Alloyed single-atom catalysts for electro- and photo- catalytic water splitting
Abstract
Water splitting by using renewable energy to produce hydrogen and oxygen can be regarded as one of the most promising approaches for sustainable energy conversion. Developing cost-effective and high-performance water splitting catalysts plays an increasingly important role in enhancing overall efficiency. Alloyed single-atom catalysts (alloyed SACs, also known as single-atom alloy), with one of the metal atoms atomically dispersed in a host metal, combine the advantages of both SACs and traditional metal alloys. They show the maximum utilization of active metal atoms and uniquely geometric and electronic structures, offering great potential in reducing the cost of catalyst and boosting the performance in catalytic water splitting. This review aims to provide a comprehensive summary of the development of alloyed SACs for oxygen and hydrogen evolution reactions by water splitting. We start with a brief introduction of the mechanism for water splitting under electrocatalytic and photocatalytic conditions, followed by emphasizing the merits of the formation of alloyed SACs for water splitting. Then, the case studies of electro- and photo- catalytic hydrogen and oxygen evolution via water splitting are illustrated and discussed. Finally, challenges and prospects are provided, with further continued efforts expected for achieving future exciting progress in tailoring the active sites for designing high-performance catalysts.
Keywords
INTRODUCTION
Water splitting using renewable energy to produce hydrogen and oxygen has been recognized as one of the most promising ways to store energy for future chemical transformation[1,2]. To realize the reaction with high energy efficiency, design and synthesis of high-performance water splitting catalysts are important. The strategies for optimizing catalysts both economically and efficiently include increasing the intrinsic reactivity of the metal atoms, optimizing the exposure of the active sites, constructing synergy effects with multiple metal elements and so forth. For instance, single-atom catalysts (SACs), with their maximum utilization of the metal active sites at the atomic level, have been extensively studied for water splitting, while the poor stability significantly limits their practical utilization[3]. Alloying strategies have long been recognized as a promising approach to enhance catalytic performance by rationally designing the electronic structures, arranging atomic configurations and optimizing the synergy effect of different metal elements[4,5]. For example, compared with their monometallic counterparts, Pt-Zn[6], Pt-Ni[7], and Pd-Ir[8] alloy nanocatalysts have been proved to demonstrate superior catalytic performance for water splitting to produce hydrogen and oxygen. Nevertheless, due to the random distribution of the metal atoms, the utilization of the active sites is insufficient.
The formation of alloyed SACs, in which the catalytically active metal atoms are atomically isolated by another metal element, can make use of the active metal element at the atomic level, and the catalytic performance will be further optimized due to the synergy effect between different metal elements. Thus, since the first demonstration of the isolated Pd atoms on Cu(111) for semi-hydrogenation of alkynes[9], such catalysts have been extensively investigated for various catalytic applications, including oxidation, dehydrogenation, photocatalysis, electrocatalysis and so forth[10-13]. Our previous work also demonstrated that group IB metals (Au, Ag and Cu) alloyed Pd SACs displayed excellent catalytic performance for acetylene semi-hydrogenation under simulated industrial conditions[14-17]. Recently, increasing attention has been paid to the catalytic applications of alloyed SACs for sustainable energy conversion[18-22]. For instance, the core/shell nanocrystals of densely packed Cu/CuAu material with intermetallic alloyed single atom layer structure demonstrated excellent selectivity toward NH3 for the electrocatalytic nitrate reduction reaction[23]. The enhanced catalytic performance has been ascribed to the formation of alloyed Au single atoms into the Cu matrix, which can introduce ligand effects and thus optimize the *NO3 and *N chemisorption. Similarly, single-atom Bi-decorated Cu also demonstrated remarkably enhanced C2+ product selectivity for CO2 electrocatalytic reduction[24], with an optimal Faradaic efficiency (FE) of 73.4%, which was superior to that of the pure Cu or Bi nanoparticle-decorated Cu nanocomposites.
The synthesis, characterization, and varied catalytic applications of alloyed SACs have been summarized and discussed by several reviews[10-13,18-22], while there is still no summarized discussion on their catalytic application for water splitting. Herein, we first start with a brief introduction of the mechanism for water splitting via electrocatalytic and photocatalytic processes, followed by discussing the merits of alloyed SACs for water splitting; then, special attention is paid to the cases of electrocatalytic and photocatalytic hydrogen evolution and oxygen evolution by water splitting. Finally, challenges and outlooks are provided and discussed for guiding the rational design of highly efficient catalysts for water splitting.
FUNDAMENTALS FOR WATER SPLITTING
Water splitting for oxygen evolution reaction (OER) and hydrogen evolution reaction (HER) can theoretically be realized with a minimum voltage of 1.23 V at room temperature. However, normally, additional energy is needed to drive these reactions. For electrocatalysis, it is manifested as the potential bias exceeding the minimum of 1.23 V. While for photocatalysis, photoexcitation energies above 2 eV are required. We will briefly discuss these in this section.
Electrocatalytic water splitting
For acidic electrolytes, OER occurs at the anode, while the HER takes place at the cathode. The corresponding reactions are as follows:
Under alkaline conditions, HER and OER can be expressed as:
The extra voltage needed for reaching specific current density is named overpotential (η). Catalysts are used to minimize η as much as possible. In alkaline solutions, transition metals and their alloys are usually used for HER[25]; transition metal oxides can function effectively for OER[26]. Under acidic conditions, precious metals and precious metal oxides are normally effective catalysts for water splitting, such as RuO2 and IrO2 at the anode and Pt at the cathode[27].
The detailed mechanism for HER and OER varies under different conditions. Herein, we will simply discuss the generally accepted mechanisms for HER. Two steps are involved for HER in alkaline and acidic media: electrochemical reaction and conversion step [Figure 1][28]. The electrochemical reaction step, also named Volmer reaction, undergoes the following reactions:
Figure 1. Mechanism for electrocatalytic HER in (A) acidic and (B) alkaline medium. HER: Hydrogen evolution reaction.
Here, *H represents H atoms adsorbed over the active sites and * denotes active centers over the catalyst. In terms of the conversion step, *H undergoes either recombination (Tafel reaction) or electrochemical desorption (Heyrovsky reaction) to generate H2, which depends on the nature of the electrode material. For the Heyrovsky reaction, one of the solvated H2O or H+ from the water layer interacts with *H to generate H2:
The Tafel step is completed via the following reaction to produce H2, followed by H2 desorption from the electrode surface:
Over decades of study, four main mechanisms are proposed for OER: lattice oxygen-mediated mechanism (LOM), adsorbate evolution mechanism (AEM), bi-nuclear mechanism (BNM), and oxide path mechanism (OPM)[1]. In this work, we will simply express the mechanism for OER in an acidic medium in
when OER occurs in a neutral or alkaline solution, we employ
with the possible elementary steps shown in Figure 2. According to the above explanation, OER involves a complex four-electron transfer process, which leads to significantly slower kinetics than HER. Thus, OER is both the thermodynamic and kinetic bottleneck for water splitting.
