Copper-doped TiO2 photocatalyst for advanced oxidation processes: reactive oxygen species generation mechanisms
Abstract
Copper-doped anatase TiO2 (Cu/TiO2) has attracted significant attention in various sustainable chemical processes, including water splitting, carbon monoxide oxidation, carbon dioxide reduction, chemical synthesis, and advanced oxidation processes for water treatment. Reactive oxygen species (ROS) are involved in these processes, but a mechanistic understanding of ROS generation on Cu/TiO2 surfaces has not been established. Combining experimental investigation and computational simulation, this work provides unequivocal evidence for superoxide radical anion (O2•-) formation via reduction of the adsorbed oxygen by Cu+ and hydroxyl radical (•OH) production by oxidation of lattice oxygen within the bridging Cu-O-Ti structure on Cu/TiO2 surfaces. Under visible light irradiation, the ROS generation rates of Cu/TiO2 are 7.2 times higher for O2•- and 11.2 times higher for •OH than those of undoped TiO2. The superior performance of Cu/TiO2 has been demonstrated through its organic dye degradation, bactericidal activity, and biofilm disruption, indicating its wide applicability in water treatment and disinfection. The results and the methodologies will benefit the wide field of heterogeneous redox chemistry.
Keywords
INTRODUCTION
Reactive oxygen species (ROS), such as hydroxyl radicals (•OH) and superoxide radical anions (O2•-), are of prime importance in advanced oxidation processes for water treatment. Titanium dioxide (TiO2), a heterogeneous photocatalyst, has been a research focus for decades because of its ability to absorb sunlight, efficient photon-to-current conversion, high chemical stability, and low toxicity[1]. However, pristine anatase has a large optical band gap (~3.2 eV) that restricts photon absorption to the ultraviolet (UV) range, which comprises only ~5% of the solar spectrum, thus limiting its energy conversion efficiency. Photocatalytic materials activated solely by visible light are particularly valuable, especially when paired with energy-efficient and cost-effective visible light-emitting diodes (LEDs)[2]. These LEDs are compact, affordable, environmentally friendly, and suitable for indoor use or scenarios where sunlight is unavailable. To enhance its absorption in the visible region, researchers have narrowed its band gap via dye sensitization[3], doping[4,5], defect engineering[6,7], and by fabricating composite materials[8-10].
Nanostructured photocatalysts based on transition metal-doped TiO2 have enabled the development of efficient and sustainable photocatalysts for environmental, chemical, and energy applications[11,12], such as water splitting[13,14], hydrogen generation[15-17], carbon monoxide oxidation[17,18], carbon dioxide reduction[19-21], hydrogen activation[22-24], organic synthesis[25,26], and the water-gas shift reaction[27-29], among others. Catalysis using doped semiconductors is at the interface between homogeneous and heterogeneous catalysis in that they have discrete active sites that confer selective reactivity[30]; meanwhile, the semiconductor support also provides photocatalytic properties and the benefits of heterogeneous systems such as partitioning and filterability. Many insights regarding the structure-reactivity relationships of metal-doped TiO2 have been discerned[16,18,31,32]; however, little research has investigated the atomic mechanisms that govern ROS generation in water and their impact on water treatment practices, such as the degradation of organic pollutants and disruption of bacteria and biofilms.
To fill this gap, this work documents the first mechanistic study of ROS production on the surface of copper-doped anatase (Cu/TiO2). First, the experimental data unambiguously showed that the surface Cu atoms are the oxygen reduction sites for O2•- production and the bridging Cu-O-Ti structures are the active centers for •OH generation. Second, the surface-dispersed Cu atoms accelerate ROS generation by increasing oxygen adsorption and surface hydrophilicity. Third, the Cu-doping modifies the electronic structure of anatase, narrows its band gap, improves charge separation, and facilitates charge migration by reducing the internal and charge transfer resistances (CTR). Finally, its promise for water treatment was demonstrated with high bactericidal, biofilm disruption, and organic dye degradation efficiencies. These discoveries not only establish Cu/TiO2 as an excellent photocatalyst but also enhance the fundamental understanding of transition metal-doped TiO2 catalysts in general, which may broaden their applicability in practice.
EXPERIMENTAL
Chemicals and materials
Titanium isopropoxide (97%), cupric acetate monohydrate [Cu(OAc)2·H2O, 98%], Rhodamine B (RhB) (95%), 1,4-benzoquinone (BQ, 98%), sodium azide (99.5%), ammonium oxalate monohydrate (99.5%), 5,5-dimethyl-1-pyrroline N-oxide (DMPO, 99%), organic solvents (≥ 99%) (n-pentane, n-hexane, n-heptane, n-octane, and n-decane), coumarin (98%), and 2,3-bis(2-methoxy-4-nitro-5-sulfophenyl)-2H-tetrazolium-5-carboxanilide (XTT sodium salt, 90%) were purchased from Sigma Aldrich (Oakville, ON, Canada). Isopropanol (99%), anhydrous ethanol, and hydrochloric acid (37%) were purchased from Thermo Fisher Scientific (Waltham, MA, USA). Methanol (95%) was purchased from VWR International Ltd. (Radnor, PA, USA). Type 1 water (18.2 MΩ·cm) was obtained from a Millipore Simplicity Water Purification System (EMD Millipore, Burlington, MA, USA). All reagents were of analytical grade and were used as received without further purification.
Analytical and characterization methods
The surface hydrophilicities of the Cu/TiO2 and undoped TiO2 nanoparticles (NPs) were evaluated using the maximum particle dispersion (MPD) method[33]. Probing liquids were composed of aqueous ethanol in various ratios to form five binary mixtures ranging in surface tension from 22 mJ/m2 (for pure ethanol) to 72 mJ/m2 (for pure water). The NP sample (2 mg) was added to the series of probing liquids, each at 0.5 mL, vortexed for 1 min, and left undisturbed for 1 h to allow for natural sedimentation. After sedimentation,
Powder X-ray diffraction (XRD) measurements measured the crystalline structure and phase. The measurements were performed using a Rigaku Ultima IV X-ray diffractometer (Rigaku, Tokyo, Japan) with Cu Kα radiation (λ = 1.54 Å) and a diffracted beam monochromator using a step scan mode with a step of 0.05° (2θ) and 3 counts/s per step. The samples were placed on a zero-background Si wafer. Anatase TiO2 powder diffraction patterns were compared with reference to the International Center for Diffraction Data (ICDD) database[34,35].
The micromorphological structures were investigated by transmission electron microscopy (TEM) using a Hitachi HT7700 (Hitachi, Tokyo, Japan) at an operating voltage of 80-120 kV. The samples were dispersed in ethanol and sonicated for 20 min. The dispersion was then drop-cast onto carbon-coated Ni TEM grids for analysis. The particle size distribution was obtained by measuring the individual diameters of ~100 particles from representative TEM images. The high-angle annular dark-field (HAADF) images in scanning TEM (STEM) mode were recorded using a Thermo Fisher Scientific Talos 200X (Waltham, MA, USA) at an operating voltage of 200 kV with a spot size of < 1 nm, and the convergence semi-angle of 10.5 mrad for analyzing Cu distribution information. The particles were drop-cast onto a Ni grid to avoid contamination from Cu grids typically used in electron microscopy experiments.
The X-ray photoelectron spectra (XPS) were measured using a Thermo VG Scientific Multilab 2000 spectrometer (Waltham, MA, USA), and high-resolution spectra were recorded with a pass energy of 30 eV and a step size of 0.1 eV. The spectra were fitted using CasaXPS software. All binding energies were referenced to the C 1s peak at 284.8 eV of the surface adventitious carbon.
