Atomically dispersed trimetallic oxygen electrocatalysts for advancing rechargeable zinc-air battery
Abstract
Developing efficient non-precious metal catalysts for oxygen electrocatalysis is crucial for advancing renewable energy storage systems such as rechargeable Zn-air batteries. Nitrogen-doped carbon (M-N-C) materials with atomically dispersed metal sites, particularly Fe-N-C, exhibit remarkable activity for the oxygen reduction reaction (ORR); however, their performance in the oxygen evolution reaction (OER) remains unsatisfactory. In this work, we present the fabrication of Fe, Co, and Ni trimetallic single-atom catalysts, which exhibit outstanding bifunctional catalytic performance. Using ZIF-8 and phytic acid as chelating agents, we achieved uniform dispersion of Fe, Co, and Ni atoms within a porous carbon matrix, preventing metal agglomeration and enhancing catalytic performance. The Fe30Co30Ni30-phosphorus and nitrogen doped carbon (PNC) catalyst, after optimization, achieved a half-wave potential of 0.85 V for ORR and an OER overpotential of 310 mV at 10 mA·cm-2, outperforming many state-of-the-art non-precious metal catalysts. When applied in a Zn-air battery, it achieved a peak power density of
Keywords
INTRODUCTION
The pressing issues of global air pollution and the escalating energy crisis have spurred the rapid advancement and implementation of clean energy technologies, especially those centered around energy conversion and storage[1-4]. Among them, rechargeable Zn-air batteries have garnered significant attention due to their low cost, high theoretical energy density, and environmental sustainability[5-7]. However, the slow kinetics of the oxygen reduction reaction (ORR) during the discharge and oxygen evolution reaction (OER) during charge present significant challenges to the performance of Zn-air batteries. These kinetic limitations are typically overcome by using precious metal-based catalysts such as platinum (Pt) for ORR and iridium oxide or ruthenium oxide (IrO2 or RuO2) for OER[8-11]. The high cost, scarcity, and susceptibility restrict their scalability and practical application[12,13].
In response to the challenges associated with precious metals, non-precious metal catalysts, particularly single-atom catalysts (SACs) based on transition metals, have emerged as promising alternatives due to their cost-effectiveness and high catalytic efficiency[14-16]. Among them, atomically dispersed iron and nitrogen co-doped carbon (Fe-N-C) materials have attracted considerable interest for their excellent ORR performance[17,18]. However, these catalysts face inherent limitations in OER performance, primarily due to the competition between multiple reaction intermediates at the single active site, which hinders their bifunctional catalytic activity[19,20]. To address these limitations, several strategies have been explored, such as the doping of heteroatoms to modify the electronic properties of active sites. For instance, Zhao et al. demonstrated boron (B)-doped single-atom Fe-N-C materials, which enhanced both ORR and OER activities by increasing the exposure of Fe active sites and modulating their electronic properties[7]. Our group reported phosphorus (P)-doped Fe-N-C (P/Fe-N-C) catalysts, synthesized by carbonizing ferric phytate-modified Fe-doped zeolitic imidazolate frameworks (Fe-doped ZIF-8). The P/Fe-N-C exhibited superior bifunctional catalytic activity due to the altered adsorption behavior of reaction intermediates, resulting in enhanced oxygen catalytic performance[21]. Additionally, the Fe-Co bimetallic SACs demonstrated enhanced bifunctional catalytic activity in alkaline medium compared to Fe SACs[22]. As an extension of bimetallic SACs, SACs containing trimetallic active sites, such as Fe, Co, and Ni, are expected to enhance bifunctional activity where Ni could synergistically facilitate both OER and ORR activity by modulating the electronic structure[23]. However, the development of trimetallic SACs remains largely unexplored. This is primarily due to the challenges associated with metal-to-metal interactions and the potential for metal agglomeration or clustering when multiple metals are incorporated in varying proportions.
