Metal-zeolite catalysts promoting low-temperature methane oxidation to oxygenates
Abstract
Catalytic conversion of methane (CH4) into value-added chemicals provides a viable path to reduce dependency on crude oil. Despite the challenges associated with activating methane’s C–H bond and limiting side reactions, low-temperature oxidation of methane to oxygenates has emerged as a promising approach, often hailed as a “grail reaction”. Zeolite-based metal (metal-zeolite) catalysts facilitate methane oxidation at low temperatures, converting methane into oxygenates while minimizing the complete oxidation to carbon dioxide (CO2). This review highlights recent achievements in metal-zeolite catalysts for methane partial and coupling oxidation. With zeolite as the core, we explore the synthesis methods, metallic active sites, reaction mechanisms, and zeolite descriptors of metal-zeolite catalysts for methane partial oxidation. Additionally, we examine the critical role of mono- and bi-metallic species in metal-zeolite catalysts for methane coupling oxidation with carbon monoxide (CO). Finally, we discuss the challenges and opportunities for metal-zeolite catalysts in methane oxidation under mild conditions, proposing future directions for rational design of metal-zeolite catalysts, revealing reaction mechanisms through operando or in situ techniques, and leveraging artificial intelligence (AI) for enhanced catalytic efficiency.
Keywords
INTRODUCTION
Natural gas, containing 70%-90% methane (CH4), is estimated to have global reserves exceeding 190 trillion cubic meters and is recognized as a clean and abundant resource[1,2]. Conventional methane utilization relies on reforming and Fischer-Tropsch processes, which are both energy-intensive and costly[3,4]. Recently, low-temperature methane oxidation to oxygenates, including methanol (CH3OH), formic acid (HCOOH), and acetic acid (CH3COOH), has been identified as an ideal alternative pathway[5]. These methane-derived oxygenated products are significant platform compounds for producing commodity chemicals, which enhances natural gas utilization efficiency and reduces dependence on petroleum-based chemicals[6-11]. However, the generated oxygenates are more reactive than methane and can be easily over-oxidized to carbon monoxide (CO) and carbon dioxide (CO2), posing a challenge for high activity and selectivity[12]. Thus, highly active and selective metal species are essential for efficient low-temperature methane oxidation. Various homogeneous and heterogeneous catalysts, including metal oxides[13,14], metal complex[15-17], and metal-organic frameworks (MOFs)[18,19], have been used for low-temperature methane oxidation in the presence of N2O or H2O2 as oxidants[20,21]. Compared to the above-mentioned catalytic systems, zeolite materials provide unique spatial nanoconfinement effects within their microporous channels for constructing enzyme-like metal species with high activity, surpassing most homogeneous and heterogeneous metal-containing catalysts for low-temperature methane oxidation[22].
Zeolites are crystalline materials with uniform micropores (typically less than 2 nm), high surface areas, tunable framework compositions, and good (hydro)thermal stabilities, which are constructed by corner-sharing TO4 tetrahedra (T represents Si, Al, P, etc.)[23]. The TO4 tetrahedra can be connected in different ways to form various zeolite topologies, each of which is assigned a three-letter code by the International Zeolite Association (IZA)[24]. In this review article, chabazite (CHA), ferrierite (FER), mordenite (MOR), faujasite (FAU), and Mobil-five (MFI) are mainly discussed. Zeolites are widely used for industrial applications in catalysis and separation[25]. Incorporation of trivalent metal atoms, such as Al and Ga, into the zeolite lattice yields a negatively charged framework, which is balanced by extra-framework cations or protons toward electrical neutrality. Diverse metallic species can be incorporated into zeolite channels via ion exchange or in situ synthesis methods. The isolation of metal cations at ion-exchange (IE) sites and the unique spatial nanoconfinement effect in zeolites impart metallic species with unique electronic structures and thus distinctive catalytic performances. The microporous structure of zeolite effectively restricts side reactions, thus enhancing the yield of the desired products[26]. Additionally, the strong acidity of zeolites plays a crucial role in light alkane conversions[27,28]. Specifically, iron (Fe) and copper (Cu) ions within zeolites exhibit remarkable activity in the methane conversion to oxygenates. Furthermore, zeolite-encapsulated metal clusters and nanoparticles (e.g., Au, AuPd, and Pd) have been employed to achieve high activity for the production of methanol from methane[29-32]. Zeolite-based metal (metal-zeolite) catalysts significantly promote the conversion of methane to oxygenates, positioning them as highly promising candidates for catalysis[33-40]. Methane oxidation establishes the links between natural gas, flare gas, shale gas, biogas and value-added chemicals such as olefin, fuel, solvent, etc., which is significant for pursuing a sustainable supply of chemicals and energy[41] [Figure 1].
Figure 1. Schematic of catalytic processes of methane oxidation to produce oxygenates under (left) gas and (right) liquid phases over metal-zeolite catalysts.
In this review, recent advancements in metal-zeolite catalysts containing various metallic species for low-temperature methane oxidation were summarized. We provide a comprehensive overview of synthesis methods, metallic active sites, reaction mechanisms, and zeolite descriptors that promote both direct methane oxidation and methane coupling oxidation with CO to form oxygenates. This highlights the efficient catalytic system arising from the synergy between metal species and zeolite framework. The outlook section addresses challenges and opportunities faced by metal-zeolite catalysts in catalyzing low-temperature methane oxidation, aiming to advance research in low-carbon alkane oxidation and inspire the improvement of metal-zeolite catalysts.
METHANE TO OXYGENATES OVER METAL-ZEOLITE CATALYSTS
Low-temperature methane oxidation to oxygenates has garnered significant interest as a potential route for substantially reducing the processing cost[42-47]. Over the past two decades, several processes have been developed: (i) continuous conversion under moderate conditions using metal-zeolite catalysts with N2O/
Fe-containing zeolite catalysts
Modulating synthesis methods to promote low-temperature methane oxidation
It is widely recognized that mononuclear and binuclear Fe located at the IE sites exhibit exceptional activity for the oxidation of methane in hydrogen peroxide (H2O2) systems, owing to their structural similarity to methane monooxygenase[28]. In addition to active mononuclear and binuclear species, metallic clusters and nanoparticles are experimentally confirmed to exist in Fe-containing zeolites, which display limited activity in low-temperature methane oxidation. Therefore, it is crucial to deliberately increase the proportion of active species during catalyst design. The synthesis method of metal-zeolite catalysts significantly influences the active species and thus the catalyst activity and selectivity.
Post-treatment strategy
Various post-treatment methods have been employed to prepare metal-containing zeolites[60,76]. These methods include wet impregnation (WI), aqueous phase IE, and solid-state ion-exchange (SSIE). Taking Fe-containing zeolite catalysts as an example, compared with IE and SSIE methods, the WI method generally leads to the formation of larger metal species with poor dispersion [Figure 2]. In 2012, Hammond et al. reported that Fe-containing ZSM-5 zeolites synthesized by SSIE enabled partial methane oxidation to oxygenates (primarily HCOOH) using H2O2 as an oxidant[77]. Additionally, Fe/MOR zeolite consisting of binuclear Fe sites was also successfully prepared using the SSIE method[78]. Similarly, Yu et al. compared Fe/ZSM-5 catalysts synthesized by WI, IE, and SSIE methods[79]. They employed scanning transmission electron microscopy (STEM), ultraviolet-visible diffuse reflectance (UV-vis DR), and 57Fe Mössbauer spectroscopy for visualization and quantitative analysis of Fe species and provided a semi-quantitative estimation of their distribution. The proportion of mononuclear Fe species decreases in the order of Fe/ZSM-5SSIE > Fe/ZSM-5IE > Fe/ZSM-5WI. The results indicated that the WI and IE processes led to unavoidable aggregation of Fe species during the hydrolysis and drying processes under an aqueous condition. In contrast, the SSIE method mitigated these issues by employing the solvent-free synthesis process, which effectively suppressed the aggregation of Fe species and prevented the formation of large Fe oxides. In another work, Kim et al. compared different synthesis methods and concluded that the IE method produced isolated or oligonuclear extra-framework Fe species more effectively than SSIE, chemical vapor infiltration (CVI), hydrothermal synthesis, and WI methods[80]. Consequently, the IE samples showed superior catalytic activity compared to those synthesized by other techniques. It should be noted that the differences in particle size, hierarchical structure, framework Si/Al ratio, location of framework Al atoms of zeolite supports significantly affect the nuclearity and distribution of extra-framework metallic species that are introduced via post-treatment methods. In addition, with rising Fe content of Fe-ZSM-5 catalyst synthesized by post-treatment strategy, the proportion of low-activity Fe clusters and nanoparticles would increase, leading to metal aggregation on the crystal surface and adversely affecting the catalyst performance.
Figure 2. Schematic illustration of post-treatment and in situ synthesis methods of Fe-containing zeolite catalysts. Compared to IE, SSIE, and in situ synthesis methods, the WI method tends to cause metal particle agglomeration. IE: Ion-exchange; SSIE: solid-state ion-exchange; WI: wet impregnation.
In situ strategy
In situ introduction method enhances Fe dispersion and increases the proportion of active species. For instance, Cheng et al. introduced Fe source into the seed-directed template-free synthesis system of ZSM-5 zeolite (Fe-HZ5-TF)[81]. This organic-template-free approach enabled introduction of more Fe atoms into the zeolite framework. These framework Fe atoms have a strong propensity to migrate out of the framework to form isolated extra-framework Fe species under thermal treatment, leading to the formation of Fe-HZ5-TF catalyst (0.39 wt% of Fe loading). Overall, Fe-HZ5-TF demonstrated three times the number of active sites compared to conventional Fe-ZSM-5 catalysts. The resultant Fe-HZ5-TF achieved a high C1 oxygenate yield of 109.4 mmol·gcat-1·h-1 during methane oxidation using 0.5 M hydrogen peroxide at 75 °C. Recently, Zhang et al. adopted an amino acid-assisted hydrothermal synthesis method to prepare a framework aluminum-rich ZSM-5 zeolite with a Si/Al ratio of 9 (Z-L0.3H6-9)[82]. The Fe source was directly introduced to the synthesis system of Z-L0.3H6-9 zeolite, leading to 0.44Fe@Z-L0.3H6-9 catalysts (0.44 represents the Fe loading of 0.44 wt%). The resultant 0.44Fe@Z-L0.3H6-9, consisting of 73% mononuclear Fe3+ and 27% binuclear Fe3+ species, exhibited exceptional oxygenate yield and turnover frequency (TOF) of
Modulating zeolite descriptors to promote methane conversion
Framework trivalent atoms
The active Fe3+ species in zeolites are typically anchored by negatively charged MO4 (M = Al, Ga) tetrahedra in the zeolite framework. Therefore, it is highly desirable to increase the concentration of framework trivalent atoms, which is effective in maximizing mononuclear and/or binuclear Fe3+ sites[25]. Hammond
Figure 3. SEM images of ZSM-5 zeolites with varied Si/Al ratios of [A(i)] 12.4, [A(ii)] 22.0, [A(iii)] 50.3, and [A(iv)] 19.6; (B) Comparisons of the catalytic performances of various Fe/ZSM-5 samples. Reprinted with permission from ref[84]. Copyright 2022. The Royal Society of Chemistry; (C) Proposed reaction pathway controlling the oxidation of methane into methanol and formic acid using
Extra-framework copper cations
Copper ions (Cu2+) exhibit a synergistic effect with Fe species in zeolite, enhancing methanol selectivity[85]. Fang et al. demonstrated that the addition of Cu2+ effectively enhances the methanol selectivity towards 71.3%[78]. This is because Cu2+ reacts with hydroxyl radicals to prevent the over-oxidation of methanol, thereby promoting methanol selectivity [Figure 3C]. Kim et al. investigated the copper-promoted Fe/ZSM-5 catalyst by preparing Cu/ZSM-5, Cu-Fe/ZSM-5, and Fe/ZSM-5 using WI, SSIE, and IE methods [Figure 3D and E][86]. Their study examined the influence of copper species on methane oxidation in H2O2. Characterization of the catalysts through UV-vis spectroscopy, nitrogen physical adsorption, hydrogen programmed temperature reduction, and Fourier transform infrared (FTIR) spectroscopy confirmed that copper promoted the methanol selectivity over the Cu-Fe/ZSM-5 catalyst. The resultant Cu/ZSM-5 exhibited low catalytic activity, with oxygenate product yield below 0.05 and 0.4 mmol at 30 and 50 °C, respectively [Figure 3D and E]. This indicates that Cu ions are not the active sites for methane conversion in the aqueous H2O2 solution. Fe/ZSM-5 prepared via IE method showed an enhanced oxygenate yield
Pore window size of zeolites
Zeolite pore apertures influence the activity of metallic species, diffusion efficiency of molecules, and product selectivity[87-90]. This is because the spatial nanoconfinement effects differ in different zeolite topologies, which can significantly alter the intermediate stability and reaction kinetics. Recently, Snyder
Active sites and reaction mechanisms of Fe-containing zeolites
The active site was initially proposed as an extra-framework binuclear Fe species, inspired by methane monooxygenase[52]. This hypothesis was supported by the results from X-ray absorption fine structure (XAFS), UV-vis, and Raman spectroscopies. The computational model indicated that binuclear Fe sites exhibit exceptional reactivity for H-abstraction from CH4[92]. Additionally, recent studies revealed that mononuclear Fe is also highly active in the direct methane oxidation to CH3OH and HCOOH in aqueous solutions[93-96]. Table 1 summarizes the catalytic performance of reported Fe-containing zeolite catalysts for the selective oxidation of methane using hydrogen peroxide as an oxidant under mild reaction conditions, focusing on metal loading, preparation method, oxygenate yield, and TOF value. The reaction mechanisms for converting methane to CH3OH and HCOOH in H2O2 using Fe-containing zeolite catalysts typically begin with the formation of highly oxidized Fe species through the activation by H2O2, which then oxidizes CH4. However, there is a lack of in situ characterizations to directly analyze the structures of mono-/binuclear Fe species and their intermediates during catalysis. Current research on the reaction mechanisms often relies on DFT calculations.
