions requires careful consideration, as the solvent should be incompatible with the template and the reaction conditions should have negligible impact on the structural integrity of the template. The synthesis conditions employed for CPOSs are relatively mild compared to the harsh synthesis conditions (e.g., high temperature, pressure, etc.) typically associated with COFs and MOFs. Furthermore, the solvents predominantly employed in fabricating CPOSs are primarily aqueous or alcoholic in nature, imparting minimal influence on the template. Consequently, the template-assisted method holds great promise for facilitating the preparation of CPOSs featuring hierarchical porous structures. Notably, the elaborately engineered hierarchical CPOSs (HCPOS) may have a potential application in the adsorption of large guest molecules.
Enzymes, as natural catalysts, facilitate highly efficient and specific catalysis under mild conditions[27,28]. Immobilizing enzymes within porous materials represents a potent approach to expand their applications[29-43]. Nevertheless, the size of enzymes typically exceeds the micropore dimensions of porous materials. This discrepancy becomes even more pronounced for large-sized enzymes, presenting persistent challenges for their immobilization. Although in-situ encapsulation can immobilize large-sized enzymes within porous materials, the interactions between the building blocks, organic solvents, and harsh synthesis conditions during the immobilization process often lead to decreased or even loss of enzyme activity[44,45]. Alternatively, the physical adsorption strategy, which allows for enzyme immobilization under mild conditions, emerges as a favorable option[46,47]. Hierarchical porous materials with an appropriate pore size endow them with the ability to immobilize large-sized enzymes. The large pore ensures sufficient space for effective mass transfer and the preservation of enzyme’s freedom, while the small pore guarantees a high surface area for adsorbing more substrates. Compared to hierarchical MOFs and COFs, CPOSs with a hierarchical porous structure have hydrophilic surfaces and no toxic metal ions, rendering them an ideal platform for immobilizing large-sized enzymes. To the best of our knowledge, there have been no reports on the immobilization of large-sized enzymes using HCPOSs.
Herein, we report, for the first time, a template-assisted method for preparing a 3D ordered HCPOS.
EXPERIMENTAL
Materials
Styrene and polyvinylpyrrolidone (K29-32, Mw = 58,000, PVP) were purchased from J&K Scientific (Beijing, China). Chlorosulfonic acid (ClSO3H) was purchased from Energy Chemical (Shanghai, China). DACH was purchased from TCI (Shanghai, China). CAT (EC 1.11.1.6 from bovine liver, 2,000-5,000 units/mg protein) was purchased from Sigma-Aldrich (USA). Fluorescein isothiocyanate (FITC), ammonium iron (II) sulfate and 2-methyl-imidazole (HmIM) were purchased from Bide (Shanghai, China). Zn(OAc)2·2H2O was purchased from Dongnan Chemical (Jinhua, China). Bradford assay kit was purchased from Bioss (Shanghai, China). H2O2 (1 M) was purchased from Beyotime (Shanghai, China). Sorbitol was purchased from Macklin (Shanghai, China). Xylenol Orange sodium salt and potassium persulfate (K2S2O8, KPS) were purchased from Mreda (Beijing, China). Tris-HCl buffer (50 mM, pH 8) and phosphate-buffered saline (PBS) buffer (50 mM, pH 7) were obtained from Yuanye (Shanghai, China). All chemicals and solvents were used as received without further purification.
Synthesis of 3D ordered polystyrene colloidal crystal template
The PS template was prepared according to the previous literature reported by Shen et al.[21]. Styrene was first washed thoroughly with 10 wt.% NaOH solution and deionized water to remove the stabilizer. Then, 32.5 mL styrene was added to 250.0 mL deionized water containing 1.25 g PVP. After bubbling with nitrogen for 30 min, the mixture was stirred at 75 oC for 30 min. Subsequently, 25.0 mL of K2S2O8
The formation of 3D ordered PS colloidal crystal template from monodisperse PS was easily achieved by filtration. The colloidal dispersions of PS were carefully poured onto a filter funnel pre-setted with three conventional filter papers under vacuum. After three days, burly filter cakes were formed, which were further washed with deionized water and dried at 80 oC overnight.
Synthesis of CPOS-1-Cryst
CPOS-1 crystals (CPOS-1-Cryst) were prepared according to previous literature with some modifications[3]. First, 21.4 mg TSPM (0.025 mmol) was dissolved in 3.0 mL methanol and 1.0 mL H2O. Then, 5.7 mg DACH (0.050 mmol) was dissolved in 3.0 mL methanol and 1.0 mL H2O. The obtained solutions were filtered and mixed. The mixture was allowed to stand at room temperature overnight to obtain CPOS-1-Cryst as a light-yellow crystal (13.4 mg, 71.3%).