Herein, we introduce a novel approach for the design and synthesis of Fe-Co-Ni trimetallic SACs for efficient bifunctional catalysis in a rechargeable Zn-air battery. Leveraging the high porosity and large surface area of ZIF-8 as a support material, we employed phytic acid (PA), a strong chelating agent, to form stable metal-chelate complexes with Fe, Co, and Ni ions. This method not only prevented metal agglomeration during the high-temperature carbonization process but also enhanced the metal loading capacity, ensuring the formation of atomic sites for catalysis with controlled metal ratios, which are critical for achieving optimal catalytic performance. The optimized Fe30Co30Ni30-phosphorus and nitrogen doped carbon (PNC) catalyst demonstrated exceptional performance, with an ORR half-wave potential (E1/2) of 0.85 V and an OER overpotential of 310 mV at a current density of 10 mA·cm-2. When integrated into a rechargeable Zn-air battery, this catalyst delivered a peak power density of 221 mW·cm-2, a high specific capacity of 791.3 mAh·gZn-1, and remarkable durability with over 330 h of stable operation. These results highlight the potential of Fe-Co-Ni trimetallic SACs as a promising strategy for enhancing the performance of rechargeable Zn-air batteries, offering a path toward the scalable and sustainable implementation of clean energy storage systems.
EXPERIMENTAL
Preparation of Fe, Co, Ni trimetallic SACs
In a typical procedure, 30 mg of iron(III) nitrate nonahydrate [Fe(NO3)3∙9H2O], 30 mg of cobalt(II) acetylacetonate [Co(acac)2] and 30 mg of nickel(II)-acetylacetonate [Ni(acac)2] were dissolved in 200 mL of methanol. After heating the solution to 60 °C, 90 μL of PA was added and the mixture was stirred vigorously for 2 h. Once cooled to room temperature, 100 mL of methanol containing 2.5 g of zinc nitrate hexahydrate [Zn(NO3)2·6H2O] was added, followed by 100 mL of methanol containing 3.0 g of 2-methylimidazole and
Characterization
X-ray diffraction (XRD) data were obtained using a Rigaku SmartLab SE diffractometer, with a Cu Kα source (λ = 1.54 Å). The micromorphology of the samples was analyzed with a Hitachi H-7800 transmission electron microscope (TEM), while high-resolution TEM (HRTEM) images were captured with a JEOL-2100F microscope. Aberration-corrected high-angle annular dark-field scanning transmission electron microscopy (AC-HAADF-STEM) images and element mapping were performed using a JEOL JEM-ARM 200, equipped with a spherical aberration corrector. X-ray photoelectron spectroscopy (XPS) analysis was conducted on a Thermo Scientific K-Alpha spectrometer utilizing an Al Kα excitation source. Metal loadings were determined using inductively coupled plasma atomic emission spectroscopy (ICP-AES) on a VISTA MPX system (Varian Inc.). First, a 10 mg sample was placed in a quartz boat and heated in air at
Electrochemical measurements
Electrochemical tests were carried out at ambient temperature using a three-electrode setup on the CHI 760E electrochemical workstation. A catalytic ink was prepared by sonicating a mixture of 2.5 mg catalyst, 490 μL ethanol, and 10 μL Nafion solution (5 wt%). A specific volume of the resulting suspension was drop-cast onto a freshly polished glassy carbon rotating disk electrode (RDE) or rotating ring-disk electrode (RRDE), forming a uniform film with a catalyst loading of 0.76 mg·cm-2. For comparison, commercial Pt/C (20 wt%) and RuO2 were tested at a loading of 0.2 mg·cm-2. ORR polarization curves were recorded in an O2-saturated 0.1 M KOH solution with a rotation speed of 1,600 rpm and a scan rate of 5 mV·s-1. The ORR durability was evaluated by continuous potential cycling from 0.6 to 1.0 V vs. reversible hydrogen electrode (RHE) in O2-saturated 0.1 M KOH at a scan rate of 100 mV·s-1. OER polarization curves were measured in an O2-saturated 1 M KOH solution with 95% iR compensation, using a carbon paper-loaded catalyst as the working electrode. In each experiment, a graphite rod acted as the counter electrode, while a Hg/HgO electrode was employed as the reference. The potentials were referenced to the RHE using[24]
The yield of H2O2 (H2O2 %) and the number of electron transfers (n) were determined through a RRDE technique and calculated based on[25,26]
where ir denotes the current from the ring, id refers to the disk current, and N represents the collection efficiency of the ring, which is 0.37.