Catalytic performance of reported Fe-containing zeolite catalysts for the selective oxidation of methane using hydrogen peroxide as oxidant under mild conditions
Catalysts | Metal loading (wt%) | Nuclearity | Synthesis method | Oxygenates yielda (mol·kgcat.-1·h-1) | TOFb (h-1) | Ref. |
Fe-ZSM-5 (WI) | Fe (0.54) | Mononuclear | WI | 2.8 | 28.6 | [80] |
Fe-ZSM-5 (HT) | Fe (0.59) | Mononuclear | In situ | 3.5 | 33.0 | [80] |
Fe-ZSM-5 (SIE) | Fe (0.50) | Mononuclear | SSIE | 6.8 | 58.2 | [80] |
Fe-ZSM-5 (IE) | Fe (0.43) | Mononuclear | IE | 17.1 | 223.0 | [80] |
Fe-ZSM-5 (84)550 | Fe (0.49) | Binuclear | In situ | 13.5 | 154.0 | [60] |
Fe/ZSM-5(23) | Fe (2.79) | Binuclear | SSIE | 8.0 | 16.1 | [97] |
Fe-HZ5-TF | Fe (0.40) | Mononuclear | In situ | 59.3 | 849.0 | [81] |
Ga,Fe-MFI(50) | Fe (1.63) | Extra-framework | In situ | 49.4 | 170.0 | [83] |
0.5%Fe/ZSM-5 | Fe (0.57) | Mononuclear | WI | 8.9 | 88.0 | [98] |
1%Fe/ZSM-5 | Fe (1.10) | Mononuclear | WI | 11.0 | 56.0 | [98] |
0.03wt%Fe/ZSM-5(66) | Fe (0.028) | Mononuclear | IE | 16.6 | 3,104.0 | [92] |
Fe3+/ZSM-5(30)COMM | Fe (2.50) | Mononuclear | SSIE | 16.7 | 37.5 | [95] |
Cu-Fe/ZSM-5 (30) | Fe (2.50) | Binuclear | SSIE | 14.0 | 31.3 | [77] |
Cu-Fe(2/0.1)/ZSM-5 | Fe (0.13) | Mononuclear | WI | 1.7 | 75.2 | [85] |
0.44Fe@Z-L0.3H6-9 | Fe (0.44) | Mononuclear | In situ | 41.3 | 526.0 | [82] |
Binuclear Fe species
Fang et al. identified the extra-framework binuclear [Fe2(μ-OH)2O2] as the active site for methane oxidation in the H2O2 solution over Fe/MOR[78]. The methanol formation mechanism involves a CH3OOH intermediate pathway: CH4 adsorbs and reacts with the terminal oxygen species in [Fe2(μ-OH)2O2] to form CH3O*. Then, CH3O* reacts with H2O2* to generate CH3OOH*. The extraction of one oxygen from
Figure 4. (A) Catalytic cycle for the liquid-phase oxidation of methane to methanol over the binuclear Fe3+ species in MOR. Reprinted with permission from ref[78]. Copyright 2019. the Royal Society of Chemistry; (B) [(H2O)2–Fe(III)–(μ-O)2–Fe(III)–(H2O)2]2+ model in ZSM5 zeolite. Reprinted with permission from ref[96]. Copyright 2018. American Chemical Society; [C(i)-(iii)] STEM images of the 0.1% Fe/ZSM-5. [C(iv)] Elemental mapping images of the 0.1% Fe/ZSM-5. Reprinted with permission from ref[98]. Copyright 2021. The Royal Society of Chemistry; Low-magnification [D(i)] TEM, [D(ii)] STEM, and Cs-corrected. [D(iii)] STEM-ADF and [D(iv)] STEM-ABF images of 0.44Fe@Z-L0.3H6-9. [D(v)-(viii)] Elemental mappings for Si, Al, O, and Fe elements; (E) Proposed reaction mechanisms and schematic illustrations for the selective oxidation of methane on the mononuclear Fe3+. Reprinted with permission from ref[82]. Copyright 2024. The Chinese Chemical Society. STEM: Scanning transmission electron microscopy; TEM: transmission electron microscopy; ADF: annular dark-field; ABF: annular bright-field.
Mononuclear Fe species
Yu et al. identified mononuclear Fe species as the active sites in Fe/ZSM-5 for methane oxidation[98]. The sample containing 0.1 wt% Fe (0.1% Fe/ZSM-5) primarily consists of mononuclear Fe with octahedral coordination. As seen from Figure 4C(i)-(iv), single-site Fe species are uniformly distributed across the zeolite crystal. In situ XAFS analysis provided evidence of the interaction between CH4 molecular and isolated Fe active centers. Moreover, in situ FTIR spectroscopy indicated a strong interaction between Fe3+ and CH4 molecular, which weakens the interaction between Brønsted acid sites and CH4 molecular. During the oxidation of methane, CH3OOH and CH3OH were first generated from CH4, with CH3OH further converted to hydroxymethyl hydroperoxide (HOCH2OOH) and subsequently to HCOOH. In addition, Cheng et al. synthesized Fe-ZSM-5 catalysts featuring mononuclear Fe (Fe-HZ5-TF)[81]. Electron paramagnetic resonance (EPR) spectroscopy and in situ DRIFTS results showed that initial mononuclear Fe(III) species are oxidized by H2O2, generating Fe(IV)=O species and hydroxyl (•OH) radicals. CH4 is consequently adsorbed on the Fe(IV)=O sites, followed by the homolytic cleavage of a C–H bond to generate Fe(III)–OH and methyl (•CH3) radicals. Subsequently, these •CH3 radicals combine with peroxyl
Gas phase methane conversion over Fe-containing zeolites
Fe-containing zeolite catalysts applied in methane oxidation in H2O2 aqueous solution have several shortcomings, such as over-oxidation of methanol, high oxidant costs, and low yields of oxygenates[99]. Due to these limitations in the aqueous-phase catalytic system, one promising option is the gas-phase oxidation of methane. Fe-containing zeolites have shown great potential in gas-phase methane oxidation using N2O or O2 as oxidants, offering a more efficient route for methane conversion[56,100].
N2O as oxidant
Catalytic conversion of CH4 with N2O to value-added chemicals can effectively reduce greenhouse gas emissions while addressing the growing demand for chemicals[101]. In Fe-containing zeolite catalysts, the mechanism for breaking C–H bonds in the Fe-N2O system typically involves two steps. Initially, N2O decomposes at the active Fe center (α-site or α-Fe), generating surface oxygen species (α-O), which can subsequently oxidize inert C–H bonds. Xiao et al. utilized the interzeolite conversion method to rapidly synthesize Fe-containing AEI zeolites with varying Si/Fe ratios in a one-pot process[102]. The reaction mechanism involves N2O dissociation at the active Fe site, producing highly reactive oxygen species (e.g., [Fe=O] or [Fe(μ-O)]2+) while releasing N2. These oxygen species activate the C–H bond of CH4 to generate methoxy intermediates, which convert to methanol upon interaction with water [Figure 5A]. Specifically, water can facilitate the regeneration of α-Fe active sites through redox interactions, thereby prolonging the catalyst’s lifespan and enabling catalytic cycling. Moreover, methane oxidation to methanol can be achieved by loading Fe species in small-pore CHA zeolite, either with or without sodium [Figure 5B and C][103]. Fe-containing zeolites show good selectivity and stability under N2O conditions, but excessive activation of oxygen species may lead to further oxidation of methanol.
Figure 5. (A) Possible reaction pathway of the direct oxidation of methane with N2O on the Fe-AEI zeolite catalyst. Reprinted with permission from ref[102]. Copyright 2023. American Chemical Society; (B) Comparing conversion and selectivity of H-type Fe-CHA(Na)-100 and Fe-CHA(Na free)-100 at 250 °C; (C) Direct oxidation of methane to methanol on active Fe sites over Fe-containing zeolites. Reprinted with permission from ref[103]. Copyright 2024. American Chemical Society; (D) Schematic representation of Fe(II) located in the β cationic position and the α cationic position; (E) Time dependence of the evolution of the bands characteristic for α-O and Fe(II) in β sites. Reprinted with permission from ref[56]. Copyright 2023. American Chemical Society.
O2 as oxidant
Fe-containing zeolite catalysts also demonstrated activity in the production of methanol from methane using O2 as the oxidant[104]. O2 is abundant, inexpensive, and environmentally friendly, making it preferable over other oxidants such as N2O and H2O2. Utilizing O2 streamlines the reaction process by eliminating the need for additional oxidant treatment steps. The unique structure of Fe-containing zeolites enhances O2 activation and stabilizes high-activity oxygen species, such as [Fe=O] and dioxygen bridge [Fe(μ-O)], facilitating methane oxidation. Mlekodaj et al. systematically investigated the spectral characteristics of the Fe(II) center in FER during an oxidation-reduction cycle at 220 °C using FTIR, Mossbauer, and X-ray absorption spectroscopy (XAS) techniques[56]. Their studies confirmed the dissociation of O2 on the cooperating Fe(II) sites [Figure 5D]. With the time reaching 60 min, all Fe(II) in β sites were oxidized and the highest intensity of the band typical for α-O was obtained [Figure 5E]. The interaction between α-O and methane at 220 °C produced methanol in three cycles. Notably, the stability of α-O at elevated temperatures promises the application of Fe-FER catalysts in methane oxidation upon a wide range of temperatures. Table 2 summarizes the catalytic performance of reported Fe- and Cu-containing zeolite catalysts in methane oxidation under the gas phase.