Synthesis of HCPOS-1
First, 5.7 mg DACH (0.050 mmol) was dissolved in 1.5 mL absolute methanol. Next, a 2.0-3.0 g PS template was immersed in the solution of DACH for 4 h. Then, the methanol was removed under vacuum at room temperature for 2 h to obtain DACH@PS. In a separate step, 21.4 mg TSPM (0.025 mmol) was dissolved in 6.0 mL methanol and 2.0 mL H2O. The DACH@PS was immersed in the TSPM solution overnight. Subsequently, vacuum at room temperature for 3 h, and then immersed in tetrahydrofuran (THF) for 24 h to remove the PS template. Finally, the resultant was washed by THF and dried at 150 oC for 8 h to give HCPOS-1 (12.2 mg, 64.7%).
Synthesis of CPOS-6
CPOS-6 was prepared according to the previous literature[6]. First, 10.3 mg TSPM (0.012 mmol) was dissolved in 1.2 mL methanol. Next, 7.2 mg tetrakis(4-amidinophenyl)methane hydrochloride (TAmPM, 0.012 mmol) was dissolved in 1.45 mL H2O. The obtained solutions were filtered and mixed. The mixture was allowed to stand at room temperature overnight to obtain CPOS-6 as a light-yellow crystal (6.8 mg, 46.6%).
Synthesis of HCPOS-6
First, 20.6 mg TSPM (0.024 mmol) was dissolved in 1.5 mL methanol. Next, 4.0-5.0 g PS template was immersed in the TSPM solution for 4 h; then, the methanol was removed under vacuum at room temperature for 2 h to obtain TSPM@PS. Subsequently, 14.4 mg TAmPM (0.025 mmol) was dissolved in
Synthesis of ZIF-8
Zeolitic imidazolate framework (ZIF)-8 was synthesized according to a previous report with some modifications[48]. First, 526.0 mg Zn(OAc)2·2H2O (2.4 mmol) was dissolved in 20.0 mL H2O, and 3.15 g HmIM (38.4 mmol) was dissolved in 20.0 mL H2O. Then, the above-obtained solutions were mixed. The reaction mixture was left under stirred conditions at room temperature. After 24 h, the precipitate was collected by filtration and dried at 80 oC overnight (0.56 g, 96.1%).
Synthesis of fluorescein-tagged CAT/HCPOS-1
Fluorescein-tagged CAT (FCAT) was prepared according to a previous report with some modifications[49]. First, 10.0 mg CAT was dissolved in carbonate-bicarbonate aqueous buffer solution (0.1 M, pH 9, 2.0 mL). Then, 5.0 mg FITC was dissolved in 1.0 mL dimethyl sulfoxide (DMSO). After mixing CAT and FITC solution and stirring for 2 h without light at room temperature, the unreacted FITC was removed by washing with ethanol and ultrapure water. Subsequently, 20.0 mg HCPOS-1 was added into FCAT solution and stirred overnight; the FCAT/HCPOS-1 was obtained by centrifugation at 18,000 rpm for 3 min and then washed, sonicated, and centrifuged in ultrapure water three times.
Synthesis of CAT/HCPOS-1
First, 3.0 mg HCPOS-1 was added into 2.0 mL CAT aqueous solution (2 mg·mL-1, centrifugated at
Synthesis of CAT/ZIF-8
First, 3.0 mg ZIF-8 was added into 2.0 mL CAT aqueous solution (2 mg·mL-1, centrifugated at 12,000 rpm for 10 min); the mixture was left under static conditions at 8 oC for 6 h. CAT/ZIF-8 was obtained by filtration.