UV-visible diffuse reflectance spectra (UV-Vis DRS) were measured by a SILVER-Nova spectrophotometer (StellarNet, Tampa, FL, USA) with a diffuse reflectance accessory over the range of 300-1,000 nm. The optical band gap energy was evaluated from the absorbance spectra through a Tauc plot, i.e., [F(Rα) × hν]1/2 against hν, where hν is the energy of the incident photon and F(Rα) is the reflection in the Kubelka–Munk function[36]. The linear part of the curve was extrapolated to zero reflectance and the band gap energy was derived.
Surface charges of the Cu/TiO2 NPs under various pH (pH 3, 5, 7, 9, and 11) were measured in triplicate using a LitesizerTM 500 Particle Analyzer (Anton Paar, Graz, Austria) using dynamic light scattering (DLS). The dry powder form of Cu/TiO2 was suspended in deionized water at a concentration of 50 µg/mL and then sonicated in a bath sonicator for 4 h to form a homogeneous dispersion before Zeta potential measurements were performed at room temperature.
Electrochemical experiments
The transient photocurrent measurements, electrochemical impedance spectroscopy (EIS) measurements, and Mott–Schottky experiments were performed on a Corrtest CS2150 bipotentiostat electrochemical workstation (Wuhan, China) in a three-electrode cell with an applied potential of +0.6 V. NP-coated fluorine-doped tin oxide (FTO) served as the working electrode. Ag/AgCl and Pt mesh served as the reference and counter electrodes, respectively, and aqueous Na2SO4 (0.5 M) was used as the electrolyte solution. The photocurrent responses of the NPs were measured under 100 mW·cm-2 LED (400-800 nm, Dongguan, China) illumination intensity to simulate one sun. The light intensity of all experiments was consistent and measured using a PM100USB power detector (Thorlabs Inc., Newton, NJ, USA) with a standard photodiode sensor. Following the protocol suggested by the manufacturer, the FTO substrates were washed sequentially with toluene, methanol, acetone, ethanol, and distilled water using ultrasonication for 20 min in each solvent. Subsequently, to prepare the working electrode, 15 mg of the as-synthesized NPs were suspended in 20 µL of Nafion solution (5 wt%) and 0.5 mL of ethanol[37]. The obtained mixture was ground to form a slurry, which was then evenly spread as a thin film onto an FTO glass substrate with an active area of 1.0 cm2 by drop casting. The coated FTO working electrode was then dried at room temperature. EIS was performed in the dark and under illumination in the frequency range of 0.1 Hz to
where C is the interfacial capacitance, A is the interfacial area, ε0 is the permittivity in vacuum, εr is the relative permittivity, ND is the donor density, E is the externally applied potential, and T is the absolute temperature, e corresponds to the electronic charge, and kB is the Boltzmann constant. Consequently, a plot of 1/C2 versus applied potential (E) will produce a line, which, when extrapolated to the x-axis, will indicate the flat-band potential of the semiconductor[37]. Potentials were referenced to the reversible hydrogen electrode (RHE) using the Nernst equation[39]:
Where
Furthermore, the conduction band (CB) and valence band (VB) edge positions of a semiconductor were determined using[40,41]
where ECB represents the CB edge, Eg is the band gap energy, EVB represents the VB edge, T1 is the semiconductor temperature (298 K), Nsc is the donor density (cm-1), and NCB is the effective density of states in the CB (7.8 × 1020 cm-3)[42].
Photocatalytic degradation experiment
RhB photodegradation in water was monitored to evaluate the photocatalytic performance of Cu/TiO2 and undoped TiO2 NPs. The mixture, including RhB (0.005 mg/mL, 20 mL) and TiO2 NPs (20 mg), was irradiated with a 100 W LED visible light source. A wavelength filter of ≥ 400 nm was employed in all the photodegradation experiments to remove any UV light from the irradiation unless noted otherwise. The mixture was stirred at 400 rpm for 30 min in the dark to reach the adsorption equilibrium. The initial concentration of RhB was measured before the adsorption process, which was used to identify the dye adsorption ability of the NPs. The total irradiation time was 120 min, and the experiments were performed at room temperature. At regular 20-min intervals, 0.4 mL of the reaction mixture was taken, and the supernatant was isolated by centrifuging at 3,900 rpm for 10 min. The visible light absorbance of the supernatants was analyzed by the absorbance program of a FLUOstar Omega microplate reader (BMG Labtech, Cary, NC, USA). Unless stated otherwise, all RhB dye absorbance measurements were performed in a 96-well CorningTM plate (Corning, NY, USA). All photodegradation experiments were performed in triplicate with high reproducibility [relative standard deviation (RSD) < 1%]. Blank groups were performed in the absence of the catalysts under light irradiation. To test the effect of pH on RhB degradation, the pH of the samples was modified by drop-wise introduction of HCl (0.1 M) and NaOH (0.1 M) solutions to pH 3, 5, 7, 9, and 11, before performing the photodegradation and visible light absorbance measurement.
Identification and quantification of ROS
Scavenger test
The detailed ROS formation pathway of the photocatalytic dye degradation under neutral conditions was elucidated through the use of different selective scavengers that target h+, O2•-, •OH, and 1O2. The scavengers were added to photodegradation experiments where the final Cu/TiO2 NP concentration was 1 mg/mL. The RhB photodegradation rate will decrease in the presence of specific scavengers that are responsible for its degradation under the reaction conditions. h+, O2•-, •OH, and 1O2 were scavenged by ammonium oxalate (AO, 10 mM), BQ (0.1 mM), methanol (200 mM), and sodium azide (200 mM), respectively[43-45].
Probe test
For the probe test to assess the ROS generation efficiency, two separate experiments were conducted using different probes for •OH and O2•-, respectively. For •OH detection, a suspension was prepared by sonicating 20 mg of Cu/TiO2 NPs in deionized water for 20 min. Coumarin was then added to this suspension in the dark and stirred at 400 rpm for 30 min to achieve adsorption equilibrium before dilution to a final volume of 20 mL with a final coumarin concentration of 100 µM. The prepared solution was irradiated under a
where It represents the fluorescence intensity of the probe (coumarin) after irradiation at a given time t, I0 denotes the initial fluorescence intensity of coumarin before irradiation, and
For detecting O2•-, a similar procedure using a final concentration of XTT of 2 mM was used and the absorbance of the product was recorded at 473 nm using the absorbance program of the FLUOstar Omega Microplate Reader. The efficiency of superoxide ion formation was determined using
Similarly, At represents the light absorbance of the probe (XTT) after irradiation over the time interval t, A0 denotes its initial absorbance, and
To determine the ROS formation efficiency under irradiation by various light sources, the probe experiment was repeated using distinct cut-off filters at 400, 420, 450, and 500 nm (Foric Optical Factory, Nantong, China). These filters were used to selectively remove wavelengths shorter than the specified cut-offs from the LED light source, allowing for the assessment of ROS production efficiency at or above 400, 420, 450, and 500 nm. For anaerobic experiments, the RhB-Cu/TiO2 NP dispersions were sparged by nitrogen
EPR experiment
To further confirm •OH formation, the Cu/TiO2 NPs (5 mg) were sonicated in deionized water for 20 min to give a suspension. Subsequently, 20 µL of DMPO was added and shaken for a few seconds. In the final mixture, the NP and DMPO concentrations remained at 1 mg/mL and 20 mM, respectively. The mixture was quickly added to a capillary tube and was irradiated in situ with 100 W LED light for 30 s, before recording their electron paramagnetic resonance (EPR) spectra at 273 K. To identify O2•-, the experiments were carried out similarly to that for •OH detection; the only difference is using methanol as the solvent (not water, methanol quenches •OH radicals). The EPR experiments were conducted using a SPINSCAN X spectrometer (Linev Systems, Conroe, TX, USA) operating at 9.200-9.500 GHz with a 100 kHz modulation frequency. The EPR parameters were as follows: field sweep, 15.0 mT; microwave frequency, 9.406 GHz; modulation amplitude, 100 uT; time constant, 0.046 s; and center field, 336.00 mT.