Photocatalytic water splitting
For photocatalysis, a semiconductor with a suitable bandgap is needed to absorb photon energy to drive the generation of electron-hole pairs; holes and electrons are generated in the valence and conduction bands, respectively. The hole and electron generated by photoexcitation drive water splitting to occur similarly to electrocatalysis [Figure 3][29]: with electrons reducing protons to produce H2 and holes oxidizing water to produce O2. Thus, a photocatalytic process can be divided into three steps[29]: (i) photons with energy higher than the bandgap values are absorbed to generate electron-hole pairs in a semiconductor; (ii) photogenerated charge migration and separation; and (iii) redox reactions at the surface.
Figure 3. Schematic illustration of the photoexcitation in a semiconductor followed by deexcitation events. This figure is quoted with permission from ref[29]. Copyright 2012, American Chemical Society.
However, though the generated holes and electrons are thermodynamically suitable for the reaction, electron-hole pairs will easily recombine if there are not enough active sites for driving transfer of holes and electrons for the reaction to occur[30]. Co-catalysts (e.g., Pt, RuO2, and alloys) can be loaded onto the semiconductor surface to act as active sites for driving water splitting[30].
For both electrocatalytic and photocatalytic processes, catalysts play important roles. Normally, synergy effects between different metal elements are usually considered for enhancing catalytic efficiency.
ADVANTAGES OF ALLOYED SACs FOR WATER SPLITTING
Catalytic stability
Atomic-level utilization of active sites is significantly important. SACs, which make use of active metal elements with atomic precision, have gained great attention in recent years. However, the stability of SACs under electrocatalytic conditions is poor which limits their utilization for water splitting and other electrocatalytic applications[3]. Different from most SACs, single atoms alloyed with other metal elements can significantly enhance stability. This is because, in one aspect, the ensembles are the thermodynamically preferred phase for many alloyed single atoms, and sintering of the isolated atom sites into ensembles can be largely inhibited. Additionally, after forming an alloy with another metal element, the stability during photocatalysis or electrocatalysis processes can be largely enhanced due to the formation of nanoparticles/clusters. For instance, the performance and structure could be well maintained for the flow cell system at a current density of 400 mA·cm-2 for single-atom Bi-decorated Cu nanocomposites[24]. Furthermore, the oxidation of metallic catalysts can be largely suppressed under positive potential, thus demonstrating superior stability for electrocatalytic OER. For instance, the electrocatalytic stability for OER over Ru1-Pt3Cu was greater than that of RuO2[31]. This illustrated that after the formation of alloyed single atoms, the stability limitation of the catalysts is the stability of the nanoparticles/clusters rather than the isolated active sites. To address this issue, more attention should be paid to methods for stabilizing the nanoparticle/cluster such as stabilizing it in a porous material.
Synergy effect
After forming alloys, the catalytic performances of alloyed SACs are usually affected by various factors, thus influencing the types and binding energy of the reactants and the intermediates. The crucial structure factors affecting the catalytic performance for alloyed SACs include geometric and electronic structure. For instance, compared with pristine Ru, substitution of Ru with single Co atoms (denoted as RuCo1) will reduce the energy barrier for water dissociation[32]. Meanwhile, the energy barriers will be increased when increasing the substitution to two (RuCo2) or three (RuCo3) neighbor Co atoms, indicating that geometrically, single Co substitution can enhance the sluggish water dissociation of both RuCon (n ≥ 2) and pristine Ru catalysts. Moreover, the addition of more Co atoms will increase the binding energy of OH (ΔEOH), while single Co atom substitution induces a moderate ΔEOH. Thus, RuCo1 structure can not only facilitate water dissociation, but also prevent the deactivation of the active sites induced by high-affinity hydroxides.
Meanwhile, the charge transfer due to the differences in electronegativity as a short-range effect also alters the electronic structures and leads to the variation in binding strength of the intermediates. These effects are summarized as the electronic effects. Different from traditional alloy catalysts, no metallic bond is formed between the neighboring alloyed atoms, inducing a free-atom-like electronic structure[33], which is common for homogeneous catalysts with the ligands tuning the electron density of the metal center and thus the reactivity of the catalysts. For example, after loading Ru single atom onto CoFe2(O-8)(110), charge transfer occurs from Ru to the coordinated surface Olattice, which will induce an electron-deficient center to accelerate electrocatalytic OER and facilitate intermediate adsorption[34].
CASE STUDIES OF ALLOYED SACs FOR WATER SPLITTING
Electrocatalytic HER
Pt-based catalysts
Since Pt nanocatalysts are the most widely used electrocatalysts for HER, decorating Pt nanocatalysts with other low-cost transition metals offers an effective way to optimize their catalytic properties. Table 1 summarizes the representative applications of alloyed SACs for electrocatalytic water splitting. For instance, by starting with the PtNi nanowire and a partial electrochemical dealloying approach, single Ni atom-modified Pt nanowires demonstrated an optimum combination of electrochemical active surface area and specific activity for not only HER but also methanol oxidation and ethanol oxidation[35]. Density functional theory (DFT) calculations indicated that Ni single atoms, which are coordinated with two hydroxyl groups [Figure 4A], electronically enhanced HER activity over the neighboring Pt atoms. Single In atom-doped sub-nanometer Pt nanowires were also reported to be excellent electrocatalysts for HER under universal pH conditions[36]. Mechanistic studies indicated that the combination of single atom In decoration [Figure 4B and C] and ultrathin 1D morphology provided the maximum active sites and effectively activated Pt atoms for the reaction to occur. Pt nanoclusters as electrocatalysts for HER are normally not stable, while when they were loaded onto Cr-N4 with atomically dispersed Cr sites, both the activity and stability were enhanced, which outperforms the activity of commercial Pt/C catalysts[37]. Theoretical and experimental studies demonstrated that a unique Pt-Cr quasi-covalent bond was formed at the interface of Cr-N4 sites, and Pt nanoclusters which effectively suppressed thermal vibration and migration of Pt atoms contributed to the significantly enhanced stability. Furthermore, exophilic Cr-N4 sites adjacent to Pt nanoclusters demonstrated favorable adsorption for hydroxyl species, which favored water dissociation nearly barrierless and thus enhanced HER activity.