Characterizations
1H nuclear magnetic resonance (NMR) was recorded on Bruker 400 MHz NMR spectrometer. Tecan infinite 200Pro microplate reader was used to record the catalytic process. The powder X-ray diffraction (PXRD) patterns of all materials were collected on a Bruker D8 Advance instrument with Cu-Kα radiation operating at 40 kV and 40 mA, and recorded from 2o to 40o (2θ) at a scan rate of 0.1 time/step. The thermogravimetric analysis (TGA) data was collected on a Netzsch STA 449 C analyzer at a heating rate of 10 oC·min-1 ranging from 30-900 °C under air atmosphere. Fourier transform infrared (FT-IR) spectra of all materials were obtained on a Nicolet iS50 FT-IR spectrometer using approximately 3 mg of ground sample. In this work, an attenuated total reflectance (ATR) sampling tool was used for the FT-IR test. Sixty-four scans were recorded over the range of 4,000-400 cm-1. Gas adsorption isotherms were obtained on ASAP 2020. Approximately 50 mg of the sample was placed into a glass analysis tube and degassed under dynamic vacuum for 8 h at 150 oC before measurement. The carbon dioxide (CO2) adsorption-desorption analysis was conducted at 273 K. The confocal laser scanning microscopy (CLSM) image was collected on Carl Zeiss LSM 880 under an excitation of 488 nm. Scanning electron microscopy (SEM) was performed on Carl Zeiss Gemini SEM 300 with 5 kV and Hitachi S-4800 SEM with 10 kV. Transmission electron microscopy (TEM) was performed on JEOL JEM2100 at an operating voltage of 200 kV. Samples were dispersed with ethanol and dripped onto a 200 mesh copper net. The ultraviolet-visible (UV-vis) spectra were attained with a Shimadzu UV-2700 spectrometer. The circular dichroism (CD) measurements were obtained using a MOS-500 Spectrometer. Samples were measured using a 1.0 cm path length cuvette. The spectra were recorded from 220 to 260 nm with a path length of 2.0 nm. Water contact angle was measured using a SINDIN SDC-100 optical contact angle tension measuring instrument (Dongguan). Surface hydrophobicity/hydrophilicity was evaluated by measuring the contact angles between sessile water droplets and materials.
Determination of the immobilization capacity of enzyme
The enzyme amounts in solution before and after the immobilization measured with the Bradford method[50,51].
Enzyme immobilization capacity = (C1 - C2)V/m
Here, C1 and C2 (mg/mL) mean the CAT concentration before and after immobilization; V (mL) is the volume of the CAT aqueous solution, and m (mg) represents the mass of material initially added to the solution.
Catalytic performance of CAT/HCPOS-1 and CAT/ZIF-8 Composites
The ferrous oxidation in the xylenol orange (FOX) assay was applied to quantify the H2O2 concentration[52,53]. The concentration of the commercial H2O2 standard was first calculated spectrophotometrically at 240 nm with ε = 39.4 M-1·cm-1. The desired mass of CAT/HCPOS-1 or CAT/ZIF-8 was added into Tris-HCl buffer (50 mM, pH 8, 500 μL). Thereafter, 500 μL H2O2 stock solution was added. At different time intervals, 50 μL aliquots of the mixtures were sampled and mixed with 950 μL of FOX reagent in a centrifugal tube and then incubated for at least 30 min at room temperature. After incubation, the samples were centrifuged. The absorbance value at 560 nm for the supernatant was recorded to calculate the H2O2 concentration.
Storage stability of CAT/HCPOS-1
The storage stability of CAT/HCPOS-1 was determined by incubating them at -20 oC, and tested the activity every seven days with 1.5 mg CAT/HCPOS-1 and 0.25 mM H2O2.
RESULTS AND DISCUSSION
A 3D ordered HCPOS was meticulously synthesized employing a template-assisted method in the presence of a PS colloid crystal template, as illustrated in Figure 1. Considering the stability of CPOS and the influence of synthetic solvents on the template during the synthesis process, CPOS-1, self-assembled from TSPM and DACH through ionic bonding in methanol, was chosen as an example for the HCPOS synthesis. In order to fabricate a magnificent HCPOS-1, we synthesized large CPOS-1 crystals, denoted as CPOS-1-Cryst, according to the previous report with some modifications[3]. CPOS-1-Cryst was synthesized from TSPM and DACH by an acid-base reaction in a mixture of methanol/H2O (3/1, v/v). Adding H2O slows the reaction rate between TSPM and DACH and prolongs the precipitation time of crystals, thereby forming large CPOS-1 crystals
Figure 1. Schematic illustration of the synthesis of 3D ordered macro-/microporous of HCPOS-1. 3D: Three-dimensional; HCPOS: hierarchical crystalline porous organic salt.
The morphology of both PS colloidal crystal and HCPOS-1 was revealed by SEM. The PS nanospheres are regularly arranged under the driving force of gravity and differential pressure during the filtration[54], forming PS colloidal crystal with an opal structure. As illustrated in Figure 2A and B, the PS colloidal crystal showcases a close-packed face center cubic (FCC) structure, where the formed PS monolayer matches well with the (111) facet of FCC crystal structure and adopts an ABC stacking mode. The PS nanospheres within this PS colloidal crystal possess a uniform size of approximately 250 nm and are densely stacked together to create well-defined voids [Figure 2B]. These voids offer sufficient space for the adsorption of precursors and the synthesis of CPOS-1. HCPOS-1 displays a block-like morphology, boasting an obvious 3D ordered macroporous structure that impeccably complements the PS colloidal crystal template [Figure 2C]. The voids in the original PS colloidal crystal template are completely filled by CPOS-1, forming an anti-opal structure upon the removal of the PS template. The regular arrangement of macropores also agrees well with the (111) crystal face in the PS colloid crystal. Additionally, the macropores possess an approximate size of 250 nm, which also aligns well with the size of the PS colloidal crystal template [Figure 2D]. TEM further confirms the macroporous structure of HCPOS-1. As shown in Figure 2E and F, numerous uniformly arranged and well-ordered macropores within HCPOS-1 are distributed across multiple layers and form a distinct anti-opal structure, which is complementary to the opal structure of PS colloidal crystal template. The diameter of the macropores is approximately 250 nm [Figure 2F], in accordance with the SEM images of HCPOS-1 and the PS colloidal crystal template, further proving the successful preparation of macro-/microporous HCPOS-1.