The electrochemical active surface area (ECSA) was determined from the double-layer capacitance (Cdl) measured in the non-faradaic potential regions. A linear correlation was found by plotting the current density difference (Janodic - Jcathodic) at 1.35 V from the anodic and cathodic sweeps against the scan rate. Using this data, the Cdl value was calculated using
The turnover frequency (TOF) value towards OER was calculated by[21]
where j (A·cm-2) represents the current density, A (cm-2) indicates the geometric surface area of the electrode, F (s·A·mol-1) denotes the Faraday constant, and m (mol) stands for the number of moles of metal on the electrode.
RESULTS AND DISCUSSION
Synthesis and structural characterization of Fe-Co-Ni trimetallic SACs
As illustrated in Figure 1A, the trimetallic SACs were synthesized via a one-step pyrolysis method using PA as a chelating agent to coordinate with Fe, Co, and Ni precursors, followed by high-temperature carbonization on a ZIF-8 support. The coordination of metal ions with the PNC support facilitated the formation of isolated metal atoms at the atomic level, while preventing metal agglomeration during the synthesis process. The metal ratios in catalysts could be well controlled during the pre-chelated step via PA. The XRD pattern of all the samples displayed two broad peaks at approximately 24.5° and 43.2°, which correspond to the (002) and (101) planes of graphitic carbon[27] [Supplementary Figure 1]. For Fe30Co30Ni30-PNC, the XRD patterns showed no obvious crystalline peaks corresponding to metal nanoparticles (e.g., metal alloys, oxides, and phosphides), implying that most metals were atomically dispersed. The formation of alloy nanoparticles occurred in Fe50Co20Ni20-PNC, Fe20Co50Ni20-PNC and Fe20Co20Ni50-PNC, evidenced by a weak peak appearing at ~40.4°. The TEM image of Fe30Co30Ni30-PNC at low magnification showed a well-maintained morphology inherited from ZIF-8 nanoparticles [Figure 1B], with no observable metal nanoparticles or clusters. In contrast, nanoparticles were found in trimetallic materials with other metal ratios [Figure 1C-E][28].
Figure 1. (A) Schematic illustration of the synthesis of Fe-Co-Ni trimetallic SACs on PNC support; TEM images of (B) Fe30Co30Ni30-PNC, (C) Fe50Co20Ni20-PNC, (D) Fe20Co50Ni20-PNC, and (E) Fe20Co20Ni50-PNC. SACs: Single-atom catalysts; PNC: phosphorus and nitrogen doped carbon; TEM: transmission electron microscope.
Furthermore, as depicted in Figure 2A and Supplementary Figure 2, the HRTEM image showed no observable metal nanoparticles or clusters. The STEM image [Figure 2B], corresponding elemental mapping images [Figure 2C-F and Supplementary Figure 3] and electron energy loss spectroscopy (EELS) analysis
Figure 2. (A) HRTEM image of Fe30Co30Ni30-PNC; (B) HAADF-STEM and corresponding elemental mapping images show the distribution of (C) P, (D) Fe, (E) Co, and (F) Ni, elements in Fe30Co30Ni30-PNC; (G) EELS and (H) aberration-corrected HAADF-STEM image of Fe30Co30Ni30-PNC. HRTEM: High-resolution transmission electron microscope; PNC: phosphorus and nitrogen doped carbon; HAADF-STEM: high-angle annular dark-field scanning transmission electron microscopy; EELS: electron energy loss spectroscopy.
