Strategies for enhancing room temperature gas sensing performance of stand-alone transition metal dichalcogenides
Abstract
Although the development of industry has significantly improved the living standards of human beings, it has also inevitably caused serious pollution of the atmosphere, which has greatly aroused concern about gas detection technology. In recent years, two-dimensional transition metal dichalcogenides (TMDs) have attracted great attention in the field of air sensing due to their excellent adsorption ability for harmful gases at room temperature. However, their inherent performance deficiencies have caused problems with weak sensor responsiveness and long response/recovery times. Research targeting the performance tuning of stand-alone system TMDs is necessary to radically improve the performance of sensors. This review summarizes a series of strategies that researchers have adopted in recent years to improve the performance of stand-alone TMDs materials. Firstly, the application of TMDs materials in gas sensors is described. Then, the methods and mechanisms for enhancing the gas sensing performance of stand-alone TMDs through different strategies are highlighted. Finally, the future development of TMDs gas sensors is summarized and projected.
Keywords
INTRODUCTION
Nowadays, human society has long entered the information age. While technology and industrial upgrading have helped mankind create great wealth, they have caused serious pollution and damage to the ecological environment. The emission of a series of toxic and harmful gases has brought about such serious environmental issues as greenhouse effect, acid rain, ozonosphere hole, etc. The leakage of combustible gases, including liquefied petroleum gas, natural gas, and coal gas, poses significant risks of fire, explosion, carbon monoxide poisoning, and other safety hazards due to their flammable and explosive nature. Rapid and accurate quality testing of ambient air appears to be essential. The market for gas sensors, as the primary means of air detection, has been expanding steadily over the past few years. Widespread deployment of gas sensors to build sensor networks in applications such as the Internet of Things (IoT) and Industrial Internet of Things (IIoT) is considered to be the focus of future construction to achieve green and smart development[1-4]. Therefore, the preparation of gas sensors with excellent sensing performance is a research direction of great practical significance and development prospect.
According to the different signal conversion methods, gas sensors can be categorized as electrochemical[5], optical[6], acoustic[7], resistive[8,9], and others. Among them, resistive gas sensors are widely used in important fields such as environmental monitoring, industrial production and scientific research due to their ease of operation, simple structure, low cost and ease of integration[10]. Their response process usually consists of two aspects: recognition of target gas molecules and signal conversion. The gas sensing phenomenon is mainly based on the adsorption/desorption process of gas molecules on the active layer of the gas sensor. Charge transfer occurs between the gas molecules and the surface of the sensing material, which changes the carrier concentration of the sensitive material[11,12]. Thus, the resistance of the sensor varies with the gas concentration at a specific operating temperature, leading to the detection of the gas molecule content. During the desorption of the gas molecules, the resistance of the sensor returns to the initial conditions. For now, metal oxides (MOs, such as ZnO, SnO2, TiO2, etc.) are mainly used on the market as sensitive materials for resistive gas sensors. A typical MOs gas sensor is the tubular structure illustrated in Figure 1A. Although their high responsiveness to toxic gases can be produced in a short response time[13-16], MOs must operate at high operating temperatures (> 250 °C), which not only increases power consumption of device, but also causes nanomaterials to agglomerate, shortening service life and posing safety risks[17,18]. Besides, the poorer gas selectivity and humidity stability of MOs also limit their development in the field of gas sensing.
Figure 1. Device schematics of (A) tubular MOs gas sensor and (B) planar TMDs gas sensor; Radar plot for performance evaluation of (C) MOs and (D) TMDs gas sensors. MOs: Metal oxides; TMDs: transition metal dichalcogenides.
In recent years, the unique atomic layer structure of transition metal dichalcogenides (TMDs), one of a family of two-dimensional (2D) materials, has been found to possess excellent physical and chemical properties, including high specific surface area near theoretical extremes, excellent semiconductor properties, abundant surface-active sites, and electronic properties highly susceptible to adsorption of chemical molecules[19-25]. These properties provide TMDs with significant “inherent” advantages for gas sensing applications. In particular, the ability of TMDs to work at room temperature (RT, 25 °C) with strong interactions with gas molecules not only reduces device energy consumption but also improves stability and practicality, which is a major challenge for conventional MO semiconductors. Researchers have mostly used the planar structure illustrated in Figure 1B to investigate the gas sensing performance of TMDs and demonstrated their sensitivity to numerous gas molecules such as ethanol, NO2, NH3, etc.[26-29], indicating their potential in the field of gas sensing. Moreover, a comparison with conventional MOs
In order to improve the performance index of TMDs for gas detection, researchers have experimented with many different methods. They are mainly divided into the following three categories: (i) Modification of the TMDs material itself. By promoting their adsorption properties to gas molecules and improving the electron transfer rate, the gas detection capability can be effectively enhanced; (ii) Modification of TMDs with noble metal nanoparticles. Based on the “electron sensitization” and “spillover effect”, the electrical properties of TMDs can be regulated and the activation energy required for adsorption and desorption can be effectively reduced[25]; (iii) Building heterogeneous structures with other semiconductor materials to achieve carrier redistribution in the junction region and improve the electronic structure and carrier flow, thus enhancing the gas-sensitive performance[30]. Although many articles on TMDs gas sensors have been summarized in recent years, most of them have focused on the heterostructure and composite materials of TMDs, and few systematic reviews have been conducted on the performance improvement strategies of the TMDs materials themselves. Therefore, this review focuses on the classification and summary of the modulation means for TMDs materials themselves. Firstly, the molecular structure and physical and chemical properties of TMDs are introduced, the reasons for their inadequate performance are analyzed, and the strategies to improve their own performance are summarized. Then, the performance enhancement mechanisms and implementation methods are analyzed for different strategies, and their advantages and challenges are summarized. Finally, potential solutions to these challenges and key perspectives for future research in this field are outlined.