Figure 2. (A) SEM image of PS colloidal crystal template and corresponding FCC crystal structure perpendicular to (111) facet (inset); (B) Zoom-in view of the SEM image, size of PS nanosphere (yellow circle) and voids formed by PS nanospheres (red triangle); (C and D) SEM images of HCPOS-1, size of macropore (yellow cycle) and anti-opal structure formed by CPOS-1 (red triangle); (E and F) TEM images of HCPOS-1, size of macropore (yellow circle) and anti-opal structure formed by CPOS-1 (red triangle). SEM: Scanning electron microscopy; PS: polystyrene; FCC: face center cubic; HCPOS: hierarchical crystalline porous organic salt; CPOS: crystalline porous organic salt; TEM: transmission electron microscopy.
The PXRD was employed to investigate the crystallinity and phase purity of HCPOS-1 [Figure 3A]. HCPOS-1 exhibits excellent crystallinity, with the PXRD pattern being identical to that of CPOS-1, indicating the preservation of crystalline nature of CPOS-1. Additionally, no excess peaks in PXRD pattern verify the phase purity of HCPOS-1. To further confirm the porous nature of HCPOS-1, CO2 adsorption experiments were performed at 273 K [Figure 3B]. The CO2 adsorption capacity of HCPOS-1 is 33.9 cm3·g-1, similar to that of CPOS-1 (38.9 cm3·g-1). Subsequently, the pore size distribution of HCPOS-1 was calculated using density functional theory (DFT). The predominant pore size of HCPOS-1 is centered at approximately 5.0 Å [Supplementary Figure 7], which agrees well with the theoretical pore size of CPOS-1 (~5.3 Å, Supplementary Figure 8). These results further support the existence of microporous structures in addition to the 3D ordered macropore in HCPOS-1. FT-IR spectra showcase a broad band at 2,950 cm-1 for HCPOS-1, which is associated with the stretching vibrations of primary ammonium cations. Additionally, the bands at 1,593 and 1,537 cm-1 correspond to the bending vibrations of primary ammonium cations. The stretching bands at 1,182 and 1,037 cm-1 can be attributed to the asymmetric and symmetric stretching of sulfonate anions, respectively [Figure 3C]. These results are consistent with the FT-IR spectrum of CPOS-1, indicating the retention of CPOS-1 structure during the synthesis. Additionally, HCPOS-1 exhibits a thermal stability up to 320 oC, showing no significant differences in TGA plot compared to CPOS-1 [Figure 3D]. Thus, it can be inferred that the stability of CPOS-1 remains.
Figure 3. (A) PXRD patterns of HCPOS-1 and CPOS-1; (B) CO2 sorption isotherms of HCPOS-1 and CPOS-1 at 273 K; (C) FT-IR spectra of HCPOS-1 and CPOS-1; (D) TGA curves of HCPOS-1 and CPOS-1. PXRD: Powder X-ray diffraction; HCPOS: hierarchical crystalline porous organic salt; CPOS: crystalline porous organic salt; FT-IR: Fourier transform infrared; TGA: thermogravimetric analysis.
To validate this approach, CPOS-6, synthesized from TSPM and tetrakis(4-amdinophenyl)methane (TAmPM) in a methanol-water mixture, was selected as an additional example for HCPOS synthesis. As shown in Supplementary Figures 9 and 10, both the PXRD pattern and FT-IR spectra show no significant changes, indicating the preservation of the CPOS-6 structure. The SEM image further confirms the successful synthesis of HCPOS-6, displaying distinct macropores with an average diameter of 250 nm
To gain further insight into the properties of macropores in HCPOS-1, enzyme immobilization was performed. As we know, the high concentration and toxicity of H2O2 can cause undesired damage to normal tissues[55]. CAT, an iron-heme enzyme, plays a crucial role in reducing the H2O2 content in the body and catalyzing reactions outside the body[56]. However, the dimensions of CAT (9.7 × 9.2 × 6.7 nm3) tend to surpass the pore size of microporous materials, presenting a formidable challenge in its immobilization[35]. HCPOS-1, featuring large enough macropore and numerous sulfonic acid and amino functional groups on the surface, can adsorb enzymes through van der Waals forces, ionic interactions and hydrogen bonding[57], making it an ideal platform for CAT immobilization through physical adsorption. This strategy is straightforward and avoids using organic solvents and harsh conditions, thus preserving the activity of enzymes to a maximum.