Antibacterial experiments
Bactericidal efficacy
E. coli strains were used to test the bactericidal effect of Cu/TiO2. Before the antibacterial experiment, E. coli was aerobically incubated at 37 ± 0.1 °C for 24 h in Luria–Bertani (LB) broth and then harvested by centrifugation at 3,500 rpm for 5 min before resuspending in saline solution (0.85%, pH = 7) for rinsing. After another 5-min centrifugation, E. coli was resuspended in a fresh saline solution. The turbidity of the
In the antibacterial experiment, a 200 mL Pyrex® crystallizing dish was used as the reaction vessel. First, 197.5 mL of sterilized saline (0.85%, pH = 7) was added to the dish. Then, NPs (200 mg) and 2.5 mL of the E. coli solution were added to the dish successively. The final concentrations of the NPs and microorganisms were 1 mg/mL and 1 × 106 CFU/mL, respectively. The mixture was placed on a magnetic stirrer to keep the NPs well dispersed during the experiment. The total irradiation time was 180 min. The mixture was irradiated with a 100 W LED lamp equipped with a UV cut-off filter (λ > 400 nm) with a light intensity of 100 mW·cm-2. The experiments were performed at room temperature and neutral pH. The solution temperature was maintained between 25-28 °C. During the experiment, sample aliquots (30 µL) were collected at 20-min intervals and spread uniformly on TS agar plates. Finally, the plates were incubated at 37 °C for 24 h before counting the E. coli colonies on each plate. The existence of bacteria was calculated using Chick’s model, following
where η is the antibacterial efficiency, t is the irradiation time, N0 represents the initial number of colonies, and Nt represents the number of E. coli at each time interval.
Antibiofilm assay
A crystal violet (CV) assay was used to evaluate biofilm disruption of Cu/TiO2. First, to grow the biofilm, fully grown E. coli was diluted in fresh LB broth, and the bacteria concentration was adjusted to an optical density (OD) ~0.1 at 600 nm. Next, 2 mL of diluted culture was added to a 48-well cell culture plate (Corning Costar) and incubated for 48 h at 37 °C without shaking to allow for biofilm formation. Bacterial growth was indicated by measuring absorbance at OD 600. After incubation, the biofilm was rinsed with 85% saline solution three times to remove planktonic bacteria. Subsequently, the biofilm was treated with
Computational simulation
The anatase TiO2 unit cell was designed using Crystal Maker X and one Ti atom was substituted with Cu to model Cu/TiO2. Water and oxygen models were added to the system to model the oxygen adsorption process in water. The system was minimized by using the conjugate gradient method over a duration of
RESULTS AND DISCUSSION
Synthesis and characterization of Cu/TiO2
The Cu/TiO2 and undoped TiO2 NPs were synthesized using a solvothermal alcoholysis method
Figure 1. Characterization of Cu/TiO2 nanocatalyst (Cu/TiO2). (A) The HAADF scanning transmission electron microscopic image and elemental maps of Cu/TiO2 NPs; High-resolution XPS spectra: the Ti 2p region for (B) TiO2 and (C) Cu/TiO2; (D) The UV-Vis DRS of TiO2 and Cu/TiO2; (E) Photographs of TiO2 and Cu/TiO2 powders. HAADF: High-angle annular dark-field; NPs: nanoparticles; XPS: X-ray photoelectron spectra; UV-Vis DRS: ultraviolet-visible diffuse reflectance spectra.
The elemental composition of Cu/TiO2 determined using energy-dispersive X-ray (EDX) spectroscopy showed that the atomic fraction of Cu is 0.16% in the crystal, ~200 times lower than that of Ti atoms (~32%) in the NP lattice [Supplementary Table 1]. EDX elemental mapping [Figure 1A, Supplementary Figure 2D and E] shows a uniform distribution of Cu atoms within the TiO2 crystal structure, indicating that the atomic ratio of Cu:Ti was 1:200 on both the surface and in the bulk of each Cu/TiO2 NP. The chemical compositions and states of the Cu/TiO2 and undoped TiO2 NP surfaces were characterized via X-ray photoelectron spectroscopy (XPS). The high-resolution spectrum for Ti 2p on undoped TiO2 NPs [Figure 1B] primarily features two peaks corresponding to the Ti4+ oxidation state: Ti4+ 2p3/2 and Ti4+ 2p1/2 at binding energies of 458.6 and 464.3 eV, respectively. The Cu-doping led to a small shift (~0.3 eV) of the Ti4+ 2p3/2 and Ti4+ 2p1/2 binding energies to 458.9 and 464.6 eV, respectively, in the Cu/TiO2 sample [Figure 1C]. The increase in binding energy is attributed to the incorporation of Cu2+ ions into the TiO2 lattice, indicating the formation of Ti-O-Cu linkages[18,49-51]. The Ti3+ 2p3/2 and Ti3+ 2p1/2 peaks were observed at 457.2 and 462.4 eV, respectively, and a ~36% increase in Ti3+ peak area was observed in Cu/TiO2 relative to that of undoped TiO2 NPs. Previous research has established that oxygen vacancies (OVs) reside exclusively in the subsurface bulk[52]. The extra electrons associated with the OVs are transferred to an empty 3d orbital of adjacent Ti4+ atoms in the TiO2 lattice forming Ti3+ sites[53,54]. Supplementary Figure 4A and B shows the O 1s spectra of doped and undoped TiO2 that were deconvoluted into two peaks at ~530 and 531 eV, which were respectively assigned to the characteristic peaks of (1) the subsurface lattice oxygen and (2) the surface oxygen including the surface lattice oxygen and the chemisorbed oxygen[51,55]. A 37.4% increase in surface oxygen was observed for Cu/TiO2 relative to undoped TiO2 NPs which is attributed to more dissolved oxygen (DO) chemisorbed onto Cu/TiO2 surfaces because of the high affinity of the surface Cu2+ towards oxygen molecules[51]. This was further validated using computational simulation of the adsorption of DO in water on the Cu/TiO2 surfaces [Supplementary Figure 5], concurring with previous research[56]. Further, Cu-doping increased the hydrophilicity of the NP surface [Supplementary Figure 6], where Cu also serves as the adsorption site of water based on computational simulation [Supplementary Figure 7], which is important for forming •OH on the NP surfaces.
The electronic structure of Cu/TiO2
Broad light absorption and efficient charge separation are critical for enhanced photocatalytic activity[57,58]. To estimate the optical band gap of Cu/TiO2, UV-Visible diffuse reflectance spectroscopy was employed to measure its light absorbance. As shown in Figure 1D, the Cu/TiO2 NPs exhibited a noticeable redshift in absorption relative to undoped TiO2, attributed to the 2Eg → 2T2g transitions from lattice O to the doped Cu atoms, which lowered the optical band gap of the material. In addition, a broad absorption hump between 450 and 650 nm was observed, which is attributed to OVs near the Cu atoms[57]. These OVs slightly distort the octahedral symmetry of the surrounding environment and facilitate d–d transitions within the Cu atoms[59]. Accordingly, the optical band gap of Cu/TiO2 was estimated to be 2.9 eV using Tauc plots
Further, the EFB of Cu/TiO2 was determined with Mott-Schottky analysis at 1 kHz. Accordingly, the CB potential (ECB) in energy diagrams for Cu/TiO2 was calculated as -0.45 V, below the ECB of the undoped TiO2 (ECB = -0.61 V), as shown in Figure 2A. The positive slopes of both plots indicate that both NPs possess n-type semiconductor characteristics, as illustrated in Figure 2B. Based on the ECB and EVB of Cu/TiO2, the photoelectrons have sufficient energy to reduce oxygen to form O2•- and the holes are sufficiently energetic to oxidize water to produce •OH[60].