Figure 4. (A) Model for single-atom Ni-decorated Pt (111) surface, with the Ni atoms in the surface layer. This figure is quoted with permission from ref[35]. Copyright 2019, Springer Nature; (B) HAADF-STEM image and (C) aberration-corrected HAADF-STEM image for single atom In decorated Pt nanowires. Figures are quoted with permission from ref[36]. Copyright 2020, Wiley-VCH; (D) TEM image of the Pt/Ni catalyst, (E) HAADF-STEM image and (F) magnified aberration-corrected image obtained from the white square in (E); (G) free adsorption energies of H over Pt-Ni-hcp and Ni-hcp; (H) corresponding dissociation–diffusion–desorption mechanism for hydrogen evolution over Pt-Ni-hcp. Figures are quoted with permission from ref[38]. Copyright 2021, American Chemical Society. HAADF-STEM: High-angle annular dark field scanning transmission electron microscopy; TEM: transmission electron microscopy.
Summary of the representative alloyed SACs for electrocatalytic HER and OER
Entry | Catalysts | Single atom | Reaction | Electrolytes | Tafel slope (mV·dec-1) | Overpotential (@10 mA·cm-2/mV) | Ref. |
1 | SANi-PtNWs | Ni | HER | 1 M NaOH | 60.3 | / | [35] |
2 | SA In-Pt NWs | In | HER | 1 M KOH | 32.4 | 46 | [36] |
3 | Pt/Ni ASs/C | Pt | HER | 1 M KOH | 47 | 28 | [38] |
4 | NiPt | Pt | HER | 1 M KOH | 45 | 18 | [39] |
5 | (Ru@Pt)–N | Pt | HER | 1 M KOH | 30 | 15 | [40] |
6 | Pd-Pt/NCNTs | Pt | HER | / | 28.5 | / | [41] |
7 | RuAu SAA | Au | HER | 1 M KOH | 37 | 24 | [42] |
8 | Ru SA-Co-N-C | Ru | HER | 0.1 M KOH | 64 | 43 | [43] |
9 | Ir1Ni@MoO2 | Ir | HER/OER | 1 M KOH | 19/91 | 48.6/280 | [44] |
10 | Co-substituted Ru NSs | Co | HER | 1 M KOH | 29 | 13 | [32] |
11 | Co/Pdm-4 | Co | HER | 0.5 m H2SO4 | 8.2 | 24.7 | [46] |
12 | Ru1-Pt3Cu | Ru | OER | 0.1 M HClO4 | / | 90 | [31] |
13 | RuSACoFe2/G | Ru | OER | 1 M KOH | 51 | 80 | [34] |
14 | NiFeIrx/Ni | Ir | OER | 1 M KOH | 44.6 | 200 | [57] |
15 | Ir@Co nanosheets | Ir | OER | 1 M KOH | 99 | 273 | [58] |
Although with high HER activity, the high price of Pt induces high costs of the catalytic systems. Atomically dispersed Pt atoms offer an opportunity to reduce the catalyst cost and promote HER activity. Ding et al. embedded isolated Pt atoms into hexagonal close-packed and anisotropic super-structured nickel (Pt/Ni, Figure 4D-F) to enhance HER performance in 1 M KOH, with the overpotential of 28.0 mV at 10 mA·cm-2 over Pt/Ni catalyst which is superior to that of 71.0 mV over Pt/C[38]. The Tafel slope of 47.0 mV·dec-1 shows the Volmer-Heyrovsky mechanism over the Pt/Ni catalyst. DFT calculations show that the adsorption free energy over the Pt-Ni-hcp model is much higher than on Ni-hcp [Figure 4G], indicating that hydrogen adsorption is favorable over Ni sites, while H2 desorption is favored over Pt sites. Thus, the improved HER activity over Pt/Ni can be explained by a dissociation-diffusion-desorption mechanism [Figure 4H]: due to the dissociation of H2O, H was firstly adsorbed over Ni, and then diffused to Pt sites, followed by H2 release from Pt sites. The synergy effects between Ni-Pt and Ni-Ni, which are responsible for excellent HER activity, are working for Pt single-atom-promoted Ni nanosheets as well, with the activity significantly outperforming that of commercial Pt/C[39]. Likewise, Ru doped with Pt single atoms also demonstrated promoted desorption of H2. N-doped Pt-Ru alloy with isolated Pt atoms was synthesized via thermal annealing under ammonium atmosphere[40]; after optimization of the studied catalysts, (Ru-N)@Pt with Ru-N-Pt structure displayed the best HER activity. The high activity can be ascribed to the incorporated N, which can regulate Ru orbital, thus accelerating water dissociation, and Pt single atoms favored H2 desorption.
Pt/Pd alloy with isolated Pt over Pd octahedrons, which was prepared by an atomic layer deposition method, was employed to enhance the electrocatalytic HER performance[41]. X-ray absorption near-edge structure (XANES) study showed that different from other catalysts, the density of 5d states was highly unoccupied for Pt atoms. Pt/Pd alloy with octahedral structure and single Pt atoms displayed significantly enhanced ORR and HER activity compared with those of the cubic Pt/Pd alloy, Pd octahedral and pristine Pt. The Tafel slope of 28.5 mV·dec-1 indicates the Volmer-Tafel mechanism for water splitting. DFT study demonstrated that H+ was strongly adsorbed by Pt atoms over Pd(111), and the adsorption of followed two H species would be promoted due to the Pt atoms with the highly unoccupied density of 5d states. Since the increased H coverage would decrease H adsorption energy, it favors the H2 molecule formation. The calculated data also demonstrated a narrower Pt single atom projected density of d state in Pt/Pd than that of Pt(111), indicating the electronic structure changes due to the synergy effects in the alloy.