To evaluate the CAT immobilization performance of HCPOS-1, we employed a simple and effective method. HCPOS-1 was immersed in an aqueous solution of CAT for six hours, forming CAT-loaded HCPOS-1 (denoted as CAT/HCPOS-1, Figure 4A). The immobilization capacity of CAT in HCPOS-1 was determined by the Bradford assay [Supplementary Figure 12][50,51]. As illustrated in Figure 4B, the value of immobilization capacity is calculated to be as high as 336.9 mg·g-1, which surpasses that in CPOS-1
Figure 4. (A) Immobilization process of CAT within HCPOS-1 and decomposition process of H2O2 catalyzed by CAT/HCPOS-1; (B) Immobilization capacity of HCPOS-1 and CPOS-1; (C) CLSM image of FITC tagged CAT/HCPOS-1; (D) PXRD patterns of CAT/HCPOS-1 and HCPOS-1; (E) Solid state UV-vis spectra of CAT/HCPOS-1, HCPOS-1 and CAT; (F) Catalytic performance of CAT/HCPOS-1 and free CAT; (G) Storage stability of CAT/HCPOS-1. CAT: Catalase; HCPOS: hierarchical crystalline porous organic salt; CPOS: crystalline porous organic salt; CLSM: confocal laser scanning microscopy; FITC: fluorescein isothiocyanate; PXRD: powder X-ray diffraction; UV-vis: ultraviolet-visible.
The FT-IR spectrum of CAT/HCPOS-1 shows a new stretching band at 1,700-1,600 cm-1 corresponding to the amide I region of CAT [Supplementary Figure 14], demonstrating that the presence of CAT within HCPOS-1 and the secondary structure of CAT has no obvious change. The new absorption peak at 408 nm in the solid-state UV-vis spectrum [Figure 4E and Supplementary Figure 15] is attributed to the Soret absorption band (π-π*) due to the iron-heme cofactor in CAT[58]. Moreover, the similarity in the Soret absorption peaks between free CAT and CAT/HCPOS-1 indicates that the immobilization process does not induce any significant changes in the active site of CAT[59]. CD spectra of free and recovered CAT show no significant change, indicating that the primary conformation is preserved during the immobilization process [Supplementary Figure 16].
The coexistence of microporous and macroporous structures within HCPOS-1 endows it with a large surface area and reduced mass transfer resistance, which are beneficial for enzyme catalysis. Subsequently, the catalytic activity of CAT/HCPOS-1 for the decomposition of H2O2 was investigated. FOX reagent was used to determine the concentration of H2O2 [Supplementary Figure 17][52,53]. Figure 4F reveals the remarkable catalytic activity of CAT/HCPOS-1 with a rate of 6.0 μM·s-1, approximately 67% of the activity of the free enzyme (8.9 μM·s-1), indicating that the enzyme structure is not significantly disrupted during the immobilization process. The hydrophilic nature of the surface plays a crucial role in maintaining the active conformation of enzymes[60,61]. In the case of HCPOS-1, numerous polar functional groups on the surface impart HCPOS-1 with a hydrophilic surface, as confirmed by water contact angle measurement
CONCLUSIONS
In summary, a template-assisted approach has been used to fabricate HCPOSs for the first time. CPOS-1, with a hierarchical porous structure, has been successfully synthesized in the presence of a PS colloid crystal template. HCPOS-1 exhibits 3D ordered macroporous and microporous structures. The 3D ordered macroporous structure in HCPOS-1 greatly enhances the diffusion of enzymes and increases the contact area for enzyme immobilization. Consequently, HCPOS-1 exhibits an impressive capacity for immobilizing the CAT enzyme, up to 336.9 mg·g-1. Furthermore, the hydrophilic surface of HCPOS-1 avoids the drastic conformation changes of CAT during the immobilization process, endowing CAT/HCPOS-1 with high catalytic activity. Our results provide a highly effective strategy for constructing hierarchical CPOSs specifically designed to immobilize large-sized enzymes. This current work also offers valuable insights for immobilizing other biomacromolecules, viruses, and even cells, thus opening up new avenues for future research in this field.