Figure 2. (A) Schematic illustration of the electronic band structure; (B) The Mott–Schottky analysis of TiO2 and Cu/TiO2; (C) The photocurrent and (D) the electrochemical impedance spectra for TiO2 and Cu/TiO2. The inset shows the equivalent circuit, consisting of R1 (solution resistance), C1 (constant phase element), and R2 (CTR). CTR: Charge transfer resistance.
The photoelectric properties of Cu/TiO2
In addition to broad light absorption, efficient photocatalysts require production of abundant active charge carriers with long lifetimes, allowing for their diffusion from the bulk to the surface where they can react with oxygen or water to produce ROS. To evaluate the photoconversion efficiency, we measured the photocurrent response under visible LED light irradiation (400-800 nm). As shown in Figure 2C, the steady-state photocurrent density of Cu/TiO2 (~3 µA·cm-2) was 15 times greater than that of undoped TiO2
The CTR of Cu/TiO2 is also critical as it affects the charge transport rate. Herein, the CTR of Cu-doped and the undoped TiO2 with and without visible light irradiation was determined by EIS. As the diameter of the semi-circle in a Nyquist plot [Figure 2D] is proportional to the CTR of a sample, the result provides insights into the charge migration process. Under the same testing conditions, the CTR for Cu/TiO2 NPs is much smaller than that of the undoped TiO2 NPs, suggesting efficient electron transmission in the Cu/TiO2 due to Cu-doping. Notably, light irradiation enhanced the electron transport in both the doped and the undoped TiO2, evidenced by the smaller semi-circle diameters under irradiation than in the dark[61].
When comparing the photoelectronic properties of our Cu/TiO2 with similar nanomaterials reported in the literature [Supplementary Table 2], we observe a complex interplay among dopants (types and concentrations), structural characteristics (e.g., solid NPs, mesoporous materials, and thin films), and photoelectrical properties (e.g., bandgap, photocurrent density, and CTR). Further efforts are needed to elucidate these relationships to optimize the design and fabrication of Cu/TiO2 for improved performance in various applications.
Identification of ROS generated on Cu/TiO2 surfaces
To identify the active centers on the Cu/TiO2 surface and understand its working mechanisms regarding ROS generation under visible light irradiation [Figure 3A], the types of ROS generated were determined using EPR measurements with the spin trap 5,5’-dimethyl-1-pyrroline N-oxide (DMPO). Under visible light illumination, we observed signals from both the superoxide ions (O2•-) and hydroxyl radicals (•OH) with typical splitting patterns of 1:2:2:1 for DMPO-•OH (Figure 3B, where pure water was used as the solvent to disperse the nanocatalysts) and 1:1:1:1 for DMPO-O2•- (where methanol was used as the solvent) in
Figure 3. ROS identification and the generation mechanisms. (A) Schematic illustration of the reversible change of the shell electrons during the cycling of Cu2+ and Cu+ on the surface of Cu/TiO2 NPs; (B) The EPR spectra of radical adducts trapped by DMPO in Cu/TiO2 under visible light irradiation, where DMPO-•OH formed in aqueous dispersions and DMPO-O2•- formed in methanol dispersions; The EPR spectra of the TiO2 and Cu/TiO2 (C) under nitrogen and (D) in air. Inset in (C): the characteristic hyperfine structure of the Cu signal; (E) DO adsorbed on the excited state of Cu/TiO2 (bearing Cu+) in water; (F) The mechanism of superoxide generation via inner sphere electron transfer, including the following steps: (1) formation of charge carriers by photon absorption, whereafter Cu2+ is converted to Cu+ by trapped photoelectrons; (2) Cu+ and DO form a new sigma bond by each donating a single electron; (3) generation of intermediate (Cu2+-dioxyenyl); (4) dioxygenyl cation accepts both sigma electrons during heterolytic Cu–O bond cleavage; (5) superoxide radical ion formation; (6) superoxide radical ion diffused off of the Cu/TiO2 surface; (G) The mechanism of superoxide generation via outer sphere electron transfer, including the following steps: (1) formation of charge carriers by photon absorption, whereafter Cu2+ trapped photoelectrons; (2) photoelectrons in the Cu site react with physisorbed oxygen; (3) desorption of reduced O2 to form superoxide ions; (4) superoxide radical diffused off the Cu/TiO2 surface; (H) The mechanism of hydroxyl radical generation, including the following steps: (1) formation of charge carriers by photon absorption and homolytic Cu–O bond cleavage wherein h+ accepts one electron and the other remains on O with simultaneous nucleophilic attack on Cu by water; (2) formation of Ti-O• and Cu-OH; (3) proton transfer between Ti-O• and Cu-OH with displacement of hydroxyl radical by nucleophilic attack of Cu-O onto Ti; (5) hydroxyl radical diffuses away from the Cu/TiO2 surface. Note: In f, g, and h, a half arrow represents the transfer of one electron, while a full arrow represents a transfer of two electrons. ROS: Reactive oxygen species; NPs: nanoparticles; EPR: electron paramagnetic resonance; DMPO: 5,5-dimethyl-1-pyrroline N-oxide; DO: dissolved oxygen.
To identify the effective wavelength range of the photons that induce the ROS generation, we detected the production of O2•- and •OH using the selective probes XTT and coumarin, respectively, under a white light LED using four wavelength cut-off filters of ≥ 400, 420, 450, and 500 nm. As depicted in Supplementary Figure 10, using a light cut-off filter at 400 nm, the Cu/TiO2 NPs produced a large amount of O2•- and •OH. As the cut-off wavelength increased, there was a notable decrease in the amount of ROS formed. After the wavelength exceeded 450 nm, almost no ROS generation was observed. These results show that the Cu/TiO2 NPs can produce ROS under irradiation with visible light between 400-500 nm. Compared to undoped
ROS formation mechanisms on Cu/TiO2 surfaces
To investigate the O2•- formation via oxygen reduction on Cu/TiO2 surface, EPR spectroscopy was used to measure the transformation of critical chemical species with unpaired electrons (including Cu2+, OVs, and Ti3+) with and without irradiation, and under an air or nitrogen atmosphere. Under nitrogen gas, the
Strikingly, the signal intensity of Cu2+ decreased significantly after 10 min of irradiation, indicating the reduction of Cu2+ to Cu+ by photoelectrons and consequent loss of EPR signal. However, exposure to air restored the EPR signal intensity of Cu2+, which was attributed to the re-oxidation of surface Cu+ by oxygen, showing the reversible valence change of Cu (Cu2+ + e-
Notably, the results in Figure 3C do not support the hypothesis that O2•- formation was mediated by electrons donated from OVs or Ti3+, which might participate in other previous work[66,67]. The EPR signal for Ti3+ (g = 1.977) barely changed both during irradiation and in the dark under nitrogen, or after regeneration in air, indicating that Ti3+ did not contribute significantly to oxygen reduction[68]. The OV signal intensity
Based on the computational simulation of the oxygen adsorption process, the oxygen dissolved in water can adsorb onto Cu/TiO2 that was either in the ground state (in the dark, physisorption, Supplementary Figure 5) bearing Cu2+ or in the excited state (during visible light irradiation, physisorption followed by chemisorption, Figure 3E) bearing Cu+ on the surface. Both reaction mechanisms, i.e., outer-sphere [Figure 3F] and inner-sphere [Figure 3G] electron transfer, may contribute to O2•- formation. The outer-sphere electron transfer applies to physisorbed oxygen on the Cu/TiO2 surface (State III in Figure 3E), whereas the inner-sphere electron transfer applies to chemisorbed oxygen (State IV in Figure 3E). Importantly, in both mechanisms, (1) Cu2+ traps photoelectrons to form Cu+, which suppresses charge recombination so that the holes can migrate to Cu/TiO2 surface to participate in surface reactions to produce •OH; and (2) Cu+ donates the electron to the adsorbed oxygen to form O2•-. As a result, Cu serves as an electron storage cell that collects the photoelectrons when generated by the anatase and releases them when the electron acceptors, such as DO, are adsorbed nearby, thus maintaining a high concentration of effective charge carriers on the surface of Cu/TiO2 for interfacial redox reactions.