The surface element information and electronic structure of the samples were determined by XPS. High-resolution N 1s XPS spectra of the samples were deconvolved into five peaks: pyridinic N (398.5 eV), metal N (399.4 eV), pyrrolic N (400.1 eV), graphitic N (401.2 eV) and oxidized N (403.4 eV) [Supplementary Figure 4]. Notably, Fe30Co30Ni30-PNC exhibited the highest metal-N content of 13.7% compared to the other trimetallic materials [Supplementary Table 2], indicating a higher atomically dispersed metal species. P signal was observed in the P 2p spectra, confirming its incorporation into the carbon structure. No peaks related to metal-P (129.0 eV) were found in the P 2p XPS spectra [Figure 3A and Supplementary Figure 5A-C], suggesting that no metal phosphide species or direct metal-P coordination were formed. High-resolution XPS spectra for Fe 2p and Co 2p showed binding energies consistent with their oxidation states (Fe2+/Fe3+, Co2+/Co3+) [Figure 3B and C], indicating the presence of metal centers in multiple oxidation states or unsaturated valence metal centers[31,32]. Similarly, the Ni site in Fe30Co30Ni30-PNC exhibits an unsaturated valence state between Ni0 (853.5 eV) and Ni2+ (855.8 eV)[33], as shown in Figure 3D. Due to the low surface atomic content in the XPS tests, the Fe 2p, Co 2p, and Ni 2p spectra of some samples did not exhibit distinct peaks [Supplementary Figure 5D-L].
Figure 3. High-resolution XPS spectra of (A) P 2p, (B) Fe 2p, (C) Co 2p, (D) Ni 2p of Fe30Co30Ni30-PNC sample; (E) N2 adsorption/desorption isotherms and (F) the corresponding pore size distributions of the Fe30Co30Ni30-PNC and Fe30Co30Ni30-NC. XPS: X-ray photoelectron spectroscopy; PNC: phosphorus and nitrogen doped carbon.
The textural properties of Fe30Co30Ni30-PNC and Fe30Co30Ni30-NC were investigated using N2 adsorption-desorption analysis. As shown in Figure 3E, the hysteresis loops confirm the presence of micropores, mesopores, and macropores in both samples. Fe30Co30Ni30-PNC exhibited a slightly higher Brunauer-Emmett-Teller (BET) surface area (1,153 m2·g-1) compared to Fe30Co30Ni30-NC (1,094 m2·g-1) [Supplementary Table 3]. Notably, Fe30Co30Ni30-PNC had a lower micropore surface area (459 m2·g-1) but a higher external surface area (695 m2·g-1) than Fe30Co30Ni30-NC. Pore size distribution analysis [Figure 3F] further confirmed this distinction, suggesting that the carbonization of PA contributed to increased mesopore formation. This enhanced porosity is expected to improve the accessibility of active sites, which is crucial for boosting electrocatalytic activity.
Electrochemical performance for OER and ORR
The electrocatalytic OER performance of Fe-Co-Ni trimetallic SACs (i.e., Fe30Co30Ni30-PNC) was first evaluated using a standard three-electrode system in an O2-saturated 1.0 M KOH solution. Fe SACs (Fe50-PNC), Co SACs (Co50-PNC), Ni SACs (Ni50-PNC), non-P doped Fe30Co30Ni30-NC, and commercial RuO2 catalysts were employed for comparison. As shown in Supplementary Figure 6, all single atomic site catalysts M SACs (M = Fe, Co or Ni) (i.e., Fe50-PNC, Co50-PNC and Ni50-PNC) exhibited poor OER activity with high overpotentials (> 390 mV) at a current density of 10 mA·cm-2, where Ni50-PNC was most active due to its higher intrinsic OER activity compared to Fe and Co. Integration of Fe, Co, Ni enhanced the OER activity of M SACs, as shown in Figure 4A. The Fe30Co30Ni30-PNC exhibited an overpotential of 310 mV at a current density of 10 mA·cm-2, outperforming the performance of RuO2 (377 mV). Among all the trimetallic SACs, the Fe30Co30Ni30-PNC (310 mV) exhibited the lowest overpotential compared to that of Fe20Co20Ni50-PNC (341 mV), Fe20Co50Ni20-PNC (395 mV) and Fe50Co20Ni20-PNC (389 mV). These results suggest that the synergistic interaction between Fe, Co, and Ni was critical for facilitating the OER, where Ni might contribute significantly to enhanced OER activity. Compared to Fe30Co30Ni30-PNC, the lower activity of