DECLARATIONS
Authors’ contributions
Conceived the idea and led the project: Su BL, Ben T
Designed the samples and performed experiments: Wang J, Xing G
Analyzed the data and wrote the paper: Wang J, Xing G, Chen LH, Zhu W, Su BL, Ben T
Gave the experimental guidance and advice: Zhao Y, Zhou J, Song B, Chen LH, Zhu W
All authors contributed to the general discussion.
Availability of data and materials
The detailed materials and methods in the experiment were listed in the Supplementary Materials. Other raw data that support the findings of this study are available from the corresponding author upon reasonable request.
Financial support and sponsorship
This study was supported by the National Key R&D Program of China (2021YFA1200400), the National Natural Science Foundation of China (No. 91956108, No. 22001191), and the Natural Science Foundation of Zhejiang Province (No. LZ22B010001).
Conflicts of interest
Ben T and Chen LH are Junior Editorial Board members of Chemical Synthesis, and Su BL is Editor-in-Chief of Chemical Synthesis. The other authors declare that there are no conflicts of interest.
Ethical approval and consent to participate
Not applicable.
Consent for publication
Not applicable.
Copyright
© The Author(s) 2024.
Supplementary Materials
REFERENCES
2. Yu S, Xing GL, Chen LH, Ben T, Su BL. Crystalline porous organic salts: from micropore to hierarchical pores. Adv Mater 2020;32:e2003270.
3. Xing G, Yan T, Das S, Ben T, Qiu S. Synthesis of crystalline porous organic salts with high proton conductivity. Angew Chem Int Ed Engl 2018;57:5345-9.
4. Xing G, Bassanetti I, Bracco S, et al. A double helix of opposite charges to form channels with unique CO2 selectivity and dynamics. Chem Sci 2019;10:730-6.
5. Zhao Y, Fan C, Pei C, et al. Colossal negative linear compressibility in porous organic salts. J Am Chem Soc 2020;142:3593-9.
6. Zhang S, Fu J, Das S, Ye K, Zhu W, Ben T. Crystalline porous organic salt for ultrarapid adsorption/desorption-based atmospheric water harvesting by dual hydrogen bond system. Angew Chem Int Ed Engl 2022;61:e202208660.
7. Xing G, Zhang S, Zhu W, Ben T. Reply to the correspondence on “Crystalline porous organic salt for ultrarapid adsorption/desorption-based atmospheric water harvesting by dual hydrogen bond system”. Angew Chem Int Ed Engl 2023;62:e202215074.
8. Yamamoto A, Hirukawa T, Hisaki I, Miyata M, Tohnai N. Multifunctionalized porosity in zeolitic diamondoid porous organic salt: selective adsorption and guest-responsive fluorescent properties. Tetrahedron Lett 2013;54:1268-73.
9. Ami T, Oka K, Tsuchiya K, Tohnai N. Porous organic salts: diversifying void structures and environments. Angew Chem Int Ed Engl 2022;61:e202202597.
10. Sei H, Oka K, Sotome H, Miyasaka H, Tohnai N. Cage-like sodalite-type porous organic salts enabling luminescent molecule’s incorporation and room-temperature phosphorescence induction in air. Small 2023;19:e2301887.
11. Brekalo I, Deliz DE, Barbour LJ, Ward MD, Friščić T, Holman KT. Microporosity of a guanidinium organodisulfonate hydrogen-bonded framework. Angew Chem Int Ed Engl 2020;59:1997-2002.
12. O'Shaughnessy M, Padgham AC, Clowes R, et al. Controlling the crystallisation and hydration state of crystalline porous organic salts. Chemistry 2023;29:e202302420.
13. Karmakar A, Illathvalappil R, Anothumakkool B, et al. Hydrogen-bonded organic frameworks (HOFs): a new class of porous crystalline proton-conducting materials. Angew Chem Int Ed Engl 2016;55:10667-71.
14. Comotti A, Bracco S, Yamamoto A, et al. Engineering switchable rotors in molecular crystals with open porosity. J Am Chem Soc 2014;136:618-21.
15. Bracco S, Miyano T, Negroni M, et al. CO2 regulates molecular rotor dynamics in porous materials. Chem Commun 2017;53:7776-9.
16. Chen LH, Li Y, Su BL. Hierarchy in materials for maximized efficiency. Natl Sci Rev 2020;7:1626-30.
17. Chen LH, Sun MH, Wang Z, Yang W, Xie Z, Su BL. Hierarchically structured zeolites: from design to application. Chem Rev 2020;120:11194-294.
18. Zhou J, Fan W, Wang Y, Xie Z. The essential mass transfer step in hierarchical/nano zeolite: surface diffusion. Natl Sci Rev 2020;7:1630-2.
19. Stein A, Wilson BE, Rudisill SG. Design and functionality of colloidal-crystal-templated materials--chemical applications of inverse opals. Chem Soc Rev 2013;42:2763-803.