Non-Pt-based HER catalysts
Non-Pt-based catalysts gained great attention for electrocatalytic HER. Their single-atom decoration to form alloys also holds significant potential for HER. Chen et al. managed to disperse isolated Au atoms onto Ru via a laser ablation technique in a liquid phase[42]. RuAu alloy showed much lower overpotential than that of Pt (24 vs. 46 mV at 10 mA·cm-2 in 1 M KOH, respectively). The Tafel slope of 37 mV·dec-1 indicates the Volmer-Heyrosky mechanism for HER over RuAu alloy. DFT study was performed to investigate the adsorbed species evolution during the reaction, which demonstrated that RuAu alloy displayed moderate water dissociation and H2 desorption capability, while poor water dissociation capability was found over Pt and strong hydrogen binding was verified over Ru. Owing to the electronic structure changes after the formation of AuRu alloy, H adsorption and H2 molecule formation were promoted over negative Au sites, while H2O adsorption was favored over positive Ru atoms; Au and Ru played synergetic roles in different orders during the reaction which resulted from the optimized electronic effects. Meanwhile, Ru can also act as an active promoter for HER; by constructing Ru single atom-decorated Co nanoparticles into carbon sheath, a Ru SA-Co-N-C catalyst was fabricated [Figure 5A-C] which demonstrated an exceptionally high catalytic performance for overall water splitting, with an outstanding mass activity of 1,251 mA·mgRu-1 and a minimal cell voltage of 1.55 V at 10 mA·cm-2[43]. DFT calculations [Figure 5D and E] further verified that the Ru SA-Co-N-C catalyst could effectively optimize the adsorption energy of the intermediates, such as H*,
Figure 5. (A) HAADF-STEM image of Ru SA-Co-N-C, and the corresponding element contrast in randomly selected region (yellow A and blue B); (B) HRTEM image, (C) HAADF-STEM image, and the corresponding STEM-EDS of Ru SA-Co-N-C; (D) side views of atomic H adsorption on the Co-N-C and Ru SA-Co-N-C surfaces; (E) calculated free energy diagram on Co-N-C, Ru SA-Co-N-C and Pt for hydrogen evolution. Figures are quoted with permission from ref[43]. Copyright 2022, Wiley-VCH. HAADF-STEM: High-angle annular dark field scanning transmission electron microscopy; HRTEM: high-resolution transmission electron microscopy; STEM-EDS: scanning transmission electron microscopy-energy dispersive X-ray spectroscopy.
Ir-based catalysts were also recognized as efficient catalysts for water splitting. The most recent work from Wang et al. demonstrated that single-atom Ir-decorated nickel supported on segregated MoO2 showed a low overpotential of 48.6 mV at 10 mA·cm-2, with a Tafel slope of 19 mV·dec-1 for HER[44]. Experimental and theoretical studies revealed that the Ir-Ni interface significantly weakens the binding energy of H, and Ir single-atom decoration boosted the surface reconstruction of Ni species, and thus enhanced the intermediates (OH*) coverage and optimized the potential-determining step. However, the study on Ir-based alloyed SACs for water splitting is very limited; owing to the high activity of Ir-based electrocatalysts, more attention is needed for alloying single atom Ir with other elements to boost water splitting activity, which will help the design of highly efficient water splitting catalysts.
To help the design of efficient HER catalysts, Zhou et al. conducted theoretical prediction of a series of binary/ternary metallic catalysts[45]. Considering the cost and stability and the location of single atoms, Ni-based catalysts with atomically dispersed Ni were predicted to be efficient for HER, as verified by the subsequent experimental study on the HER activity over the synthesized Ni-based alloyed SACs. Meanwhile, more experimental studies are needed to screen high-performance HER catalysts. Besides, a highly stable and active Co-substituted Ru nanosheet was synthesized[32] that demonstrated an extremely low overpotential of 13 mV at 10 mA·cm-2 in 1 M KOH. The ultralow Tafel slope of 29 mV·dec-1 outperformed those of other reported Pt-free electrocatalysts. DFT study revealed that the water dissociation energy barrier was greatly reduced after single Co atom substitution, which is the main reason for its superior HER performance. Another example is Co single atoms immobilized on Pd-based nanosheets[46], where the unsaturated coordination environments and unique electronic and geometric structures modulated the redistribution of charges and the d-band center, leading to highly active electronic states on the catalyst surface. The catalyst displayed outstanding HER activity in both alkaline and acid conditions, with a low overpotential of 24.7 mV at 10 mA·cm-2 and a Tafel slope of 8.2 mV·dec-1 in the acidic medium, which are superior to those of commercial Pd/C and Pt/C.
Nanoclusters prepared with atomic precision
Recently, catalytic application of metallic nanoclusters with atomic precision makes it possible for precise investigation of the structure-activity relationship[47]. Through single-atom doping/substitution, influence of single atoms in an alloy has been precisely studied for electrocatalytic HER[48,49]. Pt single atom substitution is the most widely investigated modification in nanoclusters for electrocatalytic HER[50-54]. For instance, Kwak et al. synthesized a bimetallic nanocluster of PtAu24(SC6H13)18 by single Pt atom substitution of
Figure 6. (A) Calculated reaction energies for HER over Pt1Au24 nanocluster. Figures are quoted with permission from ref[50]. Copyright 2017, Springer Nature; (B) Linear sweep voltammetry and (C) plots of turnover frequency vs. potential over different nanoclusters; (D) calculated ΔGH diagram for hydrogen adsorption with the order consistent to their HER activities; and (E) the optimized structure for different nanoclusters interacted with hydrogen. Figures are quoted with permission from ref[54], Copyright 2018, American Chemical Society. HER: Hydrogen evolution reaction.
Electrocatalytic OER
For electrocatalytic water splitting, oxygen evolution proceeds via a sluggish four-electron transfer process. Among the studied catalysts, Ru is compared to other noble metals relatively abundant, and RuO2 displays superior catalytic activity for OER compared with other noble metal oxide catalysts. However, the stability of RuO2 catalysts was not satisfied due to the dissolution of Ru via soluble ruthenate species. To enhance the stability of Ru-based catalysts for OER, Yao et al. synthesized isolated Ru atoms over a Pt-Cu host
Figure 7. (A-C) Elemental mapping of Ru1-Pt3Cu with atomic resolution; (D) the corresponding schematic atom model; (E) overpotential for the catalysts to reach 0.1 and 10 mA·cm-2; (F) chronopotentiometric tests for Ru1-Pt3Cu and RuO2 at 10 mA·cm-2; (G) calculated volcano plot for the OER overpotential. Figures are quoted with permission from ref[31], Copyright 2019, Springer Nature; (H) The calculated profiles for the free energy during OER over different catalyst surfaces. Figures are quoted with permission from ref[34], Copyright 2020, Royal Society of Chemistry. OER: Oxygen evolution reaction.