Catalytic performance of reported Fe- and Cu-containing zeolite catalysts for methane oxidation in gas phase
Catalysts | Oxidant | Type | Conditions | Performance | Ref. |
Fe/AEI-100 | N2O | Continuous | 100 mg catalyst, 250 °C, CH4/N2O/H2O/Ar = 10/10/2/3 (total: 25 mL/min) | CH3OH rate: 4.0 μmol·g-1·min-1 | [102] |
Fe/AEI-400 | N2O | Continuous | 100 mg catalyst, 350 °C, CH4/N2O/H2O/Ar = 10/10/2/3 (total: 25 mL/min) | CH3OH rate: 45.0 μmol·g-1·min-1 | [102] |
H–Cu-SSZ-13 | N2O | Step-wise | 300 °C, 30.4 kPa CH4, 30.4 kPa N2O, 3.2 kPa H2O, 37.3 kPa He, flow rate: 120 cm3·min-1, WHSV = 19,650 gfeed·gcat-1·h-1 | CH3OH rate: 55.0 μmol·g-1·min-1 | [105] |
Cu0.9-CHA13 | O2 | Continuous | 300 °C, CH4/O2/H2O/N2 = 48/2/10/50 mL·min-1 | CH3OH rate: 236.5 μmol·g-1·h-1 | [106] |
Fe-CHA | N2O | Step-wise | Activation:180 °C, 35% N2O/He Reaction: room temperature pure CH4 Extraction: water/acetonitrile mixture | CH3OH yield: 183 μmol·g-1 | [107] |
Cu-Na-MOR(0.28) | O2 | Step-wise | Activation: 250 °C, O2 Reaction: 150 °C under CH4 Extraction: water/acetonitrile mixture | CH3OH yield: 41.1 μmol·g-1 | [108] |
1.1%Cu-H-MOR (15) | O2 | Continuous | 400 °C, 33 mol% methane, 85 ppm O2, and 67 mol% steam | CH3OH rate: 143 ± 1.9 mmol·molCu-1·h-1 | [109] |
5Cu/AEI(Na)-850 | N2O | Continuous | 350 °C, N2O/CH4/H2O/Ar = 10/10/2/3 mL·min-1, WHSV = 15,000 mL·g-1·h-1 | CH3OH rate: 27.3 μmol·g-1·min-1 | [110] |
Cu(0.31)MFI(12) | O2 | Step-wise | Activation: 450 °C under O2 Reaction: 180 °C, 8 bar CH4 Extraction: 75 °C, wet Helium | CH3OH yield: 22.8 μmol·g-1 | [111] |
Cu/AEI | N2O | Continuous | 100 mg catalyst, 350 °C, CH4/N2O/H2O/Ar = 10/10/2/3 (total: 25 mL/min) | CH3OH rate: 41.0 μmol·g-1·min-1 | [112] |
Cu-MOR | O2 | Step-wise | Activation: 450 °C under O2 Reaction: 200 °C, 1 bar CH4 Extraction: 200 °C, H2O | CH3OH yield: 155.0 μmol·g-1 | [113] |
Cu-containing zeolite catalysts
Cu-containing zeolites have demonstrated the ability to convert methane to methanol with high selectivity using O2 or N2O as oxidants. Groothaert et al. first reported that O2-activated Cu-containing MFI and MOR zeolites exhibit activity for methane oxidation to methanol[52]. Subsequently, it was discovered that Cu-containing CHA zeolite also works on methane oxidation to methanol[105,106,114]. Two distinct processes for the methane conversion to methanol over Cu-containing zeolite catalysts have been proposed: a stepwise method involving successive activation-reaction cycles (referred to as “chemical looping”)[54,115,116] and a catalytic system utilizing continuous gas flow with either O2[117,118] or N2O[105] as the oxidant. As the synthesis methods of Cu-containing zeolite are similar to those of Fe-containing zeolite catalysts, this section focuses on the active metallic sites and reaction mechanisms, together with influences of zeolite descriptors on the catalytic performance.
Modulating zeolite descriptors to promote methane oxidation
Cu/Al and Si/Al ratios
Modulating the Cu/Al ratio is effective in promoting the catalytic performance of Cu-containing zeolite for methane oxidation[108,109]. An optimal Cu/Al ratio strikes a balance between methane conversion rate and methanol selectivity. Dinh et al. asserted that low copper loading (Cu/cage < 0.3) combined with high aluminum content (Si/Al > 30) is essential for facilitating methane oxidation by the enhanced amount of [Cu–O–Cu]2+ species[117]. This approach mitigates the formation of other copper structures and CuxOy nanoclusters that favor the over-oxidation of methane to CO2. Similar to Fe-containing zeolite catalysts, the reactivity of Cu-containing zeolite for methane oxidation is influenced by the Si/Al molar ratio and zeolite topology. Research on methane conversion over Fe-containing zeolite has demonstrated that iron-based α-oxygen active sites can be stabilized at the IE sites that derive from framework Al atoms[97]. This stabilization principle applies equally to Cu-containing zeolites[110,119,120]. Artsiusheuski et al. provided a clear understanding of the impact of the Cu/Al and Si/Al ratios on the catalytic performance[111]. They found that, when the Cu/Al ratio was below 0.3, binuclear Cu species with a Cu–Cu of 2.9 Å dipoles and a UV-vis absorption band at 27,200 cm-1 were observed in Cu-containing MFI zeolites. This configuration facilitates the selective methane oxidation to methanol at 450-550 K. In contrast, a higher Cu/Al ratio results in mono-μ-oxo dicopper sites (Cu–Cu = 3.2 Å), which display a characteristic band at 21,900 cm-1 and react with methane at lower temperatures (< 450 K) to produce over-oxidized products [Figure 6A].
Figure 6. (A) Characteristics of mono-μ-oxo dimeric copper sites formed in oxygen-activated Cu-MFI materials at different Cu/Al ratios. Reprinted with permission from ref[111]. Copyright 2022. American Chemical Society; (B) Structures of different copper sites hosted in zeolites with different topologies and their evolution during the reaction with methane. Reprinted with permission from ref[121]. Copyright 2023. American Chemical Society.
Pore window size of zeolites
The variations in size and shape of zeolite pore structures significantly influence the distribution, oxidation state, and stability of active Cu sites, thereby determining their reactivity and selectivity in chemical reactions. Calculations of dimer exchange probabilities across all unique 2Al configurations within various zeolite topologies indicated that binuclear Cu species are preferentially formed at the sites with an Al–Al distance of 5-9 Å[122-124]. This requirement fosters the formation of dimers in 8- and 10-membered rings (8- and 10-MR) across various zeolite topologies. In contrast, 6-membered rings (6-MR) favor monomer formation, while 4- and 5-membered rings (4- and 5-MR) are generally less favorable for copper exchange due to steric repulsions. Wijerathne et al. recently employed spectroscopic characterization, DFT calculations, and machine learning classification models to elucidate the influence of zeolite topologies on copper active sites[125]. Their study found that MFI-type zeolites, characterized by medium pores and intersecting channels, preferentially form binuclear copper sites. In comparison, CHA and AEI zeolites with small pores are more effective for stabilizing mononuclear copper sites. Furthermore, by employing machine learning models to analyze the formation preferences of mononuclear versus binuclear Cu sites across 200 zeolite types from the International Zeolite Database under varying aluminum configurations, the relationship between the metal nuclearity and zeolite topologies was established, which is of great significance to prepare efficient metal-zeolite catalysts by design. The study revealed that MOR zeolite exhibits a higher proportion of binuclear Cu species than CHA, AFX, *BEA, and FER for two primary reasons. Firstly, MOR possesses more 2Al configurations that favor binuclear Cu exchange. Secondly, commercial MOR zeolite decreases the availability of 2Al sites for monomer exchange while increasing the number of 2Al sites that contribute to lowering binuclear Cu exchange free energies. Figure 6B illustrates the structures of Cu species in various zeolite topologies and their evolution during methane conversion[121]. For Cu-containing MFI, mono-μ-oxo binuclear Cu(II) sites are the predominant species, whereas Cu-containing MOR exhibits a coexistence of mononuclear and binuclear Cu(II) sites, and Cu-containing FAU hosts Cu(II)-oxo clusters. Both mononuclear and binuclear Cu(II) sites transformed to isolated Cu(I) sites, while the Cu(II)-oxo clusters undergo restructuring during the reaction, resulting in the formation of Cu(I)-oxo clusters.
Al pairs in Cu-containing zeolites
Binuclear Cu species has been regarded as a highly active species, which is closely linked to the aluminum pairs (2Al) within the zeolite framework[126]. In Al-rich zeolites, framework Al atoms predominantly exist in Al–Si–Si–Al and Al–Si–Al sequences, forming Al pairs. These Al pairs provide two adjacent negative charges to stabilize active Cu species, thereby enhancing their reactivity and overall catalytic performance. For instance, using interzeolite conversion method, Xiao et al. synthesized AEI-type zeolite with an enhanced amount of Al pairs[112]. These Al pairs facilitated the formation of abundant binuclear Cu sites in Cu-containing AEI zeolite and therefore enhanced the methane selective oxidation efficiency. This resulted in a methanol productivity of 40.8 μmol·g-1·min-1, a methanol selectivity of 45%, and long-term stability in reactions at 350 °C using N2O as an oxidant.
Active sites in Cu-containing zeolites
It has been widely accepted that multinuclear Cu species are active sites in pMMO[106,127]. However, the precise structure of Cu species in pMMO remains debatable[128,129]. The design of Cu-containing zeolite catalysts for methane conversion processes is inspired by these naturally occurring enzymes. UV-vis spectroscopy is generally employed to analyze the configurations of active sites in Cu-containing zeolites, as the UV-vis spectrum varies with the copper composition[130-133]. The proposed active Cu sites in zeolites primarily involve mono-, bi- and trinuclear Cu species, which will be discussed in this section.
Binuclear Cu species
The high activity of Cu/ZSM-5 zeolite catalyst for CH4 oxidation to CH3OH has been demonstrated, which is ascribed to the extra-framework binuclear Cu species in zeolites[134]. Initially, a bis(μ-oxo) binuclear Cu(II) complex was proposed, denoted as [Cu(μ-O)2Cu]2+, with a UV-vis absorption peak at 22,700 cm-1. Woertink et al. highlighted the significance of a (μ-oxo) binuclear Cu(II) complex, namely [Cu(μ-O)Cu]2+ sites[75]. The resonance Raman (rR) vibrations at 237, 456, and 870 cm-1 were assigned to the Cu–O–Cu bending mode of the mono(μ-oxygen bridge) binuclear Cu site (Cu–O–Cu bond angle of 140°), the symmetric Cu-oxygen stretching vibration (νs), and the asymmetric Cu-oxygen stretching vibration (νas), respectively. Recently, utilizing the variable-field magnetic circular dichroism (VTVH MCD) technology, Heyer et al. effectively explored the magnetism of these active sites in the mixtures, thereby minimizing interference from other Cu species[135]. They characterized the magnetic exchange coupling of the [Cu2O]2+ species within CHA and MFI and demonstrated that in binuclear Cu sites, the ground-state magnetism is primarily determined by the relative orientation of the bidentate aluminosilicate zeolite linking the two Cu atoms, rather than the Cu–O–Cu angle [Figure 7A]. Plessers et al. identified a new active species for direct methane oxidation to methanol, termed MOR3, in high copper-loaded Cu-MOR[108]. Characterized by the UV-vis DR absorption and rR spectroscopy, the MOR3 was identified as a [CuOCu]2+ species with a Cu–O–Cu bond angle of 127°. The location of MOR3 sites in Cu-K-MOR (0.25) was probed using the bulky substrate tetrahydrofuran which can only react with Cu species exposed to the 12-membered ring (12-MR) channels of MOR. The results indicated that MOR3 is located inside the 8-MR side pocket or the compressed 8-MR channel. Such MOR3 species showed higher activity than previously characterized [CuOH]+ site, as observed in other [CuOCu]2+ sites (a Cu–O–Cu bond angle of 137° and 141°).
Figure 7. (A) DFT models for Cu-MFI Site 7 (left) and Cu-CHA (right). The orbital contours for the α LUMO and β LUMO for the BS singlet are given for each model along with the calculated J and orbital overlap term (Color scheme: red = O, pink = Al, light gray = Si, white = hydrogen, and mustard = Cu). Reprinted with permission from ref[135]. Copyright 2024. American Chemical Society; (B) Spin density distributions for bi- and trinuclear Cu-oxo complexes and the respective possible resonance structures that could be proposed to describe the formal charge configuration in the extra-framework species. Reprinted with permission from ref[136]. Copyright 2016. Elsevier; (C) Composition of species in Cu-MOR under various conditions. Reprinted with permission from ref[6]. Copyright 2022. American Chemical Society. DFT: Density functional theory; LUMO: lowest unoccupied molecular orbital; BS: broken symmetry.
Trinuclear Cu species
Recent research highlighted the significance of trinuclear Cu species in methane conversion[137]. It has been revealed that such a motif promotes oxygen transfer during alkane hydroxylation and enables regeneration of the active site in the presence of O2. Grundner et al. reported the successful modification of H-MOR zeolites with copper (Cu/MOR) featuring uniform extra-framework trinuclear species, which mimic the active metal species in enzyme[138]. In situ XAS results demonstrated the structure of trinuclear Cu-oxo species in activated Cu-MOR, namely [Cu3(μ–O)3]2+, stabilized by two framework aluminum atoms at the mouth of the 8-MR side pockets. The [Cu3(μ–O)3]2+ showed remarkable stability under dry conditions, consistent with ab initio thermodynamic analyses derived from DFT results. Although methanol extraction by steam treatment leads to the hydrolysis of the cluster, it can be reconstituted without a loss of activity upon reactivation in O2. Li et al. studied the structure of active sites and reaction mechanisms in Cu-containing ZSM-5 zeolite[136]. The periodic DFT calculations and thermodynamic analyses indicated that, at relatively low O2 partial pressures, the formation of binuclear [Cu(μ–O)Cu]2+ site is favored [Figure 7B], while the trinuclear Cu-oxygen species emerges as the most thermodynamically stable sites in the Cu/ZSM-5 activated at high temperatures in an O2-rich environment. Both structures can activate methane; however, only the trinuclear complex provides a favorable energy reaction pathway for methanol production, following a direct free radical rebound mechanism.