20. Li P, Chen Q, Wang TC, et al. Hierarchically engineered mesoporous metal-organic frameworks toward cell-free immobilized enzyme systems. Chem 2018;4:1022-34.
21. Shen K, Zhang L, Chen X, et al. Ordered macro-microporous metal-organic framework single crystals. Science 2018;359:206-10.
22. Sun M, Zhou J, Hu Z, et al. Hierarchical zeolite single-crystal reactor for excellent catalytic efficiency. Matter 2020;3:1226-45.
23. Yao W, Chen J, Wang Y, et al. Nitrogen-doped carbon composites with ordered macropores and hollow walls. Angew Chem Int Ed Engl 2021;60:23729-34.
24. Yao W, Hu C, Zhang Y, et al. Hierarchically ordered porous carbon with atomically dispersed cobalt for oxidative esterification of furfural. Ind Chem Mater 2023;1:106-16.
25. Zhao X, Pachfule P, Li S, et al. Macro/microporous covalent organic frameworks for efficient electrocatalysis. J Am Chem Soc 2019;141:6623-30.
26. Liu T, Zhao Y, Song M, et al. Ordered macro-microporous single crystals of covalent organic frameworks with efficient sorption of iodine. J Am Chem Soc 2023;145:2544-52.
27. Schoemaker HE, Mink D, Wubbolts MG. Dispelling the myths - biocatalysis in industrial synthesis. Science 2003;299:1694-7.
28. Straathof AJJ. Transformation of biomass into commodity chemicals using enzymes or cells. Chem Rev 2014;114:1871-908.
29. Liang W, Wied P, Carraro F, et al. Metal-organic framework-based enzyme biocomposites. Chem Rev 2021;121:1077-129.
30. Huang S, Chen G, Ouyang G. Confining enzymes in porous organic frameworks: from synthetic strategy and characterization to healthcare applications. Chem Soc Rev 2022;51:6824-63.
31. Chen W, Vázquez-gonzález M, Zoabi A, Abu-reziq R, Willner I. Biocatalytic cascades driven by enzymes encapsulated in metal–organic framework nanoparticles. Nat Catal 2018;1:689-95.
32. An H, Song J, Wang T, et al. Metal-organic framework disintegrants: enzyme preparation platforms with boosted activity. Angew Chem Int Ed Engl 2020;59:16764-9.
33. Lu J, Wu JK, Jiang Y, et al. Fabrication of microporous metal-organic frameworks in uninterrupted mesoporous tunnels: hierarchical structure for efficient trypsin immobilization and stabilization. Angew Chem Int Ed Engl 2020;59:6428-34.
34. Liang J, Bin Zulkifli MY, Yong J, et al. Locking the ultrasound-induced active conformation of metalloenzymes in metal-organic frameworks. J Am Chem Soc 2022;144:17865-75.
35. Tang J, Liu J, Zheng Q, et al. In-situ encapsulation of protein into nanoscale hydrogen-bonded organic frameworks for intracellular biocatalysis. Angew Chem Int Ed Engl 2021;60:22315-21.
36. Chen G, Huang S, Shen Y, et al. Protein-directed, hydrogen-bonded biohybrid framework. Chem 2021;7:2722-42.
37. Wied P, Carraro F, Bolivar JM, Doonan CJ, Falcaro P, Nidetzky B. Combining a genetically engineered oxidase with hydrogen-bonded organic frameworks (HOFs) for highly efficient biocomposites. Angew Chem Int Ed Engl 2022;61:e202117345.
38. Chen G, Tong L, Huang S, Huang S, Zhu F, Ouyang G. Hydrogen-bonded organic framework biomimetic entrapment allowing non-native biocatalytic activity in enzyme. Nat Commun 2022;13:4816.
39. Xing C, Mei P, Mu Z, et al. Enhancing enzyme activity by the modulation of covalent interactions in the confined channels of covalent organic frameworks. Angew Chem Int Ed Engl 2022;61:e202201378.
40. Feng M, Niu Z, Xing C, et al. Covalent organic framework based crosslinked porous microcapsules for enzymatic catalysis. Angew Chem Int Ed Engl 2023;62:e202306621.
41. Liang J, Ruan J, Njegic B, et al. Insight into bioactivity of in-situ trapped enzyme-covalent-organic frameworks. Angew Chem Int Ed Engl 2023;62:e202303001.
42. Zhang Y, Xing C, Mu Z, et al. Harnessing self-repairing and crystallization processes for effective enzyme encapsulation in covalent organic frameworks. J Am Chem Soc 2023;145:13469-75.
43. Liu H, Zhou Y, Guo J, et al. Reticular synthesis of highly crystalline three-dimensional mesoporous covalent-organic frameworks for lipase inclusion. J Am Chem Soc 2023;145:23227-37.