Figure 4. (A) OER LSV curves; (B) TOF values of Fe-Co-Ni trimetallic materials with different ratios of Fe/Co/Ni; (C) OER LSV curves of the Fe30Co30Ni30-PNC and RuO2 electrocatalysts before and after 25,000 s; (D) ORR polarization plots for Fe-Co-Ni trimetallic materials; (E) ORR LSV curves of the Fe30Co30Ni30-PNC and Pt/C electrocatalysts before and after 16,000 CV cycles; (F) The combined ORR and OER polarization plots of different catalysts and the corresponding potential gap (ΔE = Ej=10 - E1/2); (G) ΔE comparison of the
Furthermore, the Cdl value indicated a large ECSA for Fe30Co30Ni30-PNC, which likely contributed to its improved catalytic activity [Supplementary Figures 8 and 9]. To evaluate the intrinsic activity of
The ORR evaluation was carried out by an RDE technique in 0.1 M KOH and compared to that of a commercial Pt/C catalyst. The Fe30Co30Ni30-PNC exhibited the highest onset potential (Eonset) of 0.96 V, closely approaching that of Pt/C (0.97 V, Supplementary Figure 10). As revealed in Figure 4D, the
Opposite to the OER, increasing Ni loading resulted in decline of ORR activity, indicating the challenge for enhancing both OER and ORR activity. Furthermore, Fe30Co30Ni30-PNC exhibited the lowest Tafel slope of 56 mV·dec-1, confirming its rapid ORR kinetics [Supplementary Figure 11]. To investigate the ORR mechanism of the catalysts, the RRDE technique was used to monitor the formation of H2O2 during the tests. Within the potential range of 0.2 to 0.7 V (vs. RHE), Fe30Co30Ni30-PNC demonstrated a low H2O2 yield of 7.2%-10.8% and a high electron transfer number of ~4.0 [Supplementary Figure 12], indicating a dominating 4e- ORR pathway, which was in strong agreement with the results obtained from a Koutecky-Levich (K-L) method [Supplementary Figure 13]. Besides, the ORR durability of Fe30Co30Ni30-PNC was evaluated at a rotation speed of 1,600 rpm over continuous 16,000 potential cycles. As revealed in Figure 4E, the E1/2 of Fe30Co30Ni30-PNC decreased by only 10 mV, outperforming commercial Pt/C (40 mV).
Determining the precise active sites for ORR and OER in Fe30Co30Ni30-PNC is challenging due to the complexity of its structure, including the presence of Fe, Co, Ni, and N- and P-doping. However, based on the experimental data and the intrinsic activity of each metal, we propose a possible explanation for the observed catalytic trends. The ORR activity follows the order Fe30Co30Ni30-PNC > Fe50Co20Ni20-PNC >
The bifunctional oxygen electrocatalytic performance was determined by calculating the potential gap (ΔE) between the OER potential at 10 mA·cm-2 (Ej=10) and the ORR E1/2. Notably, the Fe30Co30Ni30-PNC showed a small ΔE of 0.69 V, much lower than other tested catalysts, including the Pt/C-RuO2 benchmark couple [Figure 4F]. Even in comparison with the recently reported high-performance bifunctional catalysts
Bifunctional catalytic performance in Zn-air battery
To assess the practical applicability of the Fe30Co30Ni30-PNC catalyst, it was incorporated into a Zn-air battery as the cathode catalyst. The performance of a rechargeable Zn-air battery was evaluated in a 6.0 M KOH electrolyte containing 0.2 M Zn(OAc)2 [Figure 5A]. As a comparison, a Pt/C-RuO2 couple was also tested. As shown in Figure 5B, the Fe30Co30Ni30-PNC catalyst achieved a peak power density of
Figure 5. Performance of the rechargeable Zn-air battery: (A) Schematic diagram showing the assembly process of the rechargeable Zn-air battery; (B) Discharge polarization curves and corresponding power density plots for the Zn-air battery with Fe30Co30Ni30-PNC and Pt/C–RuO2 couples as the air electrodes; (C) Specific capacities normalized to Zn mass at a current density of 10 mA·cm-2; (D) Discharge/charge cycling curves for the Zn-air battery using Fe30Co30Ni30-PNC and Pt/C–RuO2 couples as air electrodes at a current density of 10 mA·cm-2. PNC: Phosphorus and nitrogen doped carbon.