Mononuclear Cu species
The mononuclear [CuOH]+ active sites in zeolites provide complementary pathways for methane oxidation, alongside the established [Cu–O–Cu]2+ and Cu3O3 structures[139]. Kulkarni et al. prepared low aluminum-content CHA zeolite catalysts as a model system through copper ion exchange and systematically examined the methane partial oxidation reaction in the Cu-containing zeolite using DFT calculations[140]. Their findings indicated that the [CuOH]+ located in the 8-MR serves as the active species, whereas 6-MR structures are detrimental to the catalytic reaction. The 8-MR is desired for increasing the amount of [CuOH]+ species. Sushkevich et al. revealed that, in Cu-containing MOR, besides the known mono-μ-oxo binuclear copper sites, the mononuclear [CuOH]+ site was identified as an active species[141]. Both sites can react with methane at moderate temperatures to produce methoxy, methanol, and several over-oxidation products (e.g., formate and carbon monoxide). They proposed that reactions at [CuOH]+ sites require lower activation energy but proceed more slowly than those at the mono-μ-oxo binuclear copper species within the relevant temperature range. Furthermore, Heyer et al. employed a range of characterization techniques, including DR UV-vis spectroscopy and magnetic circular dichroism, to definitively identify the mononuclear [CuOH]+ as the active site in the self-reduced Cu-MOR zeolite catalyst for methane oxidation[6]. They proposed the structure of active species evolution in zeolite under different conditions [Figure 7C]. Kinetic experiments comparing the reactivities of the [CuOH]+ and [Cu2O]2+ sites revealed that the binuclear species exhibit higher reactivity, which is due to the stabilization of the [Cu2OH]2+ H-atom abstraction product over the two Cu cations.
Reaction mechanisms over Cu-containing zeolite
O2 as oxidant
In Cu-containing zeolite catalysts, the methane oxidation mechanism using O2 as the oxidant involves the activation of the copper sites with O2 to form highly reactive Cu–O species or double oxide-bridged binuclear copper species (e.g., [Cu2(μ-O)]2+). These active species interact with methane at moderate temperatures, activating the C–H bond and generating methoxy intermediates. Subsequently, these generated methoxy intermediates are hydrolyzed to produce methanol. Throughout the reaction, the active copper sites are continuously regenerated by oxidation, maintaining the catalytic cycle. Due to the strong oxidizing ability of O2, a key challenge lies in controlling the activity of oxygen species to prevent excessive methanol oxidation. Dinh et al. elucidated the reaction pathway and active sites for CH4 conversion to methanol on Cu-SSZ-13 zeolite through dynamic experiments and spectroscopic techniques[117]. CH4 activation entails critical cleavage of C–H bonds on the surface to generate a surface-bound C1 intermediate, which can desorb to form methanol in the presence of H2O/H+ or be fully oxidized to CO2 in the presence of O2. The [Cu–O–Cu]2+ structure is thought to be formed through the diffusion of hydrated copper ions along a proton-assisted pathway [Figure 8A].
Figure 8. (A) Depiction of hypothesized H+/H2O-aided diffusion of Cu+ and NH3 inhibition within SSZ-13 to form Cu dimers relevant to the proposed catalytic methane oxidation cycle. Single O atoms may correspond to framework zeolite O atoms or coordinating H2O molecules. Reprinted with permission from ref[117]. Copyright 2019. American Chemical Society; (B) The possible reaction pathway of the direct conversion of methane with N2O on the Cu/AEI zeolite catalyst. Reprinted with permission from ref[112]. Copyright 2023. American Chemical Society.
N2O as oxidant
The methane oxidation mechanism over Cu-containing zeolite catalyst using N2O as an oxidant begins with the dissociation of N2O at the copper site, generating active oxygen species (e.g., Cu–O or [Cu2O]2+) and releasing N2[73]. These activated oxygen species promote C–H bond cleavage in methane, generating methoxy intermediates. These intermediates can further react with water to produce methanol, while the copper site regenerates its activity through reaction with N2O. The binuclear copper site demonstrated superior efficiency and selectivity for methane oxidation [Figure 8B][112].
Other catalysts
Non-noble metal in zeolites
Besides Fe and Cu species, other transition metals have also attracted significant interest from researchers. For instance, cobalt (Co) and nickel (Ni) are anticipated to exhibit methane partial oxidation activities comparable to Fe[139]. Mahyuddin et al. conducted theoretical studies on MO+-ZSM-5 zeolites (M = Fe, Co, Ni, Cu) using DFT to investigate the influence of various metals on methane oxidation reactions [Figure 9A and B][142]. Their calculations revealed the following predicted activity order for the cleavage of the C–H bond of methane: CoO+-ZSM-5 < NiO+-ZSM-5 < FeO+-ZSM-5 < CuO+-ZSM-5. In contrast, the predicted methanol selectivity follows the order of FeO+-ZSM-5 < CoO+-ZSM-5 < NiO+-ZSM-5 < CuO+- ZSM-5. In Co-containing ZSM-5 zeolites, at least two distinct Co species may be present: Co2+ ions located at IE sites and Co oxide species, each with differing catalytic and magnetic properties[143]. Beznis et al. found that Co-containing ZSM-5 prepared through ion exchange with cobalt acetate primarily contains highly dispersed Co species within zeolite channels, demonstrating selectivity towards formaldehyde at 150°C in O2[57]. In contrast, impregnated Co-containing ZSM-5 samples that mainly contain cobalt oxide species on zeolite particle surfaces showed higher selectivity towards methanol. Shan et al. anchored a bent mono(μ-oxo)dinickel complex within ZSM-5 micropores using a WI method[58]. The yield of CH3OH, HCOOH, and HOCH2CH2OH over ZSM-5 catalyst at 175 °C in 1 bar of CH4 is 14.9 μmol per gram. In another work, theoretical studies proposed an alternative candidate for the active site, a bis(μ-O) bimetallic nickel structure [Figure 9C][144,145]. In 2012, Xu et al. reported that Zn-loaded H-ZSM-5 zeolite exhibits activity for methane activation[59]. Isolated Zn2+ ion facilitated the heterolytic cleavage of the C–H bond and led to the formation of zinc methyl species, while the dizinc cluster preferentially generated surface methoxy species through the homolytic cleavage of a C–H bond. Recently, Xiao et al. demonstrated that FER zeolite exhibited high reactivity in converting methane to methanol in the presence of nitrous oxide, in which a high methanol rate of 305 μmol·g-1·min-1 and a high methanol selectivity of 89% were steadily achieved[146]. As indicated by
Figure 9. (A) Possible catalytic cycle for the methane to methanol conversion by MO+-ZSM-5; (B) Optimized structures of CoO+-and NiO+-ZSM-5 in the corresponding high-spin state. Reprinted with permission from ref[142]. Copyright 2016. American Chemical Society; (C) Probable scenarios of the reaction pathway for methane overoxidation to formaldehyde (CH2O) and dimethyl ether (C2H6O) over Ni-ZSM-5 zeolites. Reprinted with permission from ref[144]. Copyright 2021. American Chemical Society; (D) 27Al MAS NMR spectra of FER zeolites after dealumination and supplement Al by impregnation; Positions of four crystallographically independent T sites and the two perpendicular intersecting channels accessible via (E) 001 and (F) 010 faces. Reprinted with permission from ref[146]. Copyright 2024. American Chemical Society. 27Al MAS NMR: 27Al magic-angle spinning nuclear magnetic resonance.
Noble metal in zeolites
Noble metal atoms[39,147-150], clusters[151], and nanoparticles[152-156] also work for C–H activation. AuPd-based catalysts are particularly effective for the catalytic synthesis of H2O2 using O2 and H2, which readily decomposes into highly active hydroperoxyl and hydroxyl species[157-161]. These species participate in various selective oxidation reactions, providing a robust strategy for enhancing catalytic activity and selectivity toward oxygenates compared to O2 as the oxidant[162,163]. A notable study by Jin et al. involved embedding AuPd alloy nanoparticles within ZSM-5 zeolite and subsequently coating the external surface of zeolite with a hydrophobic organosilane to impart hydrophobicity (AuPd@ZSM-5-C16)[31] [Figure 10A-C]. This hydrophobic shell prevented the diffusion of hydrophilic H2O2, formed from hydrogen and oxygen, away from the interior AuPd nanoparticles. It increases H2O2 concentration within the zeolite crystals. Meanwhile, hydrophobic methane molecules effectively interact with the AuPd nanoparticles in concentrated hydrogen peroxide due to the hydrophobic shell. The resulting AuPd@ZSM-5-C16 demonstrated a 17.3% methane conversion rate, 92% methanol selectivity, and 91.6 millimoles of methanol per gram of AuPd per hour [Figure 10D].
Figure 10. (A-C) Models and tomographic section TEM images of AuPd@ZSM-5-C16; (D) Data characterizing the oxidation of methane with H2 and O2 over various catalysts. Reprinted with permission from ref[31]. Copyright 2020. the American Association for the Advancement of Science; (E) Scheme of proposed reaction pathway for selective oxidation of CH4 with O2 and H2 over PdCu/Z-5. Reprinted with permission from ref[165]. Copyright 2022. Wiley-VCH GmbH. TEM: Transmission electron microscopy.
Recent studies have shown that methane oxidation can occur solely in the presence of oxygen[164]. Qi et al. demonstrated this using Au nanoparticles supported on ZSM-5 zeolite to convert CH4 to CH3OH and
CATALYTIC COUPLING OXIDATION OF CH4 WITH CO OVER METAL-ZEOLITE CATALYSTS
CO is a common carbonylation substrate that shows promise in coupling oxidation reactions with methane, providing a new strategy for low-temperature methane conversion to C2 oxygenated products, namely acetic acid[166]. Metal-zeolite catalysts, such as Rh-ZSM-5[167], Cu-ZSM-5[168] and Zn-ZSM-5[169], have demonstrated potential due to their favorable pore structures, acidic properties, and synergistic effects between metallic species and zeolite frameworks. These metal-zeolite catalysts provide a mild and efficient pathway for coupling methane with CO, effectively activating methane to generate methyl and methoxy active intermediates. This section will introduce recent research advancements in metal-zeolite catalysts for the coupling reaction of methane with CO.
Previous studies utilizing metal-zeolite catalysts for methane and CO coupling have primarily focused on acetic acid formation via the carbonylation of methanol, following the conventional Koch carbonylation mechanism[170]. In this process, methane undergoes partial oxidation to yield methanol, which subsequently reacts with CO on the catalyst surface to produce acetic acid (Pathway 1, Figure 11). However, this method necessitates high temperatures, elevated pressures, and acidic conditions, leading to substantial energy consumption and rapid catalyst deactivation[171]. These challenges limit its scalability and industrial applicability. Recent advancements have introduced the “methane carbonylation” concept, where methane is directly activated through oxidation-reduction or chemical looping processes[168]. This method generates active intermediates, such as methyl and methoxy species, which then couple with CO to form acetic acid and other oxygenated compounds under mild conditions (Pathway 2, Figure 11). This innovative pathway not only achieves high selectivity and efficiency, but also mitigates previous limitations by facilitating target conversions at significantly lower temperatures and pressures, presenting a promising route for the direct valorization of methane into high-value oxygenates.
Monometallic species in zeolites
Moteki et al. discovered that transition metals (Fe, Co, Ni, Cu) and platinum group metals (Ru, Rh, Pd, Ir, Pt) loaded on ZSM-5 effectively catalyze the coupling oxidation of CO and CH4[172]. Recently, Wang et al. found that the Fe/ZSM-5 (0.25) zeolite catalyst, featuring mononuclear Fe3+ species located in the 5-MR units, exhibited high activity and selectivity for CH4 coupling oxidation with CO using H2O2 as oxidant at
Figure 12. (A) The optimized catalytic sites, Rh1O5 anchored on Brønsted site in microspore of ZSM-5, and intermediates and transition states for a complete catalytic cycle, starting with Rh1O5. Reprinted with permission from ref[174]. Copyright 2018. Springer Nature; (B) Schematic illustration on the promotive effect of carbon additives on the methane oxidation catalyzed by Au/ZSM-5. Reprinted with permission from ref[175]. Copyright 2023. the American Chemical Society.