44. Liu S, Sun Y. Co-encapsulating cofactor and enzymes in hydrogen-bonded organic frameworks for multienzyme cascade reactions with cofactor recycling. Angew Chem Int Ed Engl 2023;62:e202308562.
45. Freund R, Zaremba O, Arnauts G, et al. The current status of MOF and COF applications. Angew Chem Int Ed Engl 2021;60:23975-4001.
46. Kandambeth S, Venkatesh V, Shinde DB, et al. Self-templated chemically stable hollow spherical covalent organic framework. Nat Commun 2015;6:6786.
47. Sun Q, Fu CW, Aguila B, et al. Pore environment control and enhanced performance of enzymes infiltrated in covalent organic frameworks. J Am Chem Soc 2018;140:984-92.
48. Liang W, Ricco R, Maddigan NK, et al. Control of structure topology and spatial distribution of biomacromolecules in protein@ZIF-8 biocomposites. Chem Mater 2018;30:1069-77.
49. Feng Y, Hu H, Wang Z, et al. Three-dimensional ordered magnetic macroporous metal-organic frameworks for enzyme immobilization. J Colloid Interface Sci 2021;590:436-45.
50. Bradford MM. A rapid and sensitive method for the quantitation of microgram quantities of protein utilizing the principle of protein-dye binding. Anal Biochem 1976;72:248-54.
51. Liang W, Flint K, Yao Y, et al. Enhanced bioactivity of enzyme/MOF biocomposite via host framework engineering. J Am Chem Soc 2023;145:20365-74.
52. Ou P, Wolff SP. A discontinuous method for catalase determination at ‘near physiological’ concentrations of H2O2 and its application to the study of H2O2 fluxes within cells. J Biochem Biophys Methods 1996;31:59-67.
53. Li M, Qiao S, Zheng Y, et al. Fabricating covalent organic framework capsules with commodious microenvironment for enzymes. J Am Chem Soc 2020;142:6675-81.
54. Stein A, Schroden RC. Colloidal crystal templating of three-dimensionally ordered macroporous solids: materials for photonics and beyond. Curr Opin Solid St M 2001;5:553-64.
55. Liu X, Yan Z, Zhang Y, et al. Two-dimensional metal-organic framework/enzyme hybrid nanocatalyst as a benign and self-activated cascade reagent for in vivo wound healing. ACS Nano 2019;13:5222-30.
56. Fita I, Rossmann MG. The NADPH binding site on beef liver catalase. Proc Natl Acad Sci U S A 1985;82:1604-8.
57. Jesionowski T, Zdarta J, Krajewska B. Enzyme immobilization by adsorption: a review. Adsorption 2014;20:801-21.
58. Liang W, Carraro F, Solomon MB, et al. Enzyme encapsulation in a porous hydrogen-bonded organic framework. J Am Chem Soc 2019;141:14298-305.
59. Dong X, Fan Y, Yang P, et al. Ultraviolet-visible (UV-Vis) and fluorescence spectroscopic investigation of the interactions of ionic liquids and catalase. Appl Spectrosc 2016;70:1851-60.
60. Liang W, Xu H, Carraro F, et al. Enhanced activity of enzymes encapsulated in hydrophilic metal-organic frameworks. J Am Chem Soc 2019;141:2348-55.
Cite This Article
How to Cite
Wang, J.; Xing G.; Zhao Y.; Zhou J.; Song B.; Chen L. H.; Zhu W.; Su B. L.; Ben T. A 3D ordered hierarchical crystalline porous organic salt for large-sized enzyme immobilization. Chem. Synth. 2024, 4, 34. http://dx.doi.org/10.20517/cs.2024.24
Download Citation
Export Citation File:
Type of Import
Tips on Downloading Citation
Citation Manager File Format
Type of Import
Direct Import: When the Direct Import option is selected (the default state), a dialogue box will give you the option to Save or Open the downloaded citation data. Choosing Open will either launch your citation manager or give you a choice of applications with which to use the metadata. The Save option saves the file locally for later use.
Indirect Import: When the Indirect Import option is selected, the metadata is displayed and may be copied and pasted as needed.
About This Article
Special Issue
Copyright
Data & Comments
Data
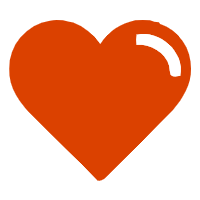
Comments
Comments must be written in English. Spam, offensive content, impersonation, and private information will not be permitted. If any comment is reported and identified as inappropriate content by OAE staff, the comment will be removed without notice. If you have any queries or need any help, please contact us at support@oaepublish.com.