To investigate the cycling performance of Zn-air batteries, a constant current charge-discharge test (discharge 10 min, charge 10 min) was carried out at the current density of 10 mA·cm-2 [Figure 5D]. This degradation is primarily associated with the stability of the catalyst under OER conditions, as OER remains the key limiting factor in long-term rechargeable Zn-air battery operation. To further understand the structural changes leading to this performance drop, TEM and XPS analysis were performed on the used Fe30Co30Ni30-PNC catalyst after cycling. TEM imaging and elemental mapping indicated the formation of Co-rich aggregates while Fe and Ni appeared to dissolve into the electrolyte [Supplementary Figure 14]. However, XPS analysis suggested significant Co dissolution, with Fe and Ni instead forming localized aggregations [Supplementary Figure 15]. This discrepancy is likely due to the localized nature and depth-dependent resolution of TEM and XPS, which probe different regions of the catalyst. Despite these changes in metal distribution, N 1s and P 2p XPS spectra revealed that carbon corrosion is the primary cause of catalyst degradation. Under OER conditions, carbon oxidation disrupts the structural integrity of the metal-coordinated sites, leading to metal leaching, aggregation, and surface restructuring, which ultimately result in decreased catalytic activity. Future work should focus on enhancing carbon stability through improved graphitization, surface functionalization, or hybridizing with oxidation-resistant supports to further extend the durability of catalysts in alkaline OER applications. In contrast, the battery based on commercial Pt/C-RuO2 catalysts demonstrated a significant loss of activity after only 54 h of cycling. This excellent performance was a direct result of the catalyst’s superior bifunctional activity for both ORR and OER, enabling efficient charge and discharge processes in the Zn-air battery.
CONCLUSIONS
In summary, we have demonstrated Fe-Co-Ni trimetallic SACs for efficient bifunctional catalysis in rechargeable Zn-air batteries. The optimized Fe30Co30Ni30-PNC catalyst exhibited exceptional catalytic performance, with both high ORR and OER activity, and outperformed many state-of-the-art non-precious metal catalysts. When integrated into a Zn-air battery, the catalyst achieved a peak power density of
DECLARATIONS
Authors’ contributions
Made substantial contributions to conception and design of the study and performed data analysis and interpretation: Zhou, Y.; Chen, G.
Material synthesis, characterization and electrochemical analyses: Zheng, Z.; Wang, L.; Maouche, C.
Provided administrative and technical support: Tao, X.; Ju, H.
All authors discussed the results and commented on the manuscript.
Availability of data and materials
The raw data supporting the findings of this study are available within this Article and its Supplementary Materials. Further data is available from the corresponding authors upon reasonable request.
Financial support and sponsorship
This work was supported by the National Natural Science Foundation of China (22102064), the European Union under the REFRESH (No. CZ.10.03.01/00/22_003/0000048) via the Operational Programme Just Transition from the Ministry of the Environment of the Czech Republic, the national funds through FCT of Portugal-Fundação para a Ciência e a Tecnologia, under a scientific contract (2023.06224.CEECIND), and the projects UIDB/00285/2020 and LA/0112/2020.
Conflicts of interest
All authors declared that there are no conflicts of interest.
Ethical approval and consent to participate
Not applicable.
Consent for publication
Not applicable.
Copyright
© The Author(s) 2025.
Supplementary Materials
REFERENCES
1. Kibsgaard, J.; Chorkendorff, I. Considerations for the scaling-up of water splitting catalysts. Nat. Energy. 2019, 4, 430-3.
2. Xu, W.; Lu, Z.; Zhang, T.; et al. An advanced zinc air battery with nanostructured superwetting electrodes. Energy. Storage. Mater. 2019, 17, 358-65.