Besides CO gas, carbonyl groups on carbon additives can also participate in the oxidation of CH4[175]. The incorporation of carbon additives into an Au/ZSM-5 catalyst significantly enhanced the performance of methane oxidation using O2 as the oxidant, yielding both methanol and acetic acid as products together with CO2. Notably, 13C labeling studies attributed the production of acetic acid to the carbon additive. X-ray photoelectron spectroscopy (XPS) results showed that the surface of the carbon additives was oxidized to
Bimetallic species in zeolites
The bimetallic catalyst design also provides a novel approach for the carbonylation of methane. Wu et al. demonstrated that Au-Fe/ZSM-5 catalysts achieve an acetic acid yield of 5.7 mmol·gcat-1 with a selectivity of 92% at 120 °C after three hours using O2 as the oxidant[176]. In situ DRIFTS results indicated that CO facilitates the dissociation of H2O to form Au–H and Au–COOH species. The presence of O2 promotes the reaction of Au–H species with O2 to form *OOH, which subsequently converts to *OH. In the presence of CO (with H2O2 as the oxidant), Fe/ZSM-5 exhibited a higher yield (2.5 mmol·gcat-1) of liquid products compared to Au/ZSM-5 and Au-Fe/ZSM-5 (both yielding 0.81 mmol·gcat-1) and Fe-free ZSM-5 (yielding 0). Therefore, dispersed iron species on ZSM-5 are crucial for the hydroxyl-mediated coupling of CH4 and CO to form acetic acid. Cheng et al. reported an efficient 0.1Cu/Fe-HZSM-5-TF catalyst synthesized via a template-free method, which features mononuclear Fe and Cu species within ZSM-5[166]. This catalyst achieved a high acetic acid yield of 40.5 mmol·gcat-1·h-1 at 50 °C, which is three times the previous record of 12.0 mmol·gcat-1·h-1. The combination of various characterizations, isotope labeling experiments, and DFT calculations indicated that mononuclear Fe species are responsible for methane conversion, while adjacent Cu species play a critical role in decelerating the oxidation process, thus enhancing the synthesis of acetic acid product. Furthermore, the methyl carbon of acetic acid was derived entirely from methane, and the carbonyl carbon was sourced solely from CO.
CONCLUSION AND OUTLOOK
In summary, metal-zeolite catalysts have demonstrated a pivotal role in the low-temperature oxidation of methane. This effectiveness stems from the unique features of zeolites, including their regular microporous structure, high specific surface area, adjustable Si/Al ratio, large IE capacity, confinement effect, and robust thermal and hydrothermal stabilities. This review summarizes recent advancements of metal-zeolite catalyst processing low-temperature methane oxidation to oxygenates, providing deeper insights into the synthesis methods, reaction mechanisms, metallic active sites, and catalytic performance. Currently, the low-temperature oxidation of methane using metal-zeolite catalysts still faces several challenges yet accompanied by opportunities. Future research directions in the field of low-temperature methane oxidation could focus on the following points.
Developing high activity and selectivity metal-zeolite catalysts
While metal-zeolite catalysts promote the low-temperature oxidation of methane to oxygenates with high TOF value and product selectivity, the low-temperature methane oxidation still suffers from low methane conversion efficiency (generally below 5%) and oxygenate productivity. This limits the commercial viability of low-temperature methane conversion processes. It is crucial to explore novel high-performance catalysts or develop new catalytic systems to facilitate the industrial application of metal-zeolite for low-temperature conversion. Based on existing mature metal-zeolite systems, fine tailoring zeolite framework, type of extra-framework metal species, metal dispersion, coordination environment of metallic species, and proximity between active sites are desirable to achieve enhanced catalytic performance. Moreover, to date, only several types of zeolites have been utilized as matrices for low-temperature methane oxidation, such as MFI, CHA, AEI, FER, and MOR. Other types of zeolite topologies with varied nanoconfinements should be employed in this field.
Breaking the loading amount limit of highly active metal species
Increasing the loading amount of highly active metallic sites, such as mono-, bi- and trinuclear metal species, is a straightforward method to achieve a substantially enhanced catalytic performance. For instance, the maximum Fe loading of Fe-containing zeolites that primarily contain mononuclear Fe species is approximately 0.5 wt%. With increasing metal loading, large metal clusters/particles are generally formed inside/outside zeolite crystals. Up till now, the synthesis of metal-zeolite catalysts with high metal loading and good metal dispersion remains a big challenge. From both academic and industrial perspectives, it is of great importance to develop novel synthetic approaches to break the loading amount limit of mono-, bi- and trinuclear metallic species. This will be an escalating significant research direction for the synthesis of metal-zeolite catalysts.
Green catalytic system over metal-zeolite catalysts
H2O2 and N2O are frequently used as oxidants in the low-temperature oxidation of methane; however, they present several limitations, such as high costs, stringent storage requirements, etc. Using O2 as an oxidant for continuous methane conversion is highly desired but remains a big challenge due to the poor productivity, selectivity, and security issue with mixing O2 and combustible gas. Two approaches could be considered to achieve a green catalytic system in the future: (i) Developing highly active metal-zeolite catalysts that can be efficiently activated by using H2O and CO2 as weak oxidants; (ii) Constructing a photo-assisted thermocatalytic system in which the sunlight promotes the activation of methane.
Mechanism investigation and identification of active metal sites via operando or in situ characterization methods
In-depth understanding of the structural evolution and dynamics of metal sites during reaction and the reaction mechanisms of low-temperature methane oxidation is important to accelerate the rational design of metal-zeolite catalysts and optimize the reaction conditions. Current research on reaction mechanisms and active sites for low-temperature methane oxidation relies on DFT calculations. Operando or in situ spectroscopic and microscopic techniques, such as XAS, Mössbauer spectroscopy, and aberration-corrected STEM, are expected to be feasible to unravel the reaction mechanism, the structure of real active sites, and structural evolution of active sites in zeolites under catalytic conditions.
Screening and developing of high-performance metal-zeolite catalysts via artificial intelligence technology
Currently, the exploration of novel metal-zeolite catalysts strongly relies on labor-intensive work. The prediction of the synthesis–structure–property relationships of the metal-zeolite system based on available data in previously reported work is crucial to promote the discovery of new catalytic systems and efficient metal-zeolite catalysts. The rapid advancement of artificial intelligence (AI) technology offers exciting prospects for catalyst design. Future research can leverage AI technology to predict new types of metal active sites, suitable catalytic conditions, and possible reaction pathways for direct methane conversion.
In conclusion, with the development of synthesis methods, powerful characterization techniques, and AI, metal-zeolite catalysts are expected to achieve more success and bring more new opportunities for efficient methane utilization under mild conditions.
DECLARATIONS
Authors’ contributions
Wrote the draft manuscript: Xiang, B.; Li, J.; Chang, B.
Initiated, supervised, and revised the manuscript: Zhang, Q.
Availability of data and materials
Not applicable.
Financial support and sponsorship
The authors thank the National Key Research and Development Program of China (Grant 2022YFA1503600) and the National Natural Science Foundation of China (Grant 22101098) for supporting this work.
Conflicts of interest
All authors declared that there are no conflicts of interest.
Ethical approval and consent to participate
Not applicable.
Consent for publication
Not applicable.
Copyright
© The Author(s) 2025.
REFERENCES
1. Liu, B.; Oh, S. C.; Chen, H.; Liu, D. The effect of oxidation of ethane to oxygenates on Pt- and Zn-containing LTA zeolites with tunable selectivity. J. Energy. Chem. 2019, 30, 42-8.
2. Armstrong, R.; Hutchings, G.; Taylor, S. An overview of recent advances of the catalytic selective oxidation of ethane to oxygenates. Catalysts 2016, 6, 71.
4. Golisz, S. R.; Brent, G. T.; Goddard, W. A.; Groves, J. T.; Periana, R. A. Chemistry in the center for catalytic hydrocarbon functionalization: an energy frontier research center. Catal. Lett. 2011, 141, 213-21.
5. Dinh, K. T.; Sullivan, M. M.; Serna, P.; Meyer, R. J.; Dincă, M.; Román-Leshkov, Y. Viewpoint on the partial oxidation of methane to methanol using Cu- and Fe-exchanged zeolites. ACS. Catal. 2018, 8, 8306-13.
6. Heyer, A. J.; Plessers, D.; Braun, A.; et al. Methane activation by a mononuclear copper active site in the zeolite mordenite: effect of metal nuclearity on reactivity. J. Am. Chem. Soc. 2022, 144, 19305-16.
7. Forde, M. M.; Armstrong, R. D.; Hammond, C.; et al. Partial oxidation of ethane to oxygenates using Fe- and Cu-containing ZSM-5. J. Am. Chem. Soc. 2013, 135, 11087-99.
8. Wang, C.; Zhang, J.; Qin, G.; et al. Direct conversion of syngas to ethanol within zeolite crystals. Chem 2020, 6, 646-57.
9. Wang, C.; Huang, Y.; Wang, L.; Xiao, F. Structure-performance interplay of rhodium-based catalysts for syngas conversion to ethanol. Mater. Chem. Front. 2022, 6, 663-79.
10. Pakhare, D.; Spivey, J. A review of dry (CO2) reforming of methane over noble metal catalysts. Chem. Soc. Rev. 2014, 43, 7813-37.
11. Movasati, A.; Alavi, S. M.; Mazloom, G. Dry reforming of methane over CeO2-ZnAl2O4 supported Ni and Ni-Co nano-catalysts. Fuel 2019, 236, 1254-62.
12. Zhang, Q.; Yu, J.; Corma, A. Applications of zeolites to C1 chemistry: recent advances, challenges, and opportunities. Adv. Mater. 2020, 32, e2002927.
13. Taylor, S. H.; Hargreaves, J. S.; Hutchings, G. J.; Joyner, R. W.; Lembacher, C. W. The partial oxidation of methane to methanol: an approach to catalyst design. Catal. Today. 1998, 42, 217-24.
14. Ito, T.; Lunsford, J. H. Synthesis of ethylene and ethane by partial oxidation of methane over lithium-doped magnesium oxide. Nature 1985, 314, 721-2.
15. Periana, R. A.; Taube, D. J.; Evitt, E. R.; et al. A mercury-catalyzed, high-yield system for the oxidation of methane to methanol. Science 1993, 259, 340-3.
16. Periana, R. A.; Taube, D. J.; Gamble, S.; Taube, H.; Satoh, T.; Fujii, H. Platinum catalysts for the high-yield oxidation of methane to a methanol derivative. Science 1998, 280, 560-4.
17. Mironov, O. A.; Bischof, S. M.; Konnick, M. M.; et al. Using reduced catalysts for oxidation reactions: mechanistic studies of the “Periana-Catalytica” system for CH4 oxidation. J. Am. Chem. Soc. 2013, 135, 14644-58.
18. Baek, J.; Rungtaweevoranit, B.; Pei, X.; et al. Bioinspired metal-organic framework catalysts for selective methane oxidation to methanol. J. Am. Chem. Soc. 2018, 140, 18208-16.
19. Wang, Q.; Astruc, D. State of the art and prospects in metal-organic framework (MOF)-based and MOF-derived nanocatalysis. Chem. Rev. 2020, 120, 1438-511.
20. Ravi, M.; Ranocchiari, M.; van, B. J. A. The direct catalytic oxidation of methane to methanol-A critical assessment. Angew. Chem. Int. Ed. Engl. 2017, 56, 16464-83.
21. Sushkevich, V. L.; Palagin, D.; Ranocchiari, M.; van, B. J. A. Selective anaerobic oxidation of methane enables direct synthesis of methanol. Science 2017, 356, 523-7.
22. Olivos-Suarez, A. I.; Szécsényi, À.; Hensen, E. J. M.; Ruiz-Martinez, J.; Pidko, E. A.; Gascon, J. Strategies for the direct catalytic valorization of methane using heterogeneous catalysis: challenges and opportunities. ACS. Catal. 2016, 6, 2965-81.
23. Wu, L.; Fan, W.; Wang, X.; et al. Methane oxidation over the zeolites-based catalysts. Catalysts 2023, 13, 604.
24. Database of zeolite structures. http://www.iza-structure.org/databases/ (accessed 2025-02-10).
25. Zhang, Q.; Gao, S.; Yu, J. Metal sites in zeolites: synthesis, characterization, and catalysis. Chem. Rev. 2023, 123, 6039-106.
26. Li, Y.; Yu, J. New stories of zeolite structures: their descriptions, determinations, predictions, and evaluations. Chem. Rev. 2014, 114, 7268-316.