The •OH generation mechanism on the Cu/TiO2 surface is illustrated in Figure 3H. Herein, •OH was formed due to oxidation of the lattice oxygen, and not the adsorbed water molecule, on the surface by photogenerated holes, which will leave an OV that will be filled by an adsorbed water molecule on Cu, which has been established by abundant experimental evidence and simulations[70-74]. Relative to the bridging Ti-O-Ti structure, bridging Cu-O-Ti facilitates oxidation of the lattice oxygen, because Cu is more electron-rich than Ti and can stabilize holes in the transition state. Notably, our computational simulation indicated that when water molecules interacted with Cu atoms on Cu/TiO2 surface, hydroxide anions produced from water ionization were preferentially adsorbed to the Cu atoms on Cu/TiO2 surfaces in both excited [Supplementary Figure 7A] or ground [Supplementary Figure 7B] states.
Cu/TiO2 for water treatment
To test the efficacy of Cu/TiO2 for water treatment, we measured their waterborne dye degradation, bactericidal, and biofilm disruption ability, because organic molecules, planktonic microbes, and biofilms represent some of the most common water quality challenges. The experimental set-up for dye degradation is shown in Figure 4A. In the photodegradation experiment, RhB, an established model organic pollutant in water treatment research, was used to test the photocatalytic efficiency of Cu/TiO2 [Figure 4B][75,76]. The initial concentration was 5 mg/L, which is ~36 times higher than the maximum acceptable concentration for RhB (140 µg/L) in water[77]. For comparison, RhB degradation using undoped TiO2 was evaluated in parallel as a reference. The results showed a ~4-times increase in the photodegradation rate by the doped relative to undoped TiO2 [Figure 4B and C, Supplementary Figure 12]. The RhB photodegradation by undoped TiO2, despite its low rate, was attributed to the dye-photosensitized oxidation mechanism[76]. The pH of the samples significantly influenced the rate of RhB degradation [Supplementary Figure 13], with faster degradation observed under acidic conditions compared to basic solutions. This behavior is attributed to variations in surface charge at different pH levels. Under acidic conditions, RhB is protonated and uncharged, allowing it to be readily adsorbed onto the protonated Cu/TiO2 NPs, facilitating degradation. Conversely, under highly basic conditions (e.g., pH 11), both RhB and the Cu/TiO2 NPs carry negative charge, leading to electrostatic repulsion and hindering adsorption and subsequent degradation
Figure 4. Evaluation of Cu/TiO2 for the removal of common water pollutants: organic dye, bacteria, and biofilm. (A) Scheme of photocatalytic degradation of RhB dye using Cu/TiO2; (B) The degradation kinetics of RhB using TiO2 and Cu/TiO2; (C) The fitted first-order degradation kinetics of RhB by Cu/TiO2 under visible light irradiation; (D) The effect of various scavengers on the RhB degradation over 3 h; (E) Scheme of photocatalytic destruction of planktonic E. coli by Cu/TiO2 NPs under visible light irradiation; (F)
The Cu/TiO2 demonstrated excellent reusability because of its structural stability and consistent reactivity, which are valuable from an application and sustainability perspective. As evidenced in Supplementary Figure 15A, after five use cycles including irradiation, filtration, washing, and drying, the photocatalytic activity of Cu/TiO2 remained constant, with a ~4% decrease that was attributed to the loss of a small amount of Cu/TiO2 during rinsing. The XRD and TEM analysis of Cu/TiO2 before and after the five cycles revealed no difference in its morphology or crystal structure [Supplementary Figure 15B and C].
To identify the ROS generated by Cu/TiO2 during RhB degradation, selective ROS scavengers were introduced into the reaction system, including methanol, AO, BQ, and sodium azide (NaN3) that trap •OH, photon-induced holes, O2•-, and singlet oxygen (1O2), respectively[43,44,78]. RhB degradation was inhibited by BQ (60%), AO (55%), and methanol (40%), but not by NaN3 [Figure 4D and Supplementary Figure 16], indicating that the ROS responsible for RhB degradation included •OH, photon-induced holes, and O2•-, but not 1O2. As the precursor of •OH, the holes migrating to Cu/TiO2 surface can directly oxidize adsorbed RhB molecules[79]. Simultaneously, the charge carriers could produce ROS (O2•- and •OH) that diffused into the bulk solution to degrade free RhB. The results of the scavenging experiments were consistent with the ROS identification using EPR and ROS probes in Figure 3B and Supplementary Figure 10.
To understand the function of DO in the photocatalytic process, we compared the ROS generation and RhB photodegradation in the presence and absence of DO. Supplementary Figure 17 shows that the production of both O2•- and •OH were significantly inhibited when the DO was removed by nitrogen sparging, and accordingly RhB degradation was inhibited. As the precursor of O2•-, DO is reduced by Cu+ [Figure 3F and G], which explains the inhibition of the O2•- production in the absence of DO in water. Moreover, without DO as the final electron acceptor, the accumulation of Cu+ on Cu/TiO2 surface will scavenge the holes generated by Cu/TiO2, which leads to a decrease in •OH production. Taken together, DO not only serve as the precursor of O2•-, but also facilitates the catalytic cycling of Cu2+ and Cu+, thus promoting •OH production.
Finally, the photocatalytic bactericidal efficacy of Cu/TiO2 was evaluated. Herein, E. coli was used as a model organism because of its wide presence in contaminated water [Figure 4E]. The bacteria cells that survived after treatment by either the doped or undoped TiO2 NPs for 3 h were plated onto Tryptic Soy (TS) agar for counting. To test the toxicity of Cu/TiO2, we first evaluated the bactericidal activity in darkness. The results show negligible antibacterial activity of Cu/TiO2 in the dark, similar to undoped TiO2 NPs. However, under visible light irradiation, Cu/TiO2 exhibited excellent disinfection properties, reducing the number of bacteria over time and up to 4.3 log values after 3 h [Figure 4F and Supplementary Figure 18]. In contrast, the undoped TiO2 NPs did not display any antibacterial activity, attributed to negligible ROS production
Encouraged by the excellent bactericidal efficacy of Cu/TiO2, we further tested its ability to photodegrade established bacterial biofilms. Herein, “established biofilm” refers to biofilms that cannot be removed through simple washing steps, though they may not have reached maturity. Using the CV assay, Figure 4G-I illustrates the superior biofilm disruption (~76%) of Cu/TiO2 under visible light irradiation over 3 h relative to either irradiated undoped TiO2 NPs (~20%) or Cu/TiO2 in the dark (~20%). More biofilm was disrupted (76%, 85%, and 92%) with extended time from 3, 6, and 9 h, respectively, evidenced by both the CV assay and micrographs of the biofilms grown on glass slides. After 9 h of Cu/TiO2 treatment, only a few dispersed bacteria were observed, with no discernible biofilm structure remaining [Supplementary Figure 19].