3. Sekhon, S. S.; Lee, J.; Park, J. Biomass-derived bifunctional electrocatalysts for oxygen reduction and evolution reaction: a review. J. Energy. Chem. 2022, 65, 149-72.
4. Chen, G.; Wang, T.; Zhang, J.; et al. Accelerated hydrogen evolution kinetics on NiFe-layered double hydroxide electrocatalysts by tailoring water dissociation active sites. Adv. Mater. 2018, 30, 1706279.
5. Fu, J.; Cano, Z. P.; Park, M. G.; Yu, A.; Fowler, M.; Chen, Z. Electrically rechargeable zinc-air batteries: progress, challenges, and perspectives. Adv. Mater. 2017, 29, 1604685.
6. Yan, Y.; Yu, R.; Liu, M.; et al. General synthesis of neighboring dual-atomic sites with a specific pre-designed distance via an interfacial-fixing strategy. Nat. Commun. 2025, 16, 334.
7. Zhao, S.; Liu, M.; Qu, Z.; et al. Cascade synthesis of Fe-N2-Fe dual-atom catalysts for superior oxygen catalysis. Angew. Chem. Int. Ed. Engl. 2024, 63, e202408914.
8. Chen, C.; Chai, J.; Sun, M.; et al. An asymmetrically coordinated ZnCoFe hetero-trimetallic atom catalyst enhances the electrocatalytic oxygen reaction. Energy. Environ. Sci. 2024, 17, 2298-308.
9. Zhang, W.; Xu, C.; Zheng, H.; Li, R.; Zhou, K. Oxygen-rich cobalt–nitrogen–carbon porous nanosheets for bifunctional oxygen electrocatalysis. Adv. Funct. Mater. 2022, 32, 2200763.
10. Chen, G.; Wang, T.; Liu, P.; et al. Promoted oxygen reduction kinetics on nitrogen-doped hierarchically porous carbon by engineering proton-feeding centers. Energy. Environ. Sci. 2020, 13, 2849-55.
11. Zhang, D.; Wang, Z.; Liu, F.; et al. Unraveling the pH-dependent oxygen reduction performance on single-atom catalysts: from single- to dual-sabatier optima. J. Am. Chem. Soc. 2024, 146, 3210-9.
12. Chen, G.; Zhong, H.; Feng, X. Active site engineering of single-atom carbonaceous electrocatalysts for the oxygen reduction reaction. Chem. Sci. 2021, 12, 15802-20.
13. Xie, H.; Xie, X.; Hu, G.; et al. Ta–TiOx nanoparticles as radical scavengers to improve the durability of Fe–N–C oxygen reduction catalysts. Nat. Energy. 2022, 7, 281-9.
14. Al-Hilfi, S. H.; Jiang, X.; Heuer, J.; et al. Single-atom catalysts through pressure-controlled metal diffusion. J. Am. Chem. Soc. 2024, 146, 19886-95.
15. Wang, A.; Li, J.; Zhang, T. Heterogeneous single-atom catalysis. Nat. Rev. Chem. 2018, 2, 65-81.
16. Li, B.; Ju, C. W.; Wang, W.; et al. Heck migratory insertion catalyzed by a single Pt atom site. J. Am. Chem. Soc. 2023, 145, 24126-35.
17. Chen, G.; An, Y.; Liu, S.; et al. Highly accessible and dense surface single metal FeN4 active sites for promoting the oxygen reduction reaction. Energy. Environ. Sci. 2022, 15, 2619-28.
18. Wang, Q.; Yang, Y.; Sun, F.; et al. Molten NaCl-assisted synthesis of porous Fe-N-C electrocatalysts with a high density of catalytically accessible FeN4 active sites and outstanding oxygen reduction reaction performance. Adv. Energy. Mater. 2021, 11, 2100219.
19. Zhang, J.; Zhao, Y.; Zhao, W.; et al. Improving electrocatalytic oxygen evolution through local field distortion in Mg/Fe dual-site catalysts. Angew. Chem. Int. Ed. 2023, 135, e202314303.