27. Zhang, Q.; Mayoral, A.; Terasaki, O.; et al. Amino acid-assisted construction of single-crystalline hierarchical nanozeolites via oriented-aggregation and intraparticle ripening. J. Am. Chem. Soc. 2019, 141, 3772-6.
28. Del Campo, P.; Martínez, C.; Corma, A. Activation and conversion of alkanes in the confined space of zeolite-type materials. Chem. Soc. Rev. 2021, 50, 8511-95.
29. Qi, G.; Davies, T. E.; Nasrallah, A.; et al. Au-ZSM-5 catalyses the selective oxidation of CH4 to CH3OH and CH3COOH using O2. Nat. Catal. 2022, 5, 45-54.
30. Lewis, R. J.; Bara-Estaun, A.; Agarwal, N.; Freakley, S. J.; Morgan, D. J.; Hutchings, G. J. The direct synthesis of H2O2 and selective oxidation of methane to methanol using HZSM-5 supported AuPd catalysts. Catal. Lett. 2019, 149, 3066-75.
31. Jin, Z.; Wang, L.; Zuidema, E.; et al. Hydrophobic zeolite modification for in situ peroxide formation in methane oxidation to methanol. Science 2020, 367, 193-7.
32. Cao, J.; Qi, G.; Yao, B.; et al. Partially bonded aluminum site on the external surface of post-treated Au/ZSM-5 enhances methane oxidation to oxygenates. ACS. Catal. 2024, 14, 1797-807.
33. Wang, A.; Li, J.; Zhang, T. Heterogeneous single-atom catalysis. Nat. Rev. Chem. 2018, 2, 65-81.
34. Yuan, J.; Zhang, W.; Li, X.; Yang, J. A high performance catalyst for methane conversion to methanol: graphene supported single atom Co. Chem. Commun. 2018, 54, 2284-7.
35. Lou, Y.; Cai, Y.; Hu, W.; et al. Identification of active area as active center for CO oxidation over single Au atom catalyst. ACS. Catal. 2020, 10, 6094-101.
36. Lou, Y.; Ma, J.; Hu, W.; et al. Low-temperature methane combustion over Pd/H-ZSM-5: active Pd sites with specific electronic properties modulated by acidic sites of H-ZSM-5. ACS. Catal. 2016, 6, 8127-39.
37. Cui, X.; Li, H.; Wang, Y.; et al. Room-temperature methane conversion by graphene-confined single iron atoms. Chem 2018, 4, 1902-10.
38. Shan, J.; Li, M.; Allard, L. F.; Lee, S.; Flytzani-Stephanopoulos, M. Mild oxidation of methane to methanol or acetic acid on supported isolated rhodium catalysts. Nature 2017, 551, 605-8.
39. Huang, W.; Zhang, S.; Tang, Y.; et al. Low-temperature transformation of methane to methanol on Pd1O4 single sites anchored on the internal surface of microporous silicate. Angew. Chem. Int. Ed. Engl. 2016, 55, 13441-5.
40. Kwon, Y.; Kim, T. Y.; Kwon, G.; Yi, J.; Lee, H. Selective activation of methane on single-atom catalyst of rhodium dispersed on zirconia for direct conversion. J. Am. Chem. Soc. 2017, 139, 17694-9.
41. Shen, Q.; Cao, C.; Huang, R.; et al. Single chromium atoms supported on titanium dioxide nanoparticles for synergic catalytic methane conversion under mild conditions. Angew. Chem. Int. Ed. Engl. 2020, 59, 1216-9.
42. Gesser, H. D.; Hunter, N. R.; Prakash, C. B. The direct conversion of methane to methanol by controlled oxidation. Chem. Rev. 1985, 85, 235-44.
43. Mahyuddin, M. H.; Shiota, Y.; Yoshizawa, K. Methane selective oxidation to methanol by metal-exchanged zeolites: a review of active sites and their reactivity. Catal. Sci. Technol. 2019, 9, 1744-68.
44. Kulkarni, A. R.; Zhao, Z.; Siahrostami, S.; Nørskov, J. K.; Studt, F. Cation-exchanged zeolites for the selective oxidation of methane to methanol. Catal. Sci. Technol. 2018, 8, 114-23.
45. Gunsalus, N. J.; Koppaka, A.; Park, S. H.; Bischof, S. M.; Hashiguchi, B. G.; Periana, R. A. Homogeneous functionalization of methane. Chem. Rev. 2017, 117, 8521-73.
46. Schwach, P.; Pan, X.; Bao, X. Direct conversion of methane to value-added chemicals over heterogeneous catalysts: challenges and prospects. Chem. Rev. 2017, 117, 8497-520.
47. Zakaria, Z.; Kamarudin, S. Direct conversion technologies of methane to methanol: an overview. Renew. Sustain. Energy. Rev. 2016, 65, 250-61.
48. Panov, G. I.; Uriarte, A. K.; Rodkin, M. A.; Sobolev, V. I. Generation of active oxygen species on solid surfaces. opportunity for novel oxidation technologies over zeolites. Catal. Today. 1998, 41, 365-85.
49. Knops-Gerrits, P.; Goddard, W. Methane partial oxidation in iron zeolites: theory versus experiment. J. Mol. Catal. A. Chem. 2001, 166, 135-45.
50. Wood, B. Methanol formation on Fe/Al-MFI via the oxidation of methane by nitrous oxide. J. Catal. 2004, 225, 300-6.
51. Dubkov, K.; Sobolev, V.; Talsi, E.; et al. Kinetic isotope effects and mechanism of biomimetic oxidation of methane and benzene on FeZSM-5 zeolite. J. Mol. Catal. A. Chem. 1997, 123, 155-61.
52. Groothaert, M. H.; Smeets, P. J.; Sels, B. F.; Jacobs, P. A.; Schoonheydt, R. A. Selective oxidation of methane by the bis(mu-oxo)dicopper core stabilized on ZSM-5 and mordenite zeolites. J. Am. Chem. Soc. 2005, 127, 1394-5.
53. Beznis, N. V.; van, L. A. N.; Weckhuysen, B. M.; Bitter, J. H. Oxidation of methane to methanol and formaldehyde over Co–ZSM-5 molecular sieves: tuning the reactivity and selectivity by alkaline and acid treatments of the zeolite ZSM-5 agglomerates. Micropor. Mesopor. Mat. 2011, 138, 176-83.
54. Bozbag, S. E.; Alayon, E. M. C.; Pecháček, J.; Nachtegaal, M.; Ranocchiari, M.; van, B. J. A. Methane to methanol over copper mordenite: yield improvement through multiple cycles and different synthesis techniques. Catal. Sci. Technol. 2016, 6, 5011-22.
55. Tomkins, P.; Ranocchiari, M.; van, B. J. A. Direct conversion of methane to methanol under mild conditions over Cu-zeolites and beyond. Acc. Chem. Res. 2017, 50, 418-25.
56. Mlekodaj, K.; Lemishka, M.; Kornas, A.; et al. Evolution of active oxygen species originating from O2 cleavage over Fe-FER for application in methane oxidation. ACS. Catal. 2023, 13, 3345-55.
57. Beznis, N. V.; Weckhuysen, B. M.; Bitter, J. H. Partial oxidation of methane over Co-ZSM-5: tuning the oxygenate selectivity by altering the preparation route. Catal. Lett. 2010, 136, 52-6.
58. Shan, J.; Huang, W.; Nguyen, L.; et al. Conversion of methane to methanol with a bent mono(μ-oxo)dinickel anchored on the internal surfaces of micropores. Langmuir 2014, 30, 8558-69.
59. Xu, J.; Zheng, A.; Wang, X.; et al. Room temperature activation of methane over Zn modified H-ZSM-5 zeolites: insight from solid-state NMR and theoretical calculations. Chem. Sci. 2012, 3, 2932.
60. Hammond, C.; Dimitratos, N.; Lopez-Sanchez, J. A.; et al. Aqueous-phase methane oxidation over Fe-MFI zeolites; promotion through isomorphous framework substitution. ACS. Catal. 2013, 3, 1835-44.
61. Sobolev, V.; Dubkov, K.; Panna, O.; Panov, G. Selective oxidation of methane to methanol on a FeZSM-5 surface. Catal. Today. 1995, 24, 251-2.
62. Lipscomb, J. D. Biochemistry of the soluble methane monooxygenase. Annu. Rev. Microbiol. 1994, 48, 371-99.
63. Chan, S. I.; Chen, K. H.; Yu, S. S.; Chen, C. L.; Kuo, S. S. Toward delineating the structure and function of the particulate methane monooxygenase from methanotrophic bacteria. Biochemistry 2004, 43, 4421-30.
64. Lieberman, R. L.; Rosenzweig, A. C. Crystal structure of a membrane-bound metalloenzyme that catalyses the biological oxidation of methane. Nature 2005, 434, 177-82.
65. Lieberman, R. L.; Shrestha, D. B.; Doan, P. E.; Hoffman, B. M.; Stemmler, T. L.; Rosenzweig, A. C. Purified particulate methane monooxygenase from Methylococcus capsulatus (Bath) is a dimer with both mononuclear copper and a copper-containing cluster. Proc. Natl. Acad. Sci. U. S. A. 2003, 100, 3820-5.
66. Yoshizawa, K.; Shiota, Y. Conversion of methane to methanol at the mononuclear and dinuclear copper sites of particulate methane monooxygenase (pMMO): a DFT and QM/MM study. J. Am. Chem. Soc. 2006, 128, 9873-81.
67. Yoshizawa, K. Nonradical mechanism for methane hydroxylation by iron-oxo complexes. Acc. Chem. Res. 2006, 39, 375-82.
68. Solomon, E. I.; Heppner, D. E.; Johnston, E. M.; et al. Copper active sites in biology. Chem. Rev. 2014, 114, 3659-853.
69. Pannov, G.; Sobolev, V.; Kharitonov, A. The role of iron in N2O decomposition on ZSM-5 zeolite and reactivity of the surface oxygen formed. J. Mol. Catal. 1990, 61, 85-97.
70. Yoshizawa, K.; Shiota, Y.; Yumura, T.; Yamabe, T. Direct methane−methanol and benzene−phenol conversions on Fe−ZSM-5 zeolite: theoretical predictions on the reaction pathways and energetics. J. Phys. Chem. B. 2000, 104, 734-40.
71. Groothaert, M. H.; van, B. J. A.; Battiston, A. A.; Weckhuysen, B. M.; Schoonheydt, R. A. Bis(mu-oxo)dicopper in Cu-ZSM-5 and its role in the decomposition of NO: a combined in situ XAFS, UV-vis-near-IR, and kinetic study. J. Am. Chem. Soc. 2003, 125, 7629-40.
72. Smeets, P. J.; Hadt, R. G.; Woertink, J. S.; et al. Oxygen precursor to the reactive intermediate in methanol synthesis by Cu-ZSM-5. J. Am. Chem. Soc. 2010, 132, 14736-8.
73. Tsai, M. L.; Hadt, R. G.; Vanelderen, P.; Sels, B. F.; Schoonheydt, R. A.; Solomon, E. I. [Cu2O]2+ active site formation in Cu-ZSM-5: geometric and electronic structure requirements for N2O activation. J. Am. Chem. Soc. 2014, 136, 3522-9.
74. Beznis, N. V.; Weckhuysen, B. M.; Bitter, J. H. Cu-ZSM-5 zeolites for the formation of methanol from methane and oxygen: probing the active sites and spectator species. Catal. Lett. 2010, 138, 14-22.
75. Woertink, J. S.; Smeets, P. J.; Groothaert, M. H.; et al. A [Cu2O]2+ core in Cu-ZSM-5, the active site in the oxidation of methane to methanol. Proc. Natl. Acad. Sci. U. S. A. 2009, 106, 18908-13.
76. Forde, M. M.; Armstrong, R. D.; Mcvicker, R.; et al. Light alkane oxidation using catalysts prepared by chemical vapour impregnation: tuning alcohol selectivity through catalyst pre-treatment. Chem. Sci. 2014, 5, 3603-16.
77. Hammond, C.; Forde, M. M.; Ab, R. M. H.; et al. Direct catalytic conversion of methane to methanol in an aqueous medium by using copper-promoted Fe-ZSM-5. Angew. Chem. Int. Ed. Engl. 2012, 51, 5129-33.
78. Fang, Z.; Murayama, H.; Zhao, Q.; et al. Selective mild oxidation of methane to methanol or formic acid on Fe–MOR catalysts. Catal. Sci. Technol. 2019, 9, 6946-56.