GAS SENSING PROPERTIES OF TMDS
Properties, advantages and disadvantages of TMDs
TMDs are a huge branch in the family of 2D materials. They are usually represented by the chemical formula MX2, where M stands for transition metal elements (e.g., Ti, Zr, Nb, Mo, W, Pd, etc.) and X stands for three elements of the sulfur group (S, Se, Te). Elemental distribution and molecular structure are shown in Figure 2. The monolayer TMDs are typical sandwich atomic structures[31,32]. The transition metal M atomic layer separates the upper and lower six atomic layers of the sulfur group elements X. The atoms within the layer are connected by covalent bonds, while the layers are connected to each other by weak van der Waals forces[33,34]. Therefore, single or few-layer TMDs materials can be obtained by mechanical exfoliation or electrochemical intercalation due to this weak interlayer bonding and can also be prepared directly using methods such as CVD or hydrothermal[35-37]. Despite belonging to the same branch, the compounds composed of these elements have different electronic properties depending on the difference in the number of electrons in the unbound d orbitals and the coordination geometry of the metal atoms[38]. For example, NbTe2 and TaTe2 are metallic in nature, NbSe2 and TaS2 are superconducting in character. While materials with typical semiconductor properties, such as MoS2, WSe2, and SnS2, are excellent candidates for use as gas-sensitive sensors.
Figure 2. Elemental distribution of partial TMDs, where red elements indicate that the synthesized material is 2D TMDs and purple elements indicate that the synthesized material is partly 2D TMDs. The top of the figure shows a model of a monolayer 2H-TMDs atomic arrangement. TMDs: Transition metal dichalcogenides; 2D: two-dimensional.
The continuous spontaneous adsorption and desorption of gas molecules on the material surface makes the gas sensing mechanism a dynamic and complex process. Here, we take the adsorption (< 200 °C) of NO2 gas as an example to explain the gas response process. When the TMDs material is placed in air, a portion of O2 molecules will be preferentially adsorbed on the surface of the material and undergo charge transfer until the state is stabilized. The reaction is written as follows:
At this point, if the surface of the material comes into contact with NO2 gas molecules, the NO2 molecules will extract electrons from the adsorbed oxygen negative ions or directly capture electrons from the conduction bands of the TMDs, which will lead to an increase in the concentration of holes in the TMDs material since NO2 (electron acceptor) has a stronger electron affinity energy than O2, as given by
As we know, semiconductor materials can be classified into n-type (electron type) and p-type (hole type) due to the different types of most carriers. The decrease in electron concentration makes the conducting properties of n-type TMDs decrease, which is manifested by the decrease in device conductivity. As the concentration of NO2 gas increases, this phenomenon will further intensify until the concentration remains constant or reaches the upper limit of adsorption of the material in order to reach dynamic equilibrium. When the NO2 concentration decreases, the NO2 gas molecules leave the surface of the material and return the transferred electrons, thus restoring the sensor conductivity to its initial state. By analogy, electron acceptors can raise the resistance of n-type TMDs semiconductors and also lower the resistance of p-type TMDs semiconductors. In contrast, electron donors can lower the resistance of n-type semiconductors and also raise the resistance of p-type semiconductors.
Table 1 compares the sensing properties of traditional MOs with TMDs. The unique physicochemical properties of semiconductor TMDs as sensitive materials for gas sensors have several distinct advantages: (i) Its atoms are arranged in a 2D pattern, which gives TMDs a large specific surface area, and brings an abundance of active sites on the surface, which can fully interact with gas molecules; (ii) The electronic structure of TMDs is extremely sensitive to gas molecules and has a high adsorption energy and charge transfer degree for some gas molecules, so that they can effectively recognize the presence of low concentrations of gases[39-42]. Table 2 shows the adsorption energy and charge transfer degree of TMDs for some gases; (iii) The ability to operate at RT avoids the safety hazards and energy consumption associated with higher operating temperatures, and extends the lifetime and operating stability of the devices; (iv) TMDs have excellent mechanical properties and flexibility. Young’s modulus of several layers of MoS2 is reported to be as high as 0.33 ± 0.07 TPa, which exceeds that of stainless steel and graphene oxide[43]. It is more suitable for use in wearable flexible devices and other specialized fields.