Non-noble transition metals are normally used for OER especially under alkaline conditions[56], which can remarkably reduce the catalyst cost. After further modification by noble metal with atomic dispersion, the coordination environments were optimized for enhancing the OER catalytic performance. Lee et al. managed to disperse Ru single atoms over the CoFe2 surface to get the catalyst named RuSACoFe2/G, which demonstrated a much lower overpotential of 180 mV at 10 mA·cm-2 than those of RuO2 and CoFe2 (298 and 313 mV, respectively)[34]. The enhanced catalytic performance of RuSACoFe2/G has been ascribed to the reduced energy barrier for the rate-limiting step, with the isolated Ru atoms stabilizing the OOH* intermediate and oxygen species pre-adsorbed on Co-Fe [Figure 7H].
Other than Ru-based catalysts, isolated Ir atoms were also verified to display excellent OER activity[57,58]. With isolated Ir atoms anchored onto 3D amorphous NiFe nanowire@nanosheets to form the NiFeIrx/Ni catalysts, over 12 h long-term stability and decreased overpotential of 200 mV at 10 mA·cm-2 were obtained[57]. The significantly enhanced OER performance has been attributed to the synergy effect between isolated Ir atoms on NiFe/Ni support. Owing to the stabilization of OOH* species and the synergy effect between Ir-Co dual-sites, Ir single atoms-decorated Co nanoparticles have been reported to show superior OER activity than monometallic Ir catalysts[58]. These studies demonstrated that the formation of alloyed single atoms can display superior electrocatalytic OER activity.
Up to now, the study on alloyed SACs for OER is very limited; continued efforts are urgently needed for high-performance OER catalysts with isolated and alloyed metal sites. To improve the activity and stability of the catalysts, special support can be chosen; for example, a recent study showed that metal-organic frameworks (MOFs) were promising for stabilizing bimetallic atoms with atomic precision for OER[59].
Photocatalytic HER
Solar-driven water splitting to produce hydrogen and oxygen is among the possible avenues for sustainable and carbon-free energy generation[29], which can simultaneously alleviate the current environmental and energy issues. The formation of alloyed Pt single atoms with other metal elements has been reported to demonstrate high efficiency for solar-driven HER[60-62]. By using a one-unit-cell of ZnIn2S4 (ZIS) nanosheet with abundant Cu as dopants, a single atom Pt alloyed Cu catalyst (Pt1/Cu-ZIS) was obtained [Figure 8A-C], which showed a notable solar-driven HER rate of 5.02 mmol·g-1·h-1; the value is nearly 5, 15, and 48 times higher than those of Ptnc/ZIS, Cu-ZIS, and pristine ZIS, respectively[60]. Control experiments, combined with various advanced characterizations and DFT calculations, confirmed that Cu dopants can not only stabilize the isolated Pt atoms, but also promote the photogenerated electron transfer efficiency. Meanwhile, Cu dopants acted as surface trap centers to capture photogenerated electrons from the conduction band after photoexcitation [Figure 8D-G]. Thus, the formation of alloyed Pt single atoms with Cu serves as the electron transfer channel to enhance the electron transfer for driving water splitting. Another example comes from a bimetallic nanoparticles/MOF system[61], where Pt single atoms are alloyed with Pd to form Pd@Pt/UiO-66-NH2. The Pt shell on Pd can be precisely controlled, with the structure varying from core-shell to single atom distribution, thus changing the coordination environment of Pt. Consequently, the surface charge of Pd and Pt can be redistributed [Figure 8H], which optimized the electronic state of the active sites to enhance photocatalytic HER activity.
Figure 8. (A) Atomic-resolution HAADF-STEM image of Pt1/Cu-ZIS with white circle showing the Pt single atoms; (B) representative HAADF-STEM image with (C) the intensity profile of the dark cyan arrow in (B); (D and E) the crystal structure of Pt1/Cu-ZIS, Mulliken charge analysis and the density difference of Pt atoms adsorbed over (F) ZIS and (G) Cu-ZIS. Figures are quoted with permission from ref[60], Copyright 2021, Wiley-VCH; (H) Calculated differential charge density indicated the charge redistribution of the Pd@Pt nanoparticles with SAA structure and core-shell structure of different Pt layers. Figures are quoted with permission from ref[61], Copyright 2021, Natl. Sci. Rev. HAADF-STEM: High-angle annular dark field scanning transmission electron microscopy; ZIS: ZnIn2S4; SAA: single-atom alloy.
Besides the conventional Pt-based co-catalysts, bimetallic nanoclusters with atomic precision have received increasing attention[47]. For instance, the work from Du et al. demonstrated enhanced photocatalytic HER activity over Pt1Ag24(SR)18/g-C3N4 [Figure 9A]; the hydrogen evolution rate was significantly higher than those of pristine g-C3N4 and Ag24(SR)18/g-C3N4 (330 and four times, respectively)[62] [Figure 9B]. The semicircle in the Nyquist plot for Pt1Ag24/g-C3N4 was significantly reduced [Figure 3A], suggesting a small transfer barrier of photogenerated charges, indicating that Pt single atom doping can seriously optimizing the photogenerated charge carrier dynamics, thus enhancing the photocatalytic HER activity of Ag24(SR)18/g-C3N4. With Ag19Pt1 as a model, investigation on quantum dynamics for the photogenerated charges and structural dynamics for plasmon-assisted solar-driven water splitting was undertaken at the atomic length scale and femtosecond time scale[63], which demonstrated that the interaction of water with the nanocluster would be enhanced mainly due to Pt single atom substitution on tip atoms of the tetrahedral Ag20 nanocluster. The photogenerated electron transfer would also be enhanced, thus accelerating the charge transfer to enhance water splitting activity.
Figure 9. (A) The possible photocatalytic H2 evolution mechanism; (B) photocatalytic HER performance of different catalysts. Figures are quoted with permission from ref[62], Copyright 2017, the Royal Society of Chemistry; (C) Proposed structures of the catalysts before (top) and during (bottom) water splitting process; (D) Photocatalytic water splitting for the generation of H2 and O2 over different catalysts. Figures are quoted with permission from ref[64], Copyright 2019, American Chemical Society. HER: Hydrogen evolution reaction.
Charge transfer can be promoted by optimizing the interface between the semiconductor and co-catalyst, which will reduce the recombination of photogenerated electron-hole pairs. By loading Pt1Ag24(SR)18 or
However, results might be confusing for the Ag25 nanoclusters; when doped with Pt, Pd and Au and then loaded onto TiO2, the catalytic activity follows an order of Ag25 ≥ PtAg24 > PdAg24 ≥ AuAg24[65]. With the geometric structure well-preserved after doping, the electronic structures of the nanoclusters were significantly altered. DFT calculations and ultraviolet photoelectron spectroscopy (UPS) measurements led to the conclusion that the mismatched energy levels between TiO2 and the doped nanoclusters were responsible for the lower activities. While, further detailed study is still needed for the underpinning reasons for the activity difference.