79. Yu, T.; Su, Y.; Wang, A.; Weckhuysen, B. M.; Luo, W. Efficient synthesis of monomeric Fe species in zeolite ZSM-5 for the low-temperature oxidation of methane. ChemCatChem 2021, 13, 2766-70.
80. Kim, M. S.; Park, K. H.; Cho, S. J.; Park, E. D. Partial oxidation of methane with hydrogen peroxide over Fe-ZSM-5 catalyst. Catal. Today. 2021, 376, 113-8.
81. Cheng, Q.; Li, G.; Yao, X.; et al. Maximizing active Fe species in ZSM-5 zeolite using organic-template-free synthesis for efficient selective methane oxidation. J. Am. Chem. Soc. 2023, 145, 5888-98.
82. Zhang, Q.; Li, J.; He, G.; et al. Regulating mono-/binuclear Fe species in framework Al-rich zeolites for efficient low-temperature alkane oxidation. CCS. Chem.2024.
83. Shahami, M.; Shantz, D. F. Zeolite acidity strongly influences hydrogen peroxide activation and oxygenate selectivity in the partial oxidation of methane over M,Fe-MFI (M: Ga, Al, B) zeolites. Catal. Sci. Technol. 2019, 9, 2945-51.
84. Oda, A.; Aono, K.; Murata, N.; et al. Rational design of ZSM-5 zeolite containing a high concentration of single Fe sites capable of catalyzing the partial oxidation of methane with high turnover frequency. Catal. Sci. Technol. 2022, 12, 542-50.
85. Yu, T.; Li, Z.; Lin, L.; et al. Highly selective oxidation of methane into methanol over Cu-promoted monomeric Fe/ZSM-5. ACS. Catal. 2021, 11, 6684-91.
86. Kim, M. S.; Yang, G. S.; Park, E. D. Effects of Cu species on liquid-phase partial oxidation of methane with H2O2 over Cu-Fe/ZSM-5 catalysts. Catalysts 2022, 12, 1224.
87. Göltl, F.; Michel, C.; Andrikopoulos, P. C.; et al. Computationally exploring confinement effects in the methane-to-methanol conversion over iron-oxo centers in zeolites. ACS. Catal. 2016, 6, 8404-9.
88. Mahyuddin, M. H.; Staykov, A.; Shiota, Y.; Miyanishi, M.; Yoshizawa, K. Roles of zeolite confinement and Cu–O–Cu angle on the direct conversion of methane to methanol by [Cu2(μ-O)]2+ -exchanged AEI, CHA, AFX, and MFI zeolites. ACS. Catal. 2017, 7, 3741-51.
89. Haw, J. F.; Song, W.; Marcus, D. M.; Nicholas, J. B. The mechanism of methanol to hydrocarbon catalysis. Acc. Chem. Res. 2003, 36, 317-26.
90. Snyder, B. E. R.; Bols, M. L.; Rhoda, H. M.; et al. Mechanism of selective benzene hydroxylation catalyzed by iron-containing zeolites. Proc. Natl. Acad. Sci. U. S. A. 2018, 115, 12124-9.
91. Snyder, B. E. R.; Bols, M. L.; Rhoda, H. M.; et al. Cage effects control the mechanism of methane hydroxylation in zeolites. Science 2021, 373, 327-31.
92. Zhu, K.; Liang, S.; Cui, X.; et al. Highly efficient conversion of methane to formic acid under mild conditions at ZSM-5-confined Fe-sites. Nano. Energy. 2021, 82, 105718.
93. Zheng, J.; Ye, J.; Ortuño, M. A.; et al. Selective methane oxidation to methanol on Cu-oxo dimers stabilized by zirconia nodes of an NU-1000 metal-organic framework. J. Am. Chem. Soc. 2019, 141, 9292-304.
94. Liu, C.; Mou, C.; Yu, S. S.; Chan, S. I. Heterogeneous formulation of the tricopper complex for efficient catalytic conversion of methane into methanol at ambient temperature and pressure. Energy. Environ. Sci. 2016, 9, 1361-74.
95. Hammond, C.; Dimitratos, N.; Jenkins, R. L.; et al. Elucidation and evolution of the active component within Cu/Fe/ZSM-5 for catalytic methane oxidation: from synthesis to catalysis. ACS. Catal. 2013, 3, 689-99.
96. Szécsényi, Á.; Li, G.; Gascon, J.; Pidko, E. A. Mechanistic complexity of methane oxidation with H2O2 by single-site Fe/ZSM-5 catalyst. ACS. Catal. 2018, 8, 7961-72.
97. Kalamaras, C.; Palomas, D.; Bos, R.; Horton, A.; Crimmin, M.; Hellgardt, K. Selective oxidation of methane to methanol over Cu- and Fe-exchanged zeolites: the effect of Si/Al molar ratio. Catal. Lett. 2016, 146, 483-92.
98. Yu, T.; Li, Z.; Jones, W.; et al. Identifying key mononuclear Fe species for low-temperature methane oxidation. Chem. Sci. 2021, 12, 3152-60.
99. Zhang, Q.; Li, J.; Li, L.; Yu, J. Zeolite-based materials for greenhouse gas capture and conversion. Sci. China. Chem.2024.
100. Jia, J.; Sun, Q.; Wen, B.; Chen, L. X.; Sachtler, W. M. H. Identification of highly active iron sites in N2O-activated Fe/MFI. Catal. Lett. 2002, 82, 7-11.
101. Chow, Y. K.; Dummer, N. F.; Carter, J. H.; et al. Investigating the influence of acid sites in continuous methane oxidation with N2O over Fe/MFI zeolites. Catal. Sci. Technol. 2018, 8, 154-63.
102. Xiao, P.; Nakamura, K.; Lu, Y.; et al. One-pot synthesized Fe-AEI zeolite catalysts contribute to direct oxidation of methane. ACS. Catal. 2023, 13, 16168-78.
103. Xiao, P.; Wang, L.; Toyoda, H.; et al. Revealing active sites and reaction pathways in direct oxidation of methane over Fe-containing CHA zeolites affected by the Al arrangement. J. Am. Chem. Soc. 2024, 146, 31969-81.
104. Zhu, L.; Xu, Q.; Liu, B.; et al. Ozone-assisted low-temperature oxidation of methane and ethane. P. Combust. Inst. 2023, 39, 375-84.
105. Ipek, B.; Lobo, R. F. Catalytic conversion of methane to methanol on Cu-SSZ-13 using N2O as oxidant. Chem. Commun. 2016, 52, 13401-4.
106. Tsuchimura, Y.; Yoshida, H.; Machida, M.; Nishimura, S.; Takahashi, K.; Ohyama, J. Investigation of the active-site structure of Cu-CHA catalysts for the direct oxidation of methane to methanol using in situ UV-vis spectroscopy. Energy. Fuels. 2023, 37, 9411-8.
107. Bols, M. L.; Devos, J.; Rhoda, H. M.; et al. Selective formation of α-Fe(II) sites on Fe-zeolites through one-pot synthesis. J. Am. Chem. Soc. 2021, 143, 16243-55.
108. Plessers, D.; Heyer, A. J.; Rhoda, H. M.; et al. Tuning copper active site composition in Cu-MOR through Co-cation modification for methane activation. ACS. Catal. 2023, 13, 1906-15.
109. Jeong, Y. R.; Jung, H.; Kang, J.; Han, J. W.; Park, E. D. Continuous synthesis of methanol from methane and steam over copper-mordenite. ACS. Catal. 2021, 11, 1065-70.
110. Xiao, P.; Wang, Y.; Lu, Y.; et al. Effects of Al distribution in the Cu-exchanged AEI zeolites on the reaction performance of continuous direct conversion of methane to methanol. Appl. Catal. B. Environ. 2023, 325, 122395.
111. Artsiusheuski, M. A.; van, B. J. A.; Sushkevich, V. L. Structure of selective and nonselective dicopper (II) sites in CuMFI for methane oxidation to methanol. ACS. Catal. 2022, 12, 15626-37.
112. Xiao, P.; Wang, Y.; Nakamura, K.; et al. Highly effective Cu/AEI zeolite catalysts contribute to continuous conversion of methane to methanol. ACS. Catal. 2023, 13, 11057-68.
113. Brezicki, G.; Kammert, J. D.; Gunnoe, T. B.; Paolucci, C.; Davis, R. J. Insights into the speciation of Cu in the Cu-H-mordenite catalyst for the oxidation of methane to methanol. ACS. Catal. 2019, 9, 5308-19.
114. Kucherov, A. V.; Hubbard, C. P.; Kucherova, T. N.; Shelef, M. Stabilization of the ethane oxidation catalytic activity of Cu-ZSM-5. Appl. Catal. B. Environ. 1996, 7, 285-98.
115. Vanelderen, P.; Hadt, R. G.; Smeets, P. J.; Solomon, E. I.; Schoonheydt, R. A.; Sels, B. F. Cu-ZSM-5: a biomimetic inorganic model for methane oxidation. J. Catal. 2011, 284, 157-64.
116. Alayon, E. M.; Nachtegaal, M.; Ranocchiari, M.; van, B. J. A. Catalytic conversion of methane to methanol using Cu-zeolites. Chimia 2012, 66, 668-74.
117. Dinh, K. T.; Sullivan, M. M.; Narsimhan, K.; et al. Continuous partial oxidation of methane to methanol catalyzed by diffusion-paired copper dimers in copper-exchanged zeolites. J. Am. Chem. Soc. 2019, 141, 11641-50.
118. Narsimhan, K.; Iyoki, K.; Dinh, K.; Román-Leshkov, Y. Catalytic oxidation of methane into methanol over copper-exchanged zeolites with oxygen at low temperature. ACS. Cent. Sci. 2016, 2, 424-9.
119. Sushkevich, V. L.; van, B. J. A. Effect of Brønsted acid sites on the direct conversion of methane into methanol over copper-exchanged mordenite. Catal. Sci. Technol. 2018, 8, 4141-50.
120. Smeets, P. J.; Groothaert, M. H.; Schoonheydt, R. A. Cu based zeolites: a UV-vis study of the active site in the selective methane oxidation at low temperatures. Catal. Today. 2005, 110, 303-9.
121. Artsiusheuski, M. A.; Safonova, O.; Palagin, D.; van, B. J. A.; Sushkevich, V. L. Structural evolution of copper-oxo sites in zeolites upon the reaction with methane investigated by means of Cu K-edge X-ray absorption spectroscopy. J. Phys. Chem. C. 2023, 127, 9603-15.
122. Lee, I.; Lee, M. S.; Tao, L.; et al. Activity of Cu-Al-oxo extra-framework clusters for selective methane oxidation on Cu-exchanged zeolites. JACS. Au. 2021, 1, 1412-21.
123. Khramenkova, E. V.; Medvedev, M. G.; Li, G.; Pidko, E. A. Unraveling the nature of extraframework catalytic ensembles in zeolites: flexibility and dynamics of the copper-oxo trimers in mordenite. J. Phys. Chem. Lett. 2021, 12, 10906-13.
124. Xiao, P.; Wang, Y.; Wang, L.; et al. Understanding the effect of spatially separated Cu and acid sites in zeolite catalysts on oxidation of methane. Nat. Commun. 2024, 15, 2718.
125. Wijerathne, A.; Sawyer, A.; Daya, R.; Paolucci, C. Competition between mononuclear and binuclear copper sites across different zeolite topologies. JACS. Au. 2024, 4, 197-215.
126. Wang, Y.; Han, J.; Chen, M.; et al. Low-silica Cu-CHA zeolite enriched with Al pairs transcribed from silicoaluminophosphate seed: synthesis and ammonia selective catalytic reduction performance. Angew. Chem. Int. Ed. Engl. 2023, 62, e202306174.
127. Brezicki, G.; Zheng, J.; Paolucci, C.; Schlögl, R.; Davis, R. J. Effect of the Co-cation on Cu speciation in Cu-exchanged mordenite and ZSM-5 catalysts for the oxidation of methane to methanol. ACS. Catal. 2021, 11, 4973-87.
128. Gabrienko, A. A.; Yashnik, S. A.; Kolganov, A. A.; et al. Methane activation on H-ZSM-5 zeolite with low copper loading. the nature of active sites and intermediates identified with the combination of spectroscopic methods. Inorg. Chem. 2020, 59, 2037-50.
129. Ohyama, J.; Tsuchimura, Y.; Hirayama, A.; et al. Relationships among the catalytic performance, redox activity, and structure of Cu-CHA catalysts for the direct oxidation of methane to methanol investigated using in situ XAFS and UV-vis spectroscopies. ACS. Catal. 2022, 12, 2454-62.