20. Li, L.; Yuan, K.; Chen, Y. Breaking the scaling relationship limit: from single-atom to dual-atom catalysts. Acc. Mater. Res. 2022, 3, 584-96.
21. Zhou, Y.; Lu, R.; Tao, X.; et al. Boosting oxygen electrocatalytic activity of Fe-N-C catalysts by phosphorus incorporation. J. Am. Chem. Soc. 2023, 145, 3647-55.
22. He, Y.; Yang, X.; Li, Y.; et al. Atomically dispersed Fe–Co dual metal sites as bifunctional oxygen electrocatalysts for rechargeable and flexible Zn–air batteries. ACS. Catal. 2022, 12, 1216-27.
23. Vij, V.; Sultan, S.; Harzandi, A. M.; et al. Nickel-based electrocatalysts for energy-related applications: oxygen reduction, oxygen evolution, and hydrogen evolution reactions. ACS. Catal. 2017, 7, 7196-225.
24. Zhu, X.; Liu, G.; Tao, X.; et al. Role of the metal precursor in preparing dual-atom catalysts for the oxygen reduction reaction. ACS. Omega. 2023, 8, 41708-17.
25. Zhu, Q.; Xiang, T.; Chen, C.; et al. Enhancing activity and stability of FeNC catalysts through co incorporation for oxygen reduction reaction. J. Colloid. Interface. Sci. 2024, 663, 53-60.
26. Chen, K.; Liu, K.; An, P.; et al. Iron phthalocyanine with coordination induced electronic localization to boost oxygen reduction reaction. Nat. Commun. 2020, 11, 4173.
27. Maouche, C.; Yang, J.; Al-Hilfi, S. H.; Tao, X.; Zhou, Y. Sulfur-doped Fe–N–C nanomaterials as catalysts for the oxygen reduction reaction in acidic medium. ACS. Appl. Nano. Mater. 2022, 5, 4397-405.
28. Zhou, Y.; Chen, G.; Wang, Q.; et al. Fe–N–C electrocatalysts with densely accessible Fe–N4 sites for efficient oxygen reduction reaction. Adv. Funct. Mater. 2021, 31, 2102420.
29. Li, R.; Fan, W.; Rao, P.; et al. Multimetallic single-atom catalysts for bifunctional oxygen electrocatalysis. ACS. Nano. 2023, 17, 18128-38.
30. Zhong, J.; Liang, Z.; Liu, N.; et al. Engineering symmetry-breaking centers and d-orbital modulation in triatomic catalysts for zinc-air batteries. ACS. Nano. 2024, 18, 5258-69.
31. Xu, Z.; Zhu, J.; Shao, J.; et al. Anti-dissolving Fe2N6 site-based carbon fiber membranes for binder-free Zn–air batteries with a 200-day lifespan. Energy. Environ. Sci. 2024, 17, 8722-33.
32. Hu, B.; Huang, A.; Zhang, X.; et al. Atomic Co/Ni dual sites with N/P-coordination as bifunctional oxygen electrocatalyst for rechargeable zinc-air batteries. Nano. Res. 2021, 14, 3482-8.
Cite This Article
How to Cite
Download Citation
Export Citation File:
Type of Import
Tips on Downloading Citation
Citation Manager File Format
Type of Import
Direct Import: When the Direct Import option is selected (the default state), a dialogue box will give you the option to Save or Open the downloaded citation data. Choosing Open will either launch your citation manager or give you a choice of applications with which to use the metadata. The Save option saves the file locally for later use.
Indirect Import: When the Indirect Import option is selected, the metadata is displayed and may be copied and pasted as needed.
About This Article
Special Issue
Copyright
Data & Comments
Data
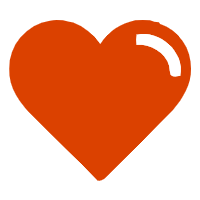
Comments
Comments must be written in English. Spam, offensive content, impersonation, and private information will not be permitted. If any comment is reported and identified as inappropriate content by OAE staff, the comment will be removed without notice. If you have any queries or need any help, please contact us at [email protected].