Besides the above-mentioned studies, less attention has been paid to photocatalytic OER. Compared to light-driven HER, photocatalytic OER would be more difficult. Transition metal oxide co-catalysts are normally efficient for photocatalytic OER. Thus, future study of alloyed single atom structures loaded onto such metal oxides might enhance the photocatalytic OER performance.
CONCLUSION AND OUTLOOK
Catalysts that alloy single metal atoms with another host metal offer the dual merits of maximizing the metal atom utilization of SACs and the synergy effect of metallic alloy catalysts. This combination can lead to significantly enhanced catalytic activity and improved long-term stability for water splitting to produce hydrogen and oxygen. The principle of alloyed SACs for their enhanced catalytic performance for electrocatalytic HER/OER and photocatalytic HER was especially discussed and compared to gain insight into the catalytic mechanism. However, despite many studies conducted on these catalysts for water splitting, multiple factors still need to be addressed to make them viable for practical application.
1. To control and maintain the uniform atomic distribution of single atoms in alloyed SAC is still challenging, though many synthetic strategies have been developed. Novel methods for simple and large-scale production of alloyed SACs are still needed urgently. Additionally, special attention needs to be paid to the deposition of the catalysts to make them more active for electrocatalysis.
2. Obviously, most studies for water splitting over alloyed SACs focus on HER; limited attention has been paid to OER, especially under photocatalytic conditions. Much more attention should be paid to developing alloyed SACs for electro/photocatalytic OER.
3. Current studies on the kinds of alloyed SACs are still very limited. Rational design and extension of other metal elements (i.e., Ir, Fe) and multi-metal alloys (i.e., high-entropy alloy)-based catalysts hold great potential for highly efficient water splitting reaction.
4. Besides the structure characterization, in situ/operando study of the structure changing of the catalysts and the gaseous products evolution of water splitting reaction are important for optimizing the activity/stability of specific catalysts and understanding the catalytic mechanism.
5. In addition to traditional theoretical prediction, machine learning and artificial intelligence (AI) are recently emerging computer-related approaches that are promising for providing theoretical guidance for rational design and catalytic mechanism clarification of alloyed SACs; special attention is needed for these aspects.
Although many challenges remained, increased enthusiasm for investigating alloyed SACs for water splitting holds promise for the development of novel catalysts to enhance the catalytic performance, which is expected to lead to substantial improvements and innovations in catalytic technologies for sustainable energy solutions.
DECLARATIONS
Authors’ contributions
Investigated all the literature and designed and wrote the manuscript: Pei, G. X.
Contributed to the critical discussion for revising the manuscript: Qi, H.; Hofmann, J. P.
The final version of the manuscript has been approved by all authors.
Availability of data and materials
Not applicable.
Financial support and sponsorship
This work was supported by the Natural Science Foundation of Shandong Province (No. ZR2023QB178). Qi, H. thanks the Marie Skłodowska-Curie Actions Postdoctoral Fellowships (101107009-AtomCat4Fuel) and UKRI (EP/Y029305/1).
Conflicts of interest
All authors declared that there are no conflicts of interest.
Ethical approval and consent to participate
Not applicable.
Consent for publication
Not applicable.
Copyright
© The Author(s) 2025.
REFERENCES
1. Miao, L.; Jia, W.; Cao, X.; Jiao, L. Computational chemistry for water-splitting electrocatalysis. Chem. Soc. Rev. 2024, 53, 2771-807.
2. Wei, S.; Sacchi, R.; Tukker, A.; Suh, S.; Steubing, B. Future environmental impacts of global hydrogen production. Energy. Environ. Sci. 2024, 17, 2157-72.
3. Zhao, L.; Wang, S.; Liang, S.; An, Q.; Fu, J.; Hu, J. Coordination anchoring synthesis of high-density single-metal-atom sites for electrocatalysis. Coord. Chem. Rev. 2022, 466, 214603.
4. Nakaya, Y.; Furukawa, S. Catalysis of alloys: classification, principles, and design for a variety of materials and reactions. Chem. Rev. 2023, 123, 5859-947.
5. Liu, L.; Corma, A. Bimetallic sites for catalysis: from binuclear metal sites to bimetallic nanoclusters and nanoparticles. Chem. Rev. 2023, 123, 4855-933.
6. Yun, Q.; Lu, Q.; Li, C.; et al. Synthesis of PdM (M = Zn, Cd, ZnCd) nanosheets with an unconventional face-centered tetragonal phase as highly efficient electrocatalysts for ethanol oxidation. ACS. Nano. 2019, 13, 14329-36.
7. Zhang, Z.; Liu, G.; Cui, X.; et al. Crystal phase and architecture engineering of lotus-thalamus-shaped Pt-Ni anisotropic superstructures for highly efficient electrochemical hydrogen evolution. Adv. Mater. 2018, 30, 1801741.
8. Zhu, J.; Elnabawy, A. O.; Lyu, Z.; et al. Facet-controlled Pt–Ir nanocrystals with substantially enhanced activity and durability towards oxygen reduction. Mater. Today. 2020, 35, 69-77.
9. Kyriakou, G.; Boucher, M. B.; Jewell, A. D.; et al. Isolated metal atom geometries as a strategy for selective heterogeneous hydrogenations. Science 2012, 335, 1209-12.
10. Hannagan, R. T.; Giannakakis, G.; Flytzani-Stephanopoulos, M.; Sykes, E. C. H. Single-atom alloy catalysis. Chem. Rev. 2020, 120, 12044-88.
11. Mao, J.; Yin, J.; Pei, J.; Wang, D.; Li, Y. Single atom alloy: an emerging atomic site material for catalytic applications. Nano. Today. 2020, 34, 100917.
12. Zhang, T.; Walsh, A. G.; Yu, J.; Zhang, P. Single-atom alloy catalysts: structural analysis, electronic properties and catalytic activities. Chem. Soc. Rev. 2021, 50, 569-88.
13. Sun, X.; Song, Y.; Jiang, G.; Lan, X.; Xu, C. Fundamentals and catalytic applications of single-atom alloys. Sci. China. Mater. 2024, 67, 1-17.