Notably, this result is significant in water treatment research and the environmental sciences. Biofilms are often implicated in sterilization failure and infections because they exhibit complex phenotypic traits and the bacterial communities within biofilms are well-protected within a dense matrix of extracellular polymeric substances (EPSs). This makes them highly resistant to conventional antibiotic or disinfectant treatment, which significantly increases bacterial resistance to environmental stresses. Nevertheless, the large number of potent ROS generated by Cu/TiO2 completely degraded the EPS and deactivated all resident bacteria cells protected by the EPS within the biofilm. Compared to other Cu-doped or composite TiO2 materials for water treatment [Supplementary Table 4], the Cu/TiO2 reported in this study exhibited not only high dye degradation efficiency but also faster bacteria elimination and biofilm eradication under visible light irradiation.
CONCLUSIONS
ROS play a primary role in advanced oxidation/reduction processes for water treatment. Cu/TiO2 NPs efficiently produce ROS under visible-light irradiation. This work documents an atomistic understanding of the mechanisms of ROS production by Cu/TiO2. On the one hand, the isolated Cu atoms in anatase modify the electronic, photoelectric, and surface properties of TiO2, which make the anatase an excellent light absorber and photocurrent generator by narrowing the bandgap that allows for visible light excitation, improving charge separation and migration, and facilitating surface adsorption of DO; on the other hand, the discrete Cu atoms on the surface of anatase serve as the active sites for reduction of DO to form O2•- and for oxidation of lattice oxygen to form •OH. The high activity in ROS production is due to the cooperative interactions between Cu and the anatase. The potent ROS generated enabled efficient removal of organic dye, planktonic bacteria, and established biofilms, and are thus promising for off-grid water treatment applications, e.g., for fabricating point-of-use devices for remote communities that lack energy infrastructure or for treating polycyclic aromatic hydrocarbons in oil sands tailing ponds while remaining environmentally friendly and cost-effective[80].
DECLARATIONS
Authors’ contributions
Conceptualization, investigation, methodology, data processing, and writing - original draft: Yu, N.
Investigation, data processing, and visualization: Nganou, C.
Methodology and writing - original draft: Yang, D.
Investigation, visualization, writing - review and editing: Carrier, A.
Writing - review and editing, funding acquisition: Oakes, K.
Conceptualization, supervision, work administration, and writing - review and editing: Dasog, M.
Conceptualization, supervision, work administration, writing - original draft, writing - review and editing, funding acquisition: Zhang, X.
Availability of data and materials
The data and materials generated and analyzed during this study are available from the corresponding author upon reasonable request.
Financial support and sponsorship
This work was supported by the Canada Research Chairs program, New Frontiers in Research Fund - Exploration (NFRFE-2018-01005), Canada Foundation for Innovation, Research Nova Scotia, Invest Nova Scotia, Cape Breton University RISE program, and the NSERC Discovery Development Grants. The authors thank Dr. Carmen Andrei (McMaster University) and the Canadian Centre for Electron Microscopy for assistance with the TEM imaging and Andy George (Dalhousie University) and Dr. Matthew Margeson (Dalhousie University) for help with XPS analysis.
Conflicts of interest
All authors declared that there are no conflicts of interest.
Ethics approval and consent to participate
Not applicable.
Consent for publication
Not applicable.
Copyright
© The Author(s) 2025.
Supplementary Materials
REFERENCES
1. Fujishima, A.; Honda, K. Electrochemical photolysis of water at a semiconductor electrode. Nature 1972, 238, 37-8.
2. Zhang, H.; Chen, D.; Lv, X.; Wang, Y.; Chang, H.; Li, J. Energy-efficient photodegradation of azo dyes with TiO2 nanoparticles based on photoisomerization and alternate UV-visible light. Environ. Sci. Technol. 2010, 44, 1107-11.
3. Hashimoto, K.; Hiramoto, M.; Sakata, T. Temperature-independent electron-transfer: Rhodamine B/oxide semiconductor dye-sensitization system. J. Phys. Chem. 1988, 92, 4272-4.
4. Basavarajappa, P. S.; Patil, S. B.; Ganganagappa, N.; Reddy, K. R.; Raghu, A. V.; Reddy, C. V. Recent progress in metal-doped TiO2, non-metal doped/codoped TiO2 and TiO2 nanostructured hybrids for enhanced photocatalysis. Int. J. Hydrogen. Energy. 2020, 45, 7764-78.
5. Paola, A. D.; Ikeda, S.; Marcì, G.; Ohtani, B.; Palmisano, L. Transition metal doped TiO2: physical properties and photocatalytic behaviour. Int. J. Photoenergy. 2001, 3, 171-6.
6. Wang, B.; Shen, S.; Mao, S. S. Black TiO2 for solar hydrogen conversion. J. Materiomics. 2017, 3, 96-111.
7. Zhang, K.; Park, J. H. Surface localization of defects in black TiO2: enhancing photoactivity or reactivity. J. Phys. Chem. Lett. 2017, 8, 199-207.
8. Erdural, B.; Bolukbasi, U.; Karakas, G. Photocatalytic antibacterial activity of TiO2–SiO2 thin films: the effect of composition on cell adhesion and antibacterial activity. J. Photochem. Photobiol. A. Chem. 2014, 283, 29-37.
9. Ya, J.; Yang, N.; Hu, F.; Liu, Z.; E, L. Preparation and activity evaluation of TiO2/Cu-TiO2 composite catalysts. J. Sol. Gel. Sci. Technol. 2015, 73, 322-31.
10. Chen, F.; Zhao, J.; Hidaka, H. Highly selective deethylation of rhodamine B: adsorption and photooxidation pathways of the dye on the TiO2/SiO2 composite photocatalyst. Int. J. Photoenergy. 2003, 5, 209-17.
11. Liu, Z.; Sun, L.; Zhang, Q.; Teng, Z.; Sun, H.; Su, C. TiO2-supported single-atom catalysts: synthesis, structure, and application. Chem. Res. Chin. Univ. 2022, 38, 1123-38.
12. Janisch, R.; Gopal, P.; Spaldin, N. A. Transition metal-doped TiO2 and ZnO - present status of the field. J. Phys. Condens. Matter. 2005, 17, R657-89.
13. Song, H.; Li, C.; Lou, Z.; Ye, Z.; Zhu, L. Effective formation of oxygen vacancies in black TiO2 nanostructures with efficient solar-driven water splitting. ACS. Sustainable. Chem. Eng. 2017, 5, 8982-7.
14. Hejazi, S.; Killian, M. S.; Mazare, A.; Mohajernia, S. Single-atom-based catalysts for photocatalytic water splitting on TiO2 nanostructures. Catalysts 2022, 12, 905.
15. Tomboc, G. M.; Kim, T.; Jung, S.; Yoon, H. J.; Lee, K. Modulating the local coordination environment of single-atom catalysts for enhanced catalytic performance in hydrogen/oxygen evolution reaction. Small 2022, 18, e2105680.
16. Lee, B. H.; Park, S.; Kim, M.; et al. Reversible and cooperative photoactivation of single-atom Cu/TiO2 photocatalysts. Nat. Mater. 2019, 18, 620-6.
17. Wang, H.; Qi, H.; Sun, X.; et al. High quantum efficiency of hydrogen production from methanol aqueous solution with PtCu-TiO2 photocatalysts. Nat. Mater. 2023, 22, 619-26.