Comparison of sensing properties of traditional MOs and TMDs
Sensitive materials | Advantages | Limitations |
MOs | Higher response Fast response/recovery speed Low preparation costs | Require high operating temperature (> 200 °C) Low carrier mobility at RT Nanomaterials are prone to agglomeration High device power consumption Poor gas selectivity |
TMDs | Relatively high carrier mobility Large surface area exposing more active sites RT workability Excellent mechanical toughness Device miniaturization and low power consumption | Slower recovery Higher preparation costs Long-term stability needs to be improved |
DFT calculations of adsorption energy and charge transfer degree of TMDs
Materials | Ea/ΔQ | O2 | CO | NH3 | H2O | NO | NO2 | H2 | Ref. |
MoS2 | Ea (eV) | -0.07 | -0.128 | -0.250 | -0.234 | -0.195 | -0.276 | -0.07 | [39] |
ΔQ (e) | 0.004 | 0.020 | -0.069 | 0.012 | 0.011 | 0.100 | 0.004 | ||
WS2 | Ea (eV) | -0.11 | -0.14 | - | -0.18 | - | - | - | [40] |
ΔQ (e) | 0.0134 | 0.0078 | - | 0.0048 | 0.0096 | - | - | ||
WSe2 | Ea (eV) | -0.0087 | -0.0092 | -0.042 | -0.045 | -0.025 | -0.067 | - | [41] |
ΔQ (e) | 0.0182 | 0.0089 | -0.0172 | 0.0186 | 0.0346 | 0.1165 | - | ||
MoTe2 | Ea (eV) | - | -0.14 | -0.24 | - | - | -0.5 | - | [42] |
ΔQ (e) | - | -0.02 | 0.03 | - | - | -0.31 | - |
Nevertheless, current studies have shown that TMDs still suffer from low response, slow speed of response, long recovery time and even incomplete recovery. These have greatly limited their application prospects in the field of gas sensing. It is mainly due to the following reasons: (i) The 2D surface of the TMDs is extremely smooth, which is not conducive to its interaction with gas molecules and makes the sensing response weak; (ii) The poor electrical conductivity and the slow carrier transport of the TMDs reduce the response and reaction speed of the devices; (iii) The diffusion of gas molecules on the surface of TMDs is slower, which may lead to longer adsorption and desorption processes, thus affecting the response and recovery speed. Besides, TMDs face the same problems as other 2D materials, such as difficulty in preparing large areas of high quality, poor air stability, and high production costs.
For these reasons, researchers have taken various approaches to optimize and develop the gas sensing performance of TMDs in an attempt to address these challenges in an effective manner. Although the introduction of new substances, such as material sensitization through noble metal nanoparticles or the construction of heterojunctions with other semiconductors, has been proven to be effective in improving the response degree and response speed. However, the sensing efficiency of TMDs can be fundamentally improved if they can be optimized for the performance defects of the materials themselves. Compounding with other materials on top of their own optimization can significantly improve the performance of gas sensors from multiple perspectives. In other words, optimizing the performance of intrinsic TMDs is crucial for advancing the performance of device over time, which has significant implications for research.
Strategies for improving gas sensing performance of stand-alone TMDs
For a range of obstacles, including the need to improve TMDs adsorption capacity, slow response rate, and incomplete recovery, researchers have recently used a variety of strategies to intervene in the intrinsic properties of materials. The main strategies are classified as [Figure 3]: morphological adjustment, crystal phase modulation and defect introduction.
Figure 3. Schematic diagram of gas sensing performance enhancement strategy for TMDs. TMDs: transition metal dichalcogenides.
Morphological adjustment
One of the major advantages of 2D materials is their large specific surface area, which permits full access to gas molecules. However, as research progresses, 2D TMDs materials with planar structures are no longer sufficient. Meanwhile, the edge sites possess higher adsorption activity compared to the 2D planar sites of TMDs. The three-dimensional (3D) structure of TMDs can be constructed by morphology adjustment, which can not only further enhance the contact area between TMDs and gas molecules and increase the number of adsorption sites, but also the 3D structure makes the edge active sites fully utilized. Therefore, the response of TMDs to gases can be effectively enhanced.
Crystal phase modulation
Fast response speed is an important metric for gas sensors. However, limitations in carrier transport rates make semiconductor TMDs have long response and recovery times. Depending on the atomic arrangement, the electrical properties of TMDs can change significantly; for example, 2H phase is a stable semiconductor phase that can interact with gas molecules, and 1T phase is a metal phase with superior electrical conductivity and carrier transport efficiency, which builds a high-speed network for electron transport. By modulating the crystal phases through specific processes, multifunctional structures can be constructed within the material and combined with the properties of each other to effectively enhance the overall gas response of the material. Constructing the TMDs material into a 1T/2H mixed-phase structure with a specific ratio not only improves its shortcoming of large RT resistance but also improves the carrier transport efficiency on the basis of ensuring the gas adsorption, thus effectively accelerating the response and recovery speed of the device.
Defect introduction
Since the 2D surfaces of pure TMDs materials are extremely smooth and do not contain any dangling bonds, they interact weakly with gas molecules, which seriously affects their adsorption effect. The unpaired electrons exhibited by the defect sites are extremely sensitive to specific gases. Therefore, more active sites can be constructed by introducing vacancy defects on the material surface. According to the study, the adsorption of gas molecules by defect sites is significantly stronger than that of planar sites, and even changes from weak physical adsorption to strong chemical adsorption. Therefore, it can significantly enhance the adsorption strength of the TMDs material on gas molecules and generate more charge transfer to enhance the device response.
Therefore, morphological adjustment, crystal phase modulation and defect introduction can enhance the gas adsorption capacity, adsorption strength, and response speed of TMDs from different angles, thus improving their gas sensing performance comprehensively. In the next chapter, the specific roles and practical applications of different strategies are analyzed in detail.