14. Pei, G.; Liu, X.; Chai, M.; Wang, A.; Zhang, T. Isolation of Pd atoms by Cu for semi-hydrogenation of acetylene: effects of Cu loading. Chin. J. Catal. 2017, 38, 1540-8.
15. Pei, G. X.; Liu, X. Y.; Yang, X.; et al. Performance of Cu-alloyed Pd single-atom catalyst for semihydrogenation of acetylene under simulated front-end conditions. ACS. Catal. 2017, 7, 1491-500.
16. Pei, G. X.; Liu, X. Y.; Wang, A.; et al. Ag alloyed Pd single-atom catalysts for efficient selective hydrogenation of acetylene to ethylene in excess ethylene. ACS. Catal. 2015, 5, 3717-25.
17. Pei, G. X.; Liu, X. Y.; Wang, A.; et al. Promotional effect of Pd single atoms on Au nanoparticles supported on silica for the selective hydrogenation of acetylene in excess ethylene. New. J. Chem. 2014, 38, 2043.
18. Shen, T.; Wang, S.; Zhao, T.; Hu, Y.; Wang, D. Recent advances of single-atom-alloy for energy electrocatalysis. Adv. Energy. Mater. 2022, 12, 2201823.
19. Da, Y.; Jiang, R.; Tian, Z.; Han, X.; Chen, W.; Hu, W. The applications of single-atom alloys in electrocatalysis: progress and challenges. SmartMat 2023, 4, e1136.
20. Zhuang, J.; Wang, D. Recent advances of single-atom alloy catalyst: properties, synthetic methods and electrocatalytic applications. Mater. Today. Catal. 2023, 2, 100009.
21. Gao, Q.; Han, X.; Liu, Y.; Zhu, H. Electrifying energy and chemical transformations with single-atom alloy nanoparticle catalysts. ACS. Catal. 2024, 14, 6045-61.
22. Ahmed, M.; Wang, C.; Zhao, Y.; et al. Bridging together theoretical and experimental perspectives in single-atom alloys for electrochemical ammonia production. Small 2024, 20, 2308084.
23. Gao, Q.; Yao, B.; Pillai, H. S.; et al. Synthesis of core/shell nanocrystals with ordered intermetallic single-atom alloy layers for nitrate electroreduction to ammonia. Nat. Synth. 2023, 2, 624-34.
24. Cao, Y.; Chen, S.; Bo, S.; et al. Single atom Bi decorated copper alloy enables C-C coupling for electrocatalytic reduction of CO2 into C2+ products. Angew. Chem. Int. Ed. Engl. 2023, 62, 202303048.
25. Wang, J.; Xin, S.; Xiao, Y.; et al. Manipulating the water dissociation electrocatalytic sites of bimetallic nickel-based alloys for highly efficient alkaline hydrogen evolution. Angew. Chem. Int. Ed. Engl. 2022, 61, 202202518.
26. Wang, H.; Zhang, K. H. L.; Hofmann, J. P.; de la Peña O’shea, V. A.; Oropeza, F. E. The electronic structure of transition metal oxides for oxygen evolution reaction. J. Mater. Chem. A. 2021, 9, 19465-88.
27. Hansen, J. N.; Prats, H.; Toudahl, K. K.; et al. Is there anything better than Pt for HER? ACS. Energy. Lett. 2021, 6, 1175-80.
28. Zhu, J.; Hu, L.; Zhao, P.; Lee, L. Y. S.; Wong, K. Y. Recent advances in electrocatalytic hydrogen evolution using nanoparticles. Chem. Rev. 2020, 120, 851-918.
29. Zhang, Z.; Yates, J. T. Jr. Band bending in semiconductors: chemical and physical consequences at surfaces and interfaces. Chem. Rev. 2012, 112, 5520-51.
30. Ding, C.; Shi, J.; Wang, Z.; Li, C. Photoelectrocatalytic water splitting: significance of cocatalysts, electrolyte, and interfaces. ACS. Catal. 2017, 7, 675-88.
31. Yao, Y.; Hu, S.; Chen, W.; et al. Engineering the electronic structure of single atom Ru sites via compressive strain boosts acidic water oxidation electrocatalysis. Nat. Catal. 2019, 2, 304-13.
32. Mao, J.; He, C. T.; Pei, J.; et al. Accelerating water dissociation kinetics by isolating cobalt atoms into ruthenium lattice. Nat. Commun. 2018, 9, 4958.
33. Greiner, M. T.; Jones, T. E.; Beeg, S.; et al. Free-atom-like d states in single-atom alloy catalysts. Nat. Chem. 2018, 10, 1008-15.
34. Lee, J.; Kumar, A.; Yang, T.; et al. Stabilizing the OOH* intermediate via pre-adsorbed surface oxygen of a single Ru atom-bimetallic alloy for ultralow overpotential oxygen generation. Energy. Environ. Sci. 2020, 13, 5152-64.
35. Li, M.; Duanmu, K.; Wan, C.; et al. Single-atom tailoring of platinum nanocatalysts for high-performance multifunctional electrocatalysis. Nat. Catal. 2019, 2, 495-503.
36. Zhu, Y.; Zhu, X.; Bu, L.; et al. Single-atom in-doped subnanometer Pt nanowires for simultaneous hydrogen generation and biomass upgrading. Adv. Funct. Mater. 2020, 30, 2004310.
37. Zeng, L.; Zhao, Z.; Huang, Q.; et al. Single-atom Cr-N4 sites with high oxophilicity interfaced with Pt atomic clusters for practical alkaline hydrogen evolution catalysis. J. Am. Chem. Soc. 2023, 145, 21432-41.
38. Ding, J.; Ji, Y.; Li, Y.; Hong, G. Monoatomic platinum-embedded hexagonal close-packed nickel anisotropic superstructures as highly efficient hydrogen evolution catalyst. Nano. Lett. 2021, 21, 9381-7.
39. Huo, L.; Jin, C.; Tang, J.; et al. Ultrathin NiPt single-atom alloy for synergistically accelerating alkaline hydrogen evolution. ACS. Appl. Energy. Mater. 2022, 5, 15136-45.
40. Luo, M.; Cai, J.; Zou, J.; Jiang, Z.; Wang, G.; Kang, X. Promoted alkaline hydrogen evolution by an N-doped Pt–Ru single atom alloy. J. Mater. Chem. A. 2021, 9, 14941-7.
41. Zhang, L.; Liu, H.; Liu, S.; et al. Pt/Pd single-atom alloys as highly active electrochemical catalysts and the origin of enhanced activity. ACS. Catal. 2019, 9, 9350-8.