18. Fang, Y.; Zhang, Q.; Zhang, H.; et al. Dual activation of molecular oxygen and surface lattice oxygen in single atom Cu1/TiO2 catalyst for CO oxidation. Angew. Chem. Int. Ed. Engl. 2022, 61, e202212273.
19. Iyemperumal, S. K.; Pham, T. D.; Bauer, J.; Deskins, N. A. Quantifying support interactions and reactivity trends of single metal atom catalysts over TiO2. J. Phys. Chem. C. 2018, 122, 25274-89.
20. Qi, R.; Zhu, B.; Han, Z.; Gao, Y. High-throughput screening of stable single-atom catalysts in CO2 reduction reactions. ACS. Catal. 2022, 12, 8269-78.
21. De, S.; Dokania, A.; Ramirez, A.; Gascon, J. Advances in the design of heterogeneous catalysts and thermocatalytic processes for CO2 utilization. ACS. Catal. 2020, 10, 14147-85.
22. Hu, G.; Wu, Z.; Jiang, D. First principles insight into H2 activation and hydride species on TiO2 surfaces. J. Phys. Chem. C. 2018, 122, 20323-8.
23. Liu, P.; Zhao, Y.; Qin, R.; et al. Photochemical route for synthesizing atomically dispersed palladium catalysts. Science 2016, 352, 797-801.
24. Hu, J.; Kim, E. M.; Janik, M. J.; Alexopoulos, K. Hydrogen activation and spillover on anatase TiO2-supported Ag single-atom catalysts. J. Phys. Chem. C. 2022, 126, 7482-91.
25. Li, D.; Zhao, Y.; Miao, Y.; et al. Accelerating electron-transfer dynamics by TiO2-immobilized reversible single-atom copper for enhanced artificial photosynthesis of urea. Adv. Mater. 2022, 34, e2207793.
26. Guo, Y.; Huang, Y.; Zeng, B.; et al. Photo-thermo semi-hydrogenation of acetylene on Pd1/TiO2 single-atom catalyst. Nat. Commun. 2022, 13, 2648.
27. Kim, S. S.; Lee, H. H.; Hong, S. C. The effect of the morphological characteristics of TiO2 supports on the reverse water–gas shift reaction over Pt/TiO2 catalysts. Appl. Catal. B. Environ. 2012, 119-20, 100-8.
28. Chen, L.; Unocic, R. R.; Hoffman, A. S.; et al. Unlocking the catalytic potential of TiO2-supported Pt single atoms for the reverse water-gas shift reaction by altering their chemical environment. JACS. Au. 2021, 1, 977-86.
29. Nelson, N. C.; Chen, L.; Meira, D.; Kovarik, L.; Szanyi, J. In situ dispersion of palladium on TiO2 during reverse water–gas shift reaction: formation of atomically dispersed palladium. Angew. Chem. Int. Ed. Engl. 2020, 132, 17810-6.
30. Astruc, D.; Lu, F.; Aranzaes, J. R. Nanoparticles as recyclable catalysts: the frontier between homogeneous and heterogeneous catalysis. Angew. Chem. Int. Ed. Engl. 2005, 44, 7852-72.
31. Byrne, C.; Moran, L.; Hermosilla, D.; et al. Effect of Cu doping on the anatase-to-rutile phase transition in TiO2 photocatalysts: theory and experiments. Appl. Catal. B. Environ. 2019, 246, 266-76.
32. Cheng, C.; Fang, W. H.; Long, R.; Prezhdo, O. V. Water splitting with a single-atom Cu/TiO2 photocatalyst: atomistic origin of high efficiency and proposed enhancement by spin selection. JACS. Au. 2021, 1, 550-9.
33. Cao, Z.; Tsai, S. N.; Zuo, Y. Y. An optical method for quantitatively determining the surface free energy of micro- and nanoparticles. Anal. Chem. 2019, 91, 12819-26.
34. Chen, X.; Liu, L.; Yu, P. Y.; Mao, S. S. Increasing solar absorption for photocatalysis with black hydrogenated titanium dioxide nanocrystals. Science 2011, 331, 746-50.
35. Kim, A.; Sanchez, C.; Haye, B.; Boissière, C.; Sassoye, C.; Debecker, D. P. Mesoporous TiO2 support materials for Ru-based CO2 methanation catalysts. ACS. Appl. Nano. Mater. 2019, 2, 3220-30.
36. Martell, S. A.; Werner-Zwanziger, U.; Dasog, M. The influence of hydrofluoric acid etching processes on the photocatalytic hydrogen evolution reaction using mesoporous silicon nanoparticles. Faraday. Discuss. 2020, 222, 176-89.
37. Kirshenbaum, M. J.; Richter, M. H.; Dasog, M. Electrochemical water oxidation in acidic solution using titanium diboride (TiB2) catalyst. ChemCatChem 2019, 11, 3877-81.
38. Gelderman, K.; Lee, L.; Donne, S. W. Flat-band potential of a semiconductor: using the Mott–Schottky equation. J. Chem. Educ. 2007, 84, 685.
39. Balu, S.; Chen, Y. L.; Chen, S. W.; Yang, T. C. K. Rational synthesis of BixFe1-xVO4 heterostructures impregnated sulfur-doped
40. Kautek, W.; Gerischer, H. Photoelectrochemical reactions and formation of inversion layers at n-type MoS2-, MoSe2-, and WSe2-electrodes in aprotic solvents. Ber. Bunsenges. Phys. Chem. 1980, 84, 645-53.
41. Feenstra, R. M.; Stroscio, J. A. Tunneling spectroscopy of the GaAs(110) surface. J. Vac. Sci. Technol. B. 1987, 5, 923-9.
42. Seger, B.; Tilley, S. D.; Pedersen, T.; et al. Silicon protected with atomic layer deposited TiO2: conducting versus tunnelling through TiO2. J. Mater. Chem. A. 2013, 1, 15089.
43. Yin, M.; Li, Z.; Kou, J.; Zou, Z. Mechanism investigation of visible light-induced degradation in a heterogeneous TiO2/eosin Y/rhodamine B system. Environ. Sci. Technol. 2009, 43, 8361-6.
44. Jo, W. K.; Kim, Y. G.; Tonda, S. Hierarchical flower-like NiAl-layered double hydroxide microspheres encapsulated with black Cu-doped TiO2 nanoparticles: highly efficient visible-light-driven composite photocatalysts for environmental remediation. J. Hazard. Mater. 2018, 357, 19-29.
45. Zhang, D.; Qiu, R.; Song, L.; Eric, B.; Mo, Y.; Huang, X. Role of oxygen active species in the photocatalytic degradation of phenol using polymer sensitized TiO2 under visible light irradiation. J. Hazard. Mater. 2009, 163, 843-7.
46. Yuan, G.; Meng, Z.; Li, Y. A modified hestenes and stiefel conjugate gradient algorithm for large-scale nonsmooth minimizations and nonlinear equations. J. Optim. Theory. Appl. 2016, 168, 129-52.
47. Mato, J.; Guidez, E. B. Accuracy of the PM6 and PM7 methods on bare and thiolate-protected gold nanoclusters. J. Phys. Chem. A. 2020, 124, 2601-15.
49. Li, G.; Dimitrijevic, N. M.; Chen, L.; Rajh, T.; Gray, K. A. Role of surface/interfacial Cu2+ sites in the photocatalytic activity of coupled CuO−TiO2 nanocomposites. J. Phys. Chem. C. 2008, 112, 19040-4.
50. Moniz, S. J. A.; Tang, J. Charge transfer and photocatalytic activity in CuO/TiO2 nanoparticle heterojunctions synthesised through a rapid, one-pot, microwave solvothermal route. ChemCatChem 2015, 7, 1659-67.