STRATEGIES FOR IMPROVING PERFORMANCE OF STAND-ALONE TMDS
Morphological adjustment
The gas sensing process is mainly manifested as an interaction process between gas molecules and sensitive materials. In this process, in addition to the physicochemical properties of the sensitive material and the gas molecules, the diffusion process and the surface chemical reaction (SCR) of the gas molecules on the material surface are also crucial. An important factor affecting this aspect is the morphology of semiconductor materials. Wang et al. constructed a model of the surface diffusion and reaction process of gas molecules through a series of experiments on layered porous SnO2 microspheres with different specific surface areas and void sizes, and deeply investigated the relationship between SCR and gas diffusion and their effects on gas sensing performance[44]. According to Knudsen diffusion theory and reaction kinetics, the rates of SCR (k) and gas diffusion (Dk) are expressed, respectively, by
where A is the pre-exponential factor, Ea is the activation energy, r is the pore radius, R is the gas constant, which is equal to 8.314 J·(mol·K)-1, T is the temperature, and M is the molecular weight. It can be seen that the effect of T on k is significantly larger than that on Dk. Combined with the experimental data, it can be concluded that in the low T range (T < 180 °C), the slow SCR is responsible for limiting the sensor response. In this case, a larger specific surface area provides more SCR and significantly improves the device response. Therefore, building a large specific surface area is a key point for the performance improvement of RT gas sensors.
On the other hand, the edge sites of TMDs have been of interest for gas sensing and photocatalytic applications[45-48]. Many research results based on density functional theory (DFT) have also demonstrated that the edge active sites are more active for gas molecules and can promote rapid adsorption and charge transfer of gases, resulting in a fast and significant electrical signal response[49-51]. Therefore, how to construct the morphological structure of the material to maximize the specific surface area and fully expose the edge active sites becomes one of the strategies to improve the gas-sensitive performance of the TMDs material itself.
In recent years, researchers have constructed TMDs materials with 3D morphology (nanorods, nanowalls, nanoflowers, etc.) using various processes, such as hydrothermal, chemical vapor deposition, and self-assembly. Table 3 summarizes the different material morphologies that researchers have tried to improve the gas sensing performance of TMDs and the performance levels achieved, including details of sensitive materials, morphological structures, target gases, detection concentrations, responses, response times, recovery times, limits of detection (LOD), and operating temperatures[48,52-61]. Although numerous studies have designed different material structures, including nanopillars, nanospheres, nanowalls and composite multistage structures, the ultimate goal is to maximize the specific surface area of the material and fully expose the active edges. This can effectively increase the active sites of the material and expand the contact space between the material and the gas molecules; combined with the strong adsorption effect of the TMDs material itself on the gas molecules, the adsorption capacity of the gas can be significantly enhanced[62,63]. Typically, for example, Cho et al. synthesized three MoS2 films with different alignment morphologies (horizontally aligned, hybrid aligned, and vertically aligned) by CVD for rapid vulcanization [Figure 4A-D] and compared the difference in their sensitivity to NO2 gas[61]. By comparison [Figure 4E], it was found that the vertically aligned MoS2 films showed about five times higher sensitivity to NO2 gas molecules compared with the horizontally aligned MoS2 films. The vertically aligned MoS2 showed excellent resistance change compared to the horizontally aligned MoS2, even with the same surface area exposed to the same concentration of gas molecules. Also, the DFT calculations show that the binding energy of the edge sites is generally lower and the adsorption distance of NO2 is shorter, verifying the positive effect of MoS2 edge site exposure on the gas sensing performance. Also using MoS2 as a sensitive material, Li et al. synthesized layered hollow MoS2 microspheres by the hydrothermal method and used them for NO2 gas detection
Figure 4. (A) Schematic diagram of the preparation of MoS2 films with different arrangements; SEM images of MoS2 films arranged in (B) horizontally aligned, (C) hybrid aligned, and (D) vertically aligned; (E) Comparison curves between the responses of MoS2 films in various arrangements to various NO2 concentrations[61]. Copyright 2015, ACS Publications; (F) Schematic diagram of the growth process for both solid and hollow hierarchical MoS2 microspheres; (G) Response curves of MoS2 spheres with different structures to different concentrations of NO2 at 150 °C[59]. Copyright 2019, Elsevier; (H) Response mechanism of WS2_Co-N-HCNCs to NO2; (I) Real-time response curves of WS2_Co-N-HCNCs to NO2 after different heat treatments; (J) Adsorption rate constants (kads) and desorption rate constants (kdes) of WS2_Co-N-HCNCs after different heat treatments[60]. Copyright 2018, John Wiley and Sons. SEM: Scanning electron microscopy.
Comprehensive performance comparison of TMDs gas sensors with various morphological structures
Material | Structure | Target gas | Concentration (ppm) | Response (%) | Response time (s) | Recovery time (s) | LOD (ppb) | Work temperature (°C) | Ref. |
MoS2 | Hierarchical hollow microspheres | NO2 | 100 | 40.3a | 79 | 225 | - | 150 | [59] |
MoS2 | Vertically aligned | NO2 | 100 | 10a | - | - | - | RT | [61] |
WS2 | Nanotriangles | NO2 | 0.8 | 55.9a | - | - | < 5 | 150 | [57] |
WS2 | Nanoflakes | NO2 | 0.8 | 26.6a | - | - | ~50 | 150 | [57] |
WS2 | 3D nanosheets | NO2 | 0.5 | 41b | - | - | - | 160 | [58] |
WS2 | Carbon nanofiber frame | NO2 | 10 | 211c | 54 | 305 | 35 | RT | [56] |
WS2 | Vertical and planar growth | NO2 | 0.5 | 22a | 600 | 1,400 | - | RT | [53] |
WS2 | Hollow carbon nanocages | NO2 | 5 | 48.2a | - | - | 100 | RT | [60] |
WS2 | Multiwall carbon nanotube skeleton | TMA | - | - | - | - | 76 | RT | [55] |
WSe2 | 3D vertical growth | NO2 | 1 | 34.6a | 66 | 1,020 | 4 | RT | [48] |
WSe2 | Nanoflower | NH3 | 40 | 24.65a | 396 | - | 2,000 | 150 | [54] |
WSe2 | Nanoflower | NO2 | 0.8 | 20.5a | 196 | - | 0.1 | 100 | [54] |
SnS2 | Vertical flakes | NO2 | 50 | 164a | 40.2 | 309 | - | 120 | [52] |
Structural design strategies are more homogeneous and reproducible for materials compared to several other strategies. Improving the conditions or using auxiliary means based on the basic synthesis methods makes it possible to achieve a certain degree of adjustment in material growth or assembly mode. However, the structural design of sensitive materials often targets multilayer TMDs or a large number of nanosheets, which will inevitably increase the contact interfaces of the materials. Despite it is investigated that van der Waals interfaces between 2D materials have superior carrier transport properties, excessive introduction of contact interfaces still leads to an increase in the internal resistance of the material, which limits the gas response[64,65]. Therefore, the tuning of the material structure also needs to be designed and controlled by the researcher.
Crystal phase modulation
The physical properties of TMDs are influenced not only by the constituent elements but also by the different coordination modes. It has been demonstrated that TMDs have several types of atomic structures, including 2H phase, 1T phase, 1T’ phase (distorted 1T), and other close distorted phases[66-69]. The 2H and 1T phases have received the most attention among them. The 2H phase is defined as a trigonal structure consisting of six sulfur or selenium atoms with intermediate transition metal atoms [Figure 5A], while the 1T phase is defined as two tetrahedra consisting of the top and bottom three sulfur or selenium atoms combined with intermediate transition metal atoms in an anti-ligand manner [Figure 5B][70,71]. Despite being composed of the same elements, the two atomic structures exhibit dramatically different electronic properties. In the case of MoS2, for example, 1T-MoS2 is in fact unstable without additional electrons injected into its S-Mo-S framework, as it tends to aggregate and then transform into stable 2H-MoS2 due to S-S van der Waals interactions[72]. In 2H-MoS2, the d orbitals split into three bands,
Figure 5. Schematic atomic structure of TMDs. (A) 2H semiconductor phase and (B) 1T metal phase. TMDs: Transition metal dichalcogenides.
Currently, TMDs phase conversion strategies are mainly applied in the directions of energy storage, catalysis, and optoelectronics[81,84,85], while only a few studies have applied them to gas sensing. Recently, Duan et al. reported a study of the phase modulation of vertical WSe2 nanosheet arrays by microwave plasma and facilitating gas sensing [Figure 6A][86,87]. Based on the original 3D structure, a 1T/2H hybrid phase structure of WSe2 was constructed by phase modulation, and Se vacancies were introduced to effectively improve its gas sensing performance [Figure 6B and C]. After only 60 s of treatment, the response of the sample to 1 ppm NO2 could reach 52.24%, and the response/recovery time were both significantly improved. The experimental results demonstrate that microwave plasma is an effective phase conversion process and can effectively enhance gas sensing performance. Chen et al. assisted the selenization of 3D WOx nanoscrews into WSe2 nanoscrews with a 1T/2H mixed-phase structure by a low-temperature plasma process and controlled their shape growth using a glancing angle deposition (GLAD) system [Figure 6D][88]. The large specific surface area of the cubic structure nanoscrews ensures sufficient contact between the sensitive material and the gas, while the 1T/2H mixed-phase structure in WSe2 reduces the potential barrier height. The prepared NO sensors exhibit the best sensing capability at a ratio of 0.41 between the 1T and 2H phases, with a response of more than 40% at 60 ppb at RT and a low LOD about 15 ppb [Figure 6E and F], which demonstrates their potential application as high-performance gas sensors. After annealing the lithium-exfoliated MoS2 monolayer nanosheets, Zong et al. found that the initial lithium-exfoliated 1T-phase MoS2 gradually transformed into 2H-phase, and the conversion ratio of the 1T and 2H phases could be modulated by this operation (the structure is shown in Figure 6G)[89]. The investigation results show that the 1T/2H hybrid phase MoS2 exhibits typical p-type semiconductor properties and significant adsorption ability for NO2 molecules. The 100 °C-annealed MoS2 with 40% 1T and 60% 2H phases exhibited the best gas sensing performance (up to 25% response to 2 ppm NO2 at RT within 10 s and LOD down to 25 ppb, shown in Figure 6H and I). This study demonstrates that a multiphase structure can improve the gas detection capability of ML-MoS2, bringing new insights into transition metal disulfide gas sensors.
Figure 6. (A) Schematic diagram of microwave plasma phase modulation; (B) TEM images of 1T/2H mixed-phase structured WSe2 nanosheets; (C) Relationship curve between 2H phase content in WSe2 nanosheets and processing time, as well as device response to
Nevertheless, the phase structure modulation strategy has some limitations. In general, the 2H/1T phase transition of TMDs can be achieved by chemical and physical methods[76,90,91]. Chemical methods, such as assisted stripping using intercalating ions (e.g., Li+ and Na+)[82,92], promote the 2H/1T phase transition by donating electrons from the corresponding atoms (Li and Na) to the van der Waals gap of 2H-TMDs and producing a stable 1T phase. However, almost all chemical methods involve rather long reaction times and the use of hazardous reagents (n-butyl lithium or Na electrodes)[93,94]. Physical methods are mainly based on strain effects and charge injection. All these methods undoubtedly cause an increase in process and time costs. On the other hand, the random nature of many phase transition processes makes it difficult to precisely control the transition ratio and the distribution of the mixed phases, and the 1T phase is a relatively unstable phase, which means that the device lifetime may be affected. In addition, the phase transition process is likely to be accompanied by the generation of defects, the effects of which will be discussed in the following subsection. Therefore, the strategy remains to be further developed and validated by researchers.
Defect introduction
With the rapid development of high-resolution characterization techniques, delicate atomic vacancies and the amazing effects they bring have attracted increasing attention from researchers. With their unique electron distribution and dense energy fluctuations, vacancy defects of various sizes have been well exploited. The presence of vacancies can potently modulate the morphology, elemental composition, lattice structure and electron distribution of a material, thus having an impact on its actual properties[95,96]. In the field of catalysis, such configurations can serve as an ideal platform to reveal the catalytic mechanism and precisely regulate the catalytic properties, becoming highly active sites or effective active promoters in various catalytic reactions[97-99]. Similarly, defect sites are also active adsorption sites for gas molecules in TMDs gas sensing. Defects significantly enhance the gas sensing performance of TMDs by altering their electronic structure and surface chemistry. Vacancy defects (e.g., missing sulfur or metal atoms) introduce unpaired electrons and localized states within the bandgap in the material, lowering the energy barrier for adsorption of gas molecules and enhancing the density of electronic states near the Fermi energy level. This modulation of the electronic structure strengthens the chemical adsorption of gas molecules to the material surface, promoting charge transfer and orbital hybridization, thereby amplifying the sensing signal. Defect sites act as highly active adsorption centers, preferentially trapping target gas molecules and optimizing the adsorption-desorption equilibrium by modulating the adsorption energy. In addition, defect engineering can be used to enhance the selective response to specific gases by adjusting the defect type (e.g., point defects, edge sites) and concentration in a targeted manner, ultimately realizing the gas sensing characteristics of high sensitivity, high selectivity, and fast response. Numerous researchers have verified its promotion of gas adsorption by means of theoretical calculations. Li et al. demonstrated by DFT calculations that monolayer MoS2 with vacancies showed higher adsorption energy and charge transfer for NO and H2O with greater resistance variation[100]. Ma et al. performed a theoretical analysis of the adsorption effect of various gas molecules on Se vacancies in WSe2[101]. The results show that H2O and N2 molecules are readily physically adsorbed on S vacancies, while CO and NO tend to undergo chemisorption. On the other hand, if temperatures are higher, NO2 and N2O molecules may engage in dissociative chemisorption on the S vacancy, resulting in the formation of O-doped WSe2 and fully filling the faulty energy level the S vacancy had previously caused. Cui et al. looked into the effects of CO, NH3, NO, and NO2 adsorption in intrinsic and defective WS2[102]. While the W vacancy showed a larger enhancement in the adsorption energy of NO with NO2, the S vacancy demonstrated an improvement in the adsorption energy for all four gases. The band gap of the flawed WS is also strongly influenced by the adsorption of all four gases. Apparently, vacancies can considerably improve charge transfer and adsorption intensity, which effectively improves responsiveness.
In addition to theoretical calculations, many experimental-based research results have reported the enhancement of defects on the performance of gas sensors. Qin et al. obtained 1-3 layers with rich S defects in SnS2 nanosheets using a chemical exfoliation method and prepared NH3 gas sensors [Figure 7A-C][103]. Although the bulk SnS2 showed insensitivity to NH3, the 2D SnS2 nanosheets exhibited a fast response. The response to 500 ppm NH3 at RT was 420% with a response time of only 16 s. The response time of 500 ppm NH3 at RT was only 16 s. Such a fast response is attributed to the high-energy S vacancies generated on the SnS2 surface during the exfoliation process that effectively enhance the gas adsorption strength. Recently, Qin et al. obtained WS2 nanosheets within three layers and rich in S defects using chemical exfoliation and vacuum filtration, and prepared NH3 sensors on flexible paper substrates [Figure 7D][104]. The exfoliated WS2 containing both W and S defects was demonstrated by the fitted peak analysis of X-ray photoelectron spectroscopy spectra (XPS), as shown in Figure 7E. RT NH3 gas sensitivity results show that the response intensity of 2D WS2 with abundant S defects is almost three times higher than that of highly crystalline 2D WS2, and can respond to device bending and gas introduction simultaneously.
Figure 7. (A) Diagrammatic representation of the few-layer 2D SnS2 exfoliation and transfer process; (B) 2D SnS2 response-recovery curves for a range of NH3 concentrations; (C) Morphological structure and elemental ratios of bulk and exfoliated SnS2[103]. Copyright 2018, Elsevier; (D) Bendable sensors prepared by exfoliated WS2 and their response curves to NH3 and respiration; (E) XPS curves of W and S of exfoliated WS2, demonstrating the presence of W and S defects[104]. Copyright 2022, Elsevier. 2D: Two-dimensional; XPS: X-ray photoelectron spectroscopy spectra.
Visibly, proper defect engineering can significantly enhance the adsorption strength of TMDs to gases and promote the occurrence of charge movement. Notably, however, the elevated gas adsorption energy not only promotes the spontaneous adsorption of gas molecules onto the material surface, but also implies that the desorption process requires higher energy. This would certainly exacerbate the problem of a slow desorption process. At the same time, the incomplete coordination of atoms due to surface defects can also reduce the air stability of the material and have an influence on its service life.
SUMMARY AND PERSPECTIVES
The performance requirements for gas sensors have always been strong responsiveness, quick sensitivity, high selectivity, low LOD, long service life, and low power consumption[105-107]. Research on gas sensing using TMDs has been going on for more than two decades. Researchers have also developed various forms of performance improvements in TMDs by integrating existing technologies and developing new opportunities. Among them, the study of stand-alone TMDs for achieving high-performance gas sensing is highly desirable and indeed necessary. It also provides a guiding experience and a solid foundation for the synthesis and regulation of subsequent composites.
Unfortunately, despite a plethora of new and inspiring design concepts to improve the gas sensing performance of TMDs, they are currently in the laboratory stage and are not available to replace traditional MOs for the time being. Up to now, compared to MOs, the commercialization of TMDs-based gas sensors still faces many challenges: (i) the chemical stability of TMDs materials is insufficient, and they are prone to oxidation or degradation when working in humid or oxygen-containing environments for a long period of time, leading to performance degradation; (ii) the large-area homogeneous film-forming process for TMDs still needs to be developed, which has higher requirements for raw materials and equipment, and is currently difficult to realize low-cost mass production; (iii) the gas response and response/recovery speeds of TMDs still need to be improved in comparison with those of commercialized metal-oxide gas sensors. As mentioned above, no performance enhancement strategy is “perfect”. On the one hand, the gas adsorption strength and device responsiveness of TMDs can be steadily improved by morphology modulation, phase structure conversion and defect introduction. However, unlike other sensors, gas sensors cannot isolate the sensitive material from the outside world through traditional encapsulation structures[108]. The introduction of interface and defect elements at this point adds fuel to the fire for the accuracy and stability of device detection. On the other hand, there is a certain instability in the process of phase transition. If the transition region and ratio need to be precisely controlled, it has extremely high requirements for the precision of the equipment, which also hinders its application in actual production. Therefore, it is difficult to determine which strategy has the most significant effect on the enhancement of gas sensing performance of TMDs. Additionally, it is necessary for researchers to optimize the material according to its characteristics and select the most suitable strategy to maximize the device performance.
In order to realize the transition of TMDs gas sensing from the laboratory to the market, a comprehensive strategy must be developed to integrate advanced manufacturing, characterization and modeling techniques, and encapsulation design to rationally design TMDs sensitive material systems to achieve the combined requirements of high response, fast response/recovery, and a long lifetime. These points are discussed separately below [Figure 8].
Figure 8. Schematic of development needs for high-performance TMDs RT gas sensors. TMDs: Transition metal dichalcogenides; RT: room temperature.
(1) Advanced manufacturing technology is an important requirement in almost all fields of micro- and nano-processing. And the morphology design and stable realization of TMDs sensitive materials put higher requirements on processing and manufacturing methods. In this case, 3D printing technology has great application prospects. Numerous reports of 3D printing-based sensors have also verified the effectiveness of their precise control of material morphology and device structure. However, TMDs-based 3D printing inks are not yet well developed, and only a few applications in energy, catalysis, and optoelectronic sensing have been reported[109,110]. It is also a research priority to improve the printing accuracy, which enables more complex structures to be obtained accurately. In addition, the proximity-field nanopatterning (PnP) technology enables the preparation of ordered nanostructures in a shorter time than 3D printing. The process is also highly compatible and can be seamlessly integrated with other device fabrication processes. The 3D nanostructures prepared by PnP technology have a uniform network structure and a large surface area, which is favorable for chemical sensor applications. Therefore, it can be further developed as one of the powerful alternatives to the traditional morphological adjustment process.
(2) Advanced characterization and modeling technology. The detection mechanism of TMDs for gas molecules is derived to some extent from the mechanistic model of MOs, which remains to be explored in depth. Advanced in-situ characterization methods, such as in-situ Raman and in-situ infrared spectroscopy, can visually identify intermediate states in the adsorption and resolution processes. These methods can then be used in conjunction with scientific modeling to reduce carrier migration, energy band states, resistance changes, etc., in adsorption systems to give researchers a clear understanding of exactly what is happening in the reaction process. This is crucial for enhancing the performance of the sensing system.
(3) The encapsulation design. Due to the unique properties of gas sensors, it is challenging to completely isolate sensitive materials, which has a significant impact on the responsiveness, selectivity, and stability of devices. Especially in high humidity environments, the resistance and responsiveness of gas sensors is generally significantly affected[111,112], which will not be conducive to the universal application of sensors. The advanced encapsulation technology can not only isolate the molecules such as H2O and O2 in the air, but also effectively shield other interfering gas molecules, which can significantly improve the gas selectivity and device stability. This is essential for practical applications.
Overall, this paper reviews strategies to enhance gas sensing performance for stand-alone TMDs systems. It will provide reasonable inspiration for researchers working in the fields of gas sensing, 2D material properties, and semiconductor material processing. It is hoped that this review will generate research interest and attention for gas sensing applications of TMDs.
DECLARATIONS
Authors’ contributions
Writing-original draft: Duan, Y.; Qiu, J.
Validation, review and editing: Feng, S.; Zhao, H.; Lei, Y.
Availability of data and materials
Not applicable.
Financial support and sponsorship
The authors acknowledge support from the German Research Foundation (DFG, Project number 501766751) and the Sino-German Centre for Research Promotion (GZ1579). Duan, Y. and Qiu, J. appreciate support from the China Scholarship Council (Nos. 202206990027 and 201908530218).
Conflicts of interest
All authors declared that there are no conflicts of interest.
Ethical approval and consent to participate
Not applicable.
Consent for publication
Not applicable.
Copyright
© The Author(s) 2025.
REFERENCES
1. Renzone, G. D.; Landi, E.; Mugnaini, M.; Parri, L.; Peruzzi, G.; Pozzebon, A. Assessment of LoRaWAN transmission systems under temperature and humidity, gas, and vibration aging effects within IIoT contexts. IEEE. Trans. Instrum. Meas. 2022, 71, 1-11.
2. Filipovic, L.; Selberherr, S. Application of two-dimensional materials towards CMOS-integrated gas sensors. Nanomaterials 2022, 12, 3651.
3. Lombardi, A.; Grassi, M.; Malcovati, P.; et al. A CMOS integrated interface circuit for metal-oxide gas sensors. Sens. Actuators. B. 2009, 142, 82-9.
4. Malcovati, P.; Grassi, M.; Baschirotto, A. Towards high-dynamic range CMOS integrated interface circuits for gas sensors. Sens. Actuators. B. 2013, 179, 301-12.
5. Jiang, G.; Goledzinowski, M.; Comeau, F. J. E.; et al. Free-standing functionalized graphene oxide solid electrolytes in electrochemical gas sensors. Adv. Funct. Mater. 2016, 26, 1729-36.
6. Xie, S.; Lu, Y.; Zhang, S.; Wang, L.; Zhang, X. Electro-optical gas sensor based on a planar light-emitting electrochemical cell microarray. Small 2010, 6, 1897-9.
7. Zhao, H.; Fan, S.; Chen, Y.; et al. Oxygen plasma-treated graphene oxide surface functionalization for sensitivity enhancement of thin-film piezoelectric acoustic gas sensors. ACS. Appl. Mater. Interfaces. 2017, 9, 40774-81.
8. Kwon, B.; Bae, H.; Lee, H.; et al. Ultrasensitive N-channel graphene gas sensors by nondestructive molecular doping. ACS. Nano. 2022, 16, 2176-87.
9. Zhang, C.; Xu, K.; Liu, K.; Xu, J.; Zheng, Z. Metal oxide resistive sensors for carbon dioxide detection. Coord. Chem. Rev. 2022, 472, 214758.
10. Majhi, S. M.; Mirzaei, A.; Kim, H. W.; Kim, S. S.; Kim, T. W. Recent advances in energy-saving chemiresistive gas sensors: a review. Nano. Energy. 2021, 79, 105369.
11. Degler, D.; Weimar, U.; Barsan, N. Current understanding of the fundamental mechanisms of doped and loaded semiconducting metal-oxide-based gas sensing materials. ACS. Sens. 2019, 4, 2228-49.
12. Kim, H.; Lee, J. Highly sensitive and selective gas sensors using p-type oxide semiconductors: overview. Sens. Actuators. B. 2014, 192, 607-27.
13. Fang, X.; Zong, B.; Mao, S. Metal-organic framework-based sensors for environmental contaminant sensing. Nanomicro. Lett. 2018, 10, 64.
14. Hu, X.; Yu, J.; Gong, J.; Li, Q.; Li, G. α-Fe2O3 nanorings prepared by a microwave-assisted hydrothermal process and their sensing properties. Adv. Mater. 2007, 19, 2324-9.
15. Xu, S.; Xu, Y.; Zhao, H.; Xu, R.; Lei, Y. Sensitive gas-sensing by creating adsorption active sites: coating an SnO2 layer on triangle arrays. ACS. Appl. Mater. Interfaces. 2018, 10, 29092-9.
16. Xu, S.; Zhao, H.; Xu, Y.; Xu, R.; Lei, Y. Carrier mobility-dominated gas sensing: a room-temperature gas-sensing mode for SnO2 nanorod array sensors. ACS. Appl. Mater. Interfaces. 2018, 10, 13895-902.
17. Jo, Y. M.; Jo, Y. K.; Lee, J. H.; Jang, H. W.; Hwang, I. S.; Yoo, D. J. MOF-based chemiresistive gas sensors: toward new functionalities. Adv. Mater. 2023, 35, e2206842.
18. Xie, J.; Liu, X.; Jing, S.; Pang, C.; Liu, Q.; Zhang, J. Chemical and electronic modulation via atomic layer deposition of NiO on porous In