42. Chen, C.; Wu, D.; Li, Z.; et al. Ruthenium-based single-atom alloy with high electrocatalytic activity for hydrogen evolution. Adv. Energy. Mater. 2019, 9, 1803913.
43. Tong, Y.; Liu, J.; Wang, L.; et al. Carbon-shielded single-atom alloy material family for multi-functional electrocatalysis. Adv. Funct. Mater. 2022, 32, 2205654.
44. Wang, B.; Li, J.; Li, D.; et al. Single atom iridium decorated nickel alloys supported on segregated MoO2 for alkaline water electrolysis. Adv. Mater. 2024, 36, e2305437.
45. Zhou, C.; Zhao, J. Y.; Liu, P. F.; et al. Towards the object-oriented design of active hydrogen evolution catalysts on single-atom alloys. Chem. Sci. 2021, 12, 10634-42.
46. Yang, S.; Si, Z.; Li, G.; et al. Single cobalt atoms immobilized on palladium-based nanosheets as 2D single-atom alloy for efficient hydrogen evolution reaction. Small 2023, 19, e2207651.
47. Jin, R.; Li, G.; Sharma, S.; Li, Y.; Du, X. Toward active-site tailoring in heterogeneous catalysis by atomically precise metal nanoclusters with crystallographic structures. Chem. Rev. 2021, 121, 567-648.
48. Walsh, A. G.; Zhang, P. Thiolate-protected single-atom alloy nanoclusters: correlation between electronic properties and catalytic activities. Adv. Mater. Inter. 2021, 8, 2001342.
49. Kumar, B.; Kawawaki, T.; Shimizu, N.; et al. Gold nanoclusters as electrocatalysts: size, ligands, heteroatom doping, and charge dependences. Nanoscale 2020, 12, 9969-79.
50. Kwak, K.; Choi, W.; Tang, Q.; et al. A molecule-like PtAu24(SC6H13)18 nanocluster as an electrocatalyst for hydrogen production. Nat. Commun. 2017, 8, 14723.
51. Kwak, K.; Choi, W.; Tang, Q.; Jiang, D.; Lee, D. Rationally designed metal nanocluster for electrocatalytic hydrogen production from water. J. Mater. Chem. A. 2018, 6, 19495-501.
52. Li, X.; Takano, S.; Tsukuda, T. Ligand effects on the hydrogen evolution reaction catalyzed by Au13 and Pt@Au12: alkynyl vs thiolate. J. Phys. Chem. C. 2021, 125, 23226-30.
53. Hu, G.; Tang, Q.; Lee, D.; Wu, Z.; Jiang, D. Metallic hydrogen in atomically precise gold nanoclusters. Chem. Mater. 2017, 29, 4840-7.
54. Choi, W.; Hu, G.; Kwak, K.; et al. Effects of metal-doping on hydrogen evolution reaction catalyzed by MAu24 and M2Au36 nanoclusters (M = Pt, Pd). ACS. Appl. Mater. Interfaces. 2018, 10, 44645-53.
55. Jo, Y.; Choi, M.; Kim, M.; Yoo, J. S.; Choi, W.; Lee, D. Promotion of alkaline hydrogen production via Ni-doping of atomically precise Ag nanoclusters. Bulletin. Korean. Chem. Soc. 2021, 42, 1672-7.
56. Ding, H.; Liu, H.; Chu, W.; Wu, C.; Xie, Y. Structural transformation of heterogeneous materials for electrocatalytic oxygen evolution reaction. Chem. Rev. 2021, 121, 13174-212.
57. Luo, X.; Wei, X.; Zhong, H.; et al. Single-atom Ir-anchored 3D amorphous NiFe nanowire@nanosheets for boosted oxygen evolution reaction. ACS. Appl. Mater. Interfaces. 2020, 12, 3539-46.
58. Babu, D. D.; Huang, Y.; Anandhababu, G.; et al. Atomic iridium@cobalt nanosheets for dinuclear tandem water oxidation. J. Mater. Chem. A. 2019, 7, 8376-83.
59. Mu, X.; Yu, M.; Liu, X.; et al. High-entropy ultrathin amorphous metal–organic framework-stabilized Ru(Mo) dual-atom sites for water oxidation. ACS. Energy. Lett. 2024, 9, 5763-70.
60. Su, L.; Wang, P.; Wang, J.; et al. Pt–Cu interaction induced construction of single Pt sites for synchronous electron capture and transfer in photocatalysis. Adv. Funct. Mater. 2021, 31, 2104343.
61. Pan, Y.; Qian, Y.; Zheng, X.; et al. Precise fabrication of single-atom alloy co-catalyst with optimal charge state for enhanced photocatalysis. Natl. Sci. Rev. 2021, 8, nwaa224.
62. Du, X. L.; Wang, X. L.; Li, Y. H.; et al. Isolation of single Pt atoms in a silver cluster: forming highly efficient silver-based cocatalysts for photocatalytic hydrogen evolution. Chem. Commun. 2017, 53, 9402-5.
63. Zhang, Y.; Chen, D.; Meng, W.; Li, S.; Meng, S. Plasmon-induced water splitting on Ag-alloyed Pt single-atom catalysts. Front. Chem. 2021, 9, 742794.
64. Kurashige, W.; Hayashi, R.; Wakamatsu, K.; et al. Atomic-level understanding of the effect of heteroatom doping of the cocatalyst on water-splitting activity in AuPd or AuPt alloy cluster-loaded BaLa4Ti4O15. ACS. Appl. Energy. Mater. 2019, 2, 4175-87.
Cite This Article
How to Cite
Download Citation
Export Citation File:
Type of Import
Tips on Downloading Citation
Citation Manager File Format
Type of Import
Direct Import: When the Direct Import option is selected (the default state), a dialogue box will give you the option to Save or Open the downloaded citation data. Choosing Open will either launch your citation manager or give you a choice of applications with which to use the metadata. The Save option saves the file locally for later use.
Indirect Import: When the Indirect Import option is selected, the metadata is displayed and may be copied and pasted as needed.
About This Article
Special Issue
Copyright
Data & Comments
Data
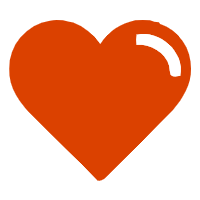
Comments
Comments must be written in English. Spam, offensive content, impersonation, and private information will not be permitted. If any comment is reported and identified as inappropriate content by OAE staff, the comment will be removed without notice. If you have any queries or need any help, please contact us at [email protected].