130. Göltl, F.; Bhandari, S.; Lebrón-Rodríguez, E. A.; et al. Identifying hydroxylated copper dimers in SSZ-13 via UV-vis-NIR spectroscopy. Catal. Sci. Technol. 2022, 12, 2744-8.
131. Li, H.; Paolucci, C.; Khurana, I.; et al. Consequences of exchange-site heterogeneity and dynamics on the UV-visible spectrum of Cu-exchanged SSZ-13. Chem. Sci. 2019, 10, 2373-84.
132. Rhoda, H. M.; Plessers, D.; Heyer, A. J.; et al. Spectroscopic definition of a highly reactive site in Cu-CHA for selective methane oxidation: tuning a mono-μ-oxo dicopper(II) active site for reactivity. J. Am. Chem. Soc. 2021, 143, 7531-40.
133. Zhang, Y.; Zhang, J.; Wang, H.; et al. Selective catalytic reduction of NOx with NH3 over Cu/SSZ-13: elucidating dynamics of Cu active sites with in situ UV-vis spectroscopy and DFT calculations. J. Phys. Chem. C. 2022, 126, 8720-33.
134. Tang, X.; Wang, L.; Yang, B.; et al. Direct oxidation of methane to oxygenates on supported single Cu atom catalyst. Appl. Catal. B. Environ. 2021, 285, 119827.
135. Heyer, A. J.; Plessers, D.; Ma, J.; et al. Magnetic exchange coupling in zeolite copper dimers and its contribution to methane activation. J. Am. Chem. Soc. 2024, 146, 6061-71.
136. Li, G.; Vassilev, P.; Sanchez-Sanchez, M.; Lercher, J. A.; Hensen, E. J.; Pidko, E. A. Stability and reactivity of copper oxo-clusters in ZSM-5 zeolite for selective methane oxidation to methanol. J. Catal. 2016, 338, 305-12.
137. Armstrong, R. D.; Peneau, V.; Ritterskamp, N.; Kiely, C. J.; Taylor, S. H.; Hutchings, G. J. The role of copper speciation in the low temperature oxidative upgrading of short chain alkanes over Cu/ZSM-5 catalysts. Chemphyschem 2018, 19, 469-78.
138. Grundner, S.; Markovits, M. A.; Li, G.; et al. Single-site trinuclear copper oxygen clusters in mordenite for selective conversion of methane to methanol. Nat. Commun. 2015, 6, 7546.
139. Yuan, Q.; Deng, W.; Zhang, Q.; Wang, Y. Osmium-catalyzed selective oxidations of methane and ethane with hydrogen peroxide in aqueous medium. Adv. Synth. Catal. 2007, 349, 1199-209.
140. Kulkarni, A. R.; Zhao, Z.; Siahrostami, S.; Nørskov, J. K.; Studt, F. Monocopper active site for partial methane oxidation in Cu-exchanged 8MR zeolites. ACS. Catal. 2016, 6, 6531-6.
141. Sushkevich, V. L.; Artsiusheuski, M.; Klose, D.; Jeschke, G.; van, B. J. A. Identification of kinetic and spectroscopic signatures of copper sites for direct oxidation of methane to methanol. Angew. Chem. Int. Ed. Engl. 2021, 60, 15944-53.
142. Mahyuddin, M. H.; Staykov, A.; Shiota, Y.; Yoshizawa, K. Direct conversion of methane to methanol by metal-exchanged ZSM-5 zeolite (metal = Fe, Co, Ni, Cu). ACS. Catal. 2016, 6, 8321-31.
143. Pierella, L. B.; Saux, C.; Caglieri, S. C.; Bertorello, H. R.; Bercoff, P. G. Catalytic activity and magnetic properties of Co–ZSM-5 zeolites prepared by different methods. Appl. Catal. A. Gen. 2008, 347, 55-61.
144. Mahyuddin, M. H.; Tanaka, S.; Kitagawa, R.; et al. Distinct behaviors of Cu- and Ni-ZSM-5 zeolites toward the post-activation reactions of methane. J. Phys. Chem. C. 2021, 125, 19333-44.
145. Mahyuddin, M. H.; Yoshizawa, K. DFT exploration of active site motifs in methane hydroxylation by Ni-ZSM-5 zeolite. Catal. Sci. Technol. 2018, 8, 5875-85.
146. Xiao, P.; Wang, Y.; Lu, Y.; et al. Direct oxidation of methane to methanol over transition-metal-free ferrierite zeolite catalysts. J. Am. Chem. Soc. 2024, 146, 10014-22.
147. Wang, L.; Zhang, S.; Zhu, Y.; et al. Catalysis and in situ studies of Rh1/Co3O4 nanorods in reduction of NO with H2. ACS. Catal. 2013, 3, 1011-9.
148. Nguyen, L.; Zhang, S.; Wang, L.; et al. Reduction of nitric oxide with hydrogen on catalysts of singly dispersed bimetallic sites Pt1Com and Pd1Con. ACS. Catal. 2016, 6, 840-50.
149. Zhang, S.; Nguyen, L.; Liang, J. X.; et al. Catalysis on singly dispersed bimetallic sites. Nat. Commun. 2015, 6, 7938.
150. Bai, S.; Liu, F.; Huang, B.; et al. High-efficiency direct methane conversion to oxygenates on a cerium dioxide nanowires supported rhodium single-atom catalyst. Nat. Commun. 2020, 11, 954.
151. Zhu, J.; Osuga, R.; Ishikawa, R.; et al. Ultrafast encapsulation of metal nanoclusters into MFI zeolite in the course of its crystallization: catalytic application for propane dehydrogenation. Angew. Chem. Int. Ed. Engl. 2020, 59, 19669-74.
152. Tao, F. F.; Shan, J. J.; Nguyen, L.; et al. Understanding complete oxidation of methane on spinel oxides at a molecular level. Nat. Commun. 2015, 6, 7798.
153. Guo, X.; Fang, G.; Li, G.; et al. Direct, nonoxidative conversion of methane to ethylene, aromatics, and hydrogen. Science 2014, 344, 616-9.
154. Bai, S.; Yao, Q.; Xu, Y.; Cao, K.; Huang, X. Strong synergy in a lichen-like RuCu nanosheet boosts the direct methane oxidation to methanol. Nano. Energy. 2020, 71, 104566.
155. Ab Rahim, M. H.; Forde, M. M.; Hammond, C.; et al. Systematic study of the oxidation of methane using supported gold palladium nanoparticles under mild aqueous conditions. Top. Catal. 2013, 56, 1843-57.
156. Bai, S.; Xu, Y.; Wang, P.; Shao, Q.; Huang, X. Activating and converting CH4 to CH3OH via the CuPdO2/CuO nanointerface. ACS. Catal. 2019, 9, 6938-44.
157. Plauck, A.; Stangland, E. E.; Dumesic, J. A.; Mavrikakis, M. Active sites and mechanisms for H2O2 decomposition over Pd catalysts. Proc. Natl. Acad. Sci. U. S. A. 2016, 113, E1973-82.
158. Flaherty, D. W. Direct synthesis of H2O2 from H2 and O2 on Pd catalysts: current understanding, outstanding questions, and research needs. ACS. Catal. 2018, 8, 1520-7.
159. Agarwal, N.; Freakley, S. J.; McVicker, R. U.; et al. Aqueous Au-Pd colloids catalyze selective CH4 oxidation to CH3OH with O2 under mild conditions. Science 2017, 358, 223-7.
160. Williams, C.; Carter, J. H.; Dummer, N. F.; et al. Selective oxidation of methane to methanol using supported AuPd catalysts prepared by stabilizer-free sol-immobilization. ACS. Catal. 2018, 8, 2567-76.
161. He, Y.; Liang, J.; Imai, Y.; et al. Highly selective synthesis of methanol from methane over carbon materials supported Pd-Au nanoparticles under mild conditions. Catal. Today. 2020, 352, 104-10.
162. Freakley, S. J.; Kochius, S.; van, M. J.; et al. A chemo-enzymatic oxidation cascade to activate C-H bonds with in situ generated H2O2. Nat. Commun. 2019, 10, 4178.
163. Crombie, C. M.; Lewis, R. J.; Taylor, R. L.; et al. Enhanced selective oxidation of benzyl alcohol via in situ H2O2 production over supported Pd-based catalysts. ACS. Catal. 2021, 11, 2701-14.
164. Ab Rahim, M. H.; Forde, M. M.; Jenkins, R. L.; et al. Oxidation of methane to methanol with hydrogen peroxide using supported gold-palladium alloy nanoparticles. Angew. Chem. Int. Ed. Engl. 2013, 52, 1280-4.
165. Wu, B.; Lin, T.; Huang, M.; et al. Tandem catalysis for selective oxidation of methane to oxygenates using oxygen over PdCu/zeolite. Angew. Chem. Int. Ed. Engl. 2022, 61, e202204116.
166. Cheng, Q.; Yao, X.; Li, G.; et al. Atomically dispersed iron-copper dual-metal sites synergistically boost carbonylation of methane. Angew. Chem. Int. Ed. Engl. 2024, 63, e202411048.
167. Moteki, T.; Tominaga, N.; Ogura, M. Mechanism investigation and product selectivity control on CO-assisted direct conversion of methane into C1 and C2 oxygenates catalyzed by zeolite-supported Rh. Appl. Catal. B. Environ. 2022, 300, 120742.
168. Narsimhan, K.; Michaelis, V. K.; Mathies, G.; Gunther, W. R.; Griffin, R. G.; Román-Leshkov, Y. Methane to acetic acid over Cu-exchanged zeolites: mechanistic insights from a site-specific carbonylation reaction. J. Am. Chem. Soc. 2015, 137, 1825-32.
169. Wang, X.; Qi, G.; Xu, J.; Li, B.; Wang, C.; Deng, F. NMR-spectroscopic evidence of intermediate-dependent pathways for acetic acid formation from methane and carbon monoxide over a ZnZSM-5 zeolite catalyst. Angew. Chem. Int. Ed. Engl. 2012, 51, 3850-3.
170. Blasco, T.; Boronat, M.; Concepción, P.; Corma, A.; Law, D.; Vidal-Moya, J. A. Carbonylation of methanol on metal-acid zeolites: evidence for a mechanism involving a multisite active center. Angew. Chem. Int. Ed. Engl. 2007, 46, 3938-41.
171. Boronat, M.; Martínez-Sánchez, C.; Law, D.; Corma, A. Enzyme-like specificity in zeolites: a unique site position in mordenite for selective carbonylation of methanol and dimethyl ether with CO. J. Am. Chem. Soc. 2008, 130, 16316-23.
172. Moteki, T.; Tominaga, N.; Ogura, M. CO-assisted direct methane conversion into C1 and C2 oxygenates over ZSM-5 supported transition and platinum group metal catalysts using oxygen as an oxidant. ChemCatChem 2020, 12, 2957-61.
173. Wang, C.; Sun, Y.; Wang, L.; et al. Oxidative carbonylation of methane to acetic acid on an Fe-modified ZSM-5 zeolite. Appl. Catal. B. Environ. 2023, 329, 122549.
174. Tang, Y.; Li, Y.; Fung, V.; et al. Single rhodium atoms anchored in micropores for efficient transformation of methane under mild conditions. Nat. Commun. 2018, 9, 1231.
175. Cao, J.; Lewis, R. J.; Qi, G.; et al. Methane conversion to methanol using Au/ZSM-5 is promoted by carbon. ACS. Catal. 2023, 13, 7199-209.
Cite This Article
How to Cite
Download Citation
Export Citation File:
Type of Import
Tips on Downloading Citation
Citation Manager File Format
Type of Import
Direct Import: When the Direct Import option is selected (the default state), a dialogue box will give you the option to Save or Open the downloaded citation data. Choosing Open will either launch your citation manager or give you a choice of applications with which to use the metadata. The Save option saves the file locally for later use.
Indirect Import: When the Indirect Import option is selected, the metadata is displayed and may be copied and pasted as needed.
About This Article
Special Issue
Copyright
Data & Comments
Data
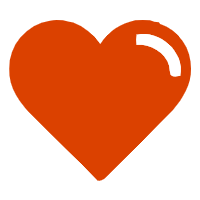
Comments
Comments must be written in English. Spam, offensive content, impersonation, and private information will not be permitted. If any comment is reported and identified as inappropriate content by OAE staff, the comment will be removed without notice. If you have any queries or need any help, please contact us at [email protected].