51. Bhattacharyya, K.; Mane, G. P.; Rane, V.; Tripathi, A. K.; Tyagi, A. K. Selective CO2 photoreduction with Cu-doped TiO2 photocatalyst: delineating the crucial role of Cu-oxidation state and oxygen vacancies. J. Phys. Chem. C. 2021, 125, 1793-810.
52. Setvín, M.; Aschauer, U.; Scheiber, P.; et al. Reaction of O2 with subsurface oxygen vacancies on TiO2 anatase (101). Science 2013, 341, 988-91.
53. Bredow, T.; Pacchioni, G. Electronic structure of an isolated oxygen vacancy at the TiO2(110) surface. Chem. Phys. Lett. 2002, 355, 417-23.
54. Pacchioni, G. Oxygen vacancy: the invisible agent on oxide surfaces. Chemphyschem 2003, 4, 1041-7.
55. You, M.; Kim, T. G.; Sung, Y. Synthesis of Cu-doped TiO2 nanorods with various aspect ratios and dopant concentrations. Cryst. Growth. Des. 2010, 10, 983-7.
56. Kang, L.; Wang, B.; Bing, Q.; et al. Adsorption and activation of molecular oxygen over atomic copper(I/II) site on ceria. Nat. Commun. 2020, 11, 4008.
57. Colón, G.; Maicu, M.; Hidalgo, M.; Navío, J. Cu-doped TiO2 systems with improved photocatalytic activity. Appl. Catal. B. Environ. 2006, 67, 41-51.
58. Takanabe, K. Photocatalytic water splitting: quantitative approaches toward photocatalyst by design. ACS. Catal. 2017, 7, 8006-22.
59. Choudhury, B.; Dey, M.; Choudhury, A. Defect generation, d-d transition, and band gap reduction in Cu-doped TiO2 nanoparticles. Int. Nano. Lett. 2013, 3, 52.
60. Rengifo-Herrera, J. A.; Pulgarin, C. Why five decades of massive research on heterogeneous photocatalysis, especially on TiO2, has not yet driven to water disinfection and detoxification applications? Critical review of drawbacks and challenges. Chem. Eng. J. 2023, 477, 146875.
61. Kandoth, N.; Chaudhary, S. P.; Gupta, S.; et al. Multimodal biofilm inactivation using a photocatalytic bismuth perovskite-TiO2-Ru(II)polypyridyl-based multisite heterojunction. ACS. Nano. 2023, 17, 10393-406.
62. Wu, T.; Lin, T.; Zhao, J.; Hidaka, H.; Serpone, N. TiO2-assisted photodegradation of dyes. 9. Photooxidation of a squarylium cyanine dye in aqueous dispersions under visible light irradiation. Environ. Sci. Technol. 1999, 33, 1379-87.
63. Amorelli, A.; Evans, J. C.; Rowlands, C. C. Electron paramagnetic resonance study of the effect of temperature upon copper-impregnated titanium dioxide powders. J. Chem. Soc. Faraday. Trans. 1. 1989, 85, 4111.
64. Shi, X.; Lin, Y.; Huang, L.; et al. Copper catalysts in semihydrogenation of acetylene: from single atoms to nanoparticles. ACS. Catal. 2020, 10, 3495-504.
65. Zhang, Y.; Zhao, J.; Wang, H.; et al. Single-atom Cu anchored catalysts for photocatalytic renewable H2 production with a quantum efficiency of 56. Nat. Commun. 2022, 13, 58.
66. Zhao, Y.; Zhao, Y.; Shi, R.; et al. Tuning oxygen vacancies in ultrathin TiO2 nanosheets to boost photocatalytic nitrogen fixation up to 700 nm. Adv. Mater. 2019, 31, e1806482.
67. Zhao, Y.; Zhao, Y.; Waterhouse, G. I. N.; et al. Layered-double-hydroxide nanosheets as efficient visible-light-driven photocatalysts for dinitrogen fixation. Adv. Mater. 2017, 29, 1703828.
68. Dohshi, S.; Anpo, M.; Okuda, S.; Kojima, T. Effect of γ-ray Irradiation on the Wettability of TiO2 single crystals. Top. Catal. 2005, 35, 327-30.
69. Pan, C.; Shen, H.; Liu, G.; et al. CuO/TiO2 nanobelt with oxygen vacancies for visible-light-driven photocatalytic bacterial inactivation. ACS. Appl. Nano. Mater. 2022, 5, 10980-90.
70. Nakamura, R.; Nakato, Y. Molecular mechanism of water oxidation reaction at photo-irradiated TiO2 and related metal oxide surfaces. Solid. State. Phenom. 2010, 162, 1-27.
71. Nosaka, Y.; Nosaka, A. Understanding hydroxyl radical (•OH) generation processes in photocatalysis. ACS. Energy. Lett. 2016, 1, 356-9.
72. Kim, B.; Jeong, D.; Ohta, T.; Cho, J. Nucleophilic reactivity of a copper(II)-hydroperoxo complex. Commun. Chem. 2019, 2, 187.
73. Nosaka, Y.; Nosaka, A. Y. Generation and detection of reactive oxygen species in photocatalysis. Chem. Rev. 2017, 117, 11302-36.
74. Nosaka, Y.; Daimon, T.; Nosaka, A. Y.; Murakami, Y. Singlet oxygen formation in photocatalytic TiO2 aqueous suspension. Phys. Chem. Chem. Phys. 2004, 6, 2917.
75. Jakimińska, A.; Pawlicki, M.; Macyk, W. Photocatalytic transformation of Rhodamine B to Rhodamine-110 - the mechanism revisited. J. Photochem. Photobiol. A. Chem. 2022, 433, 114176.
76. Wu, T.; Liu, G.; Zhao, J.; Hidaka, H.; Serpone, N. Photoassisted degradation of dye pollutants. V. Self-photosensitized oxidative transformation of Rhodamine B under visible light irradiation in aqueous TiO2 dispersions. J. Phys. Chem. B. 1998, 102, 5845-51.
77. Skjolding, L. M.; Jørgensen, L. V.; Dyhr, K. S.; et al. Assessing the aquatic toxicity and environmental safety of tracer compounds Rhodamine B and Rhodamine WT. Water. Res. 2021, 197, 117109.
78. Jańczyk, A.; Krakowska, E.; Stochel, G.; Macyk, W. Singlet oxygen photogeneration at surface modified titanium dioxide. J. Am. Chem. Soc. 2006, 128, 15574-5.
79. Curry, D. E.; Andrea, K. A.; Carrier, A. J.; et al. Surface interaction of doxorubicin with anatase determines its photodegradation mechanism: insights into removal of waterborne pharmaceuticals by TiO2 nanoparticles. Environ. Sci. Nano. 2018, 5, 1027-35.
Cite This Article
How to Cite
Download Citation
Export Citation File:
Type of Import
Tips on Downloading Citation
Citation Manager File Format
Type of Import
Direct Import: When the Direct Import option is selected (the default state), a dialogue box will give you the option to Save or Open the downloaded citation data. Choosing Open will either launch your citation manager or give you a choice of applications with which to use the metadata. The Save option saves the file locally for later use.
Indirect Import: When the Indirect Import option is selected, the metadata is displayed and may be copied and pasted as needed.
About This Article
Special Issue
Copyright
Data & Comments
Data
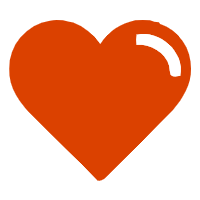
Comments
Comments must be written in English. Spam, offensive content, impersonation, and private information will not be permitted. If any comment is reported and identified as inappropriate content by OAE staff, the comment will be removed without notice. If you have any queries or need any help, please contact us at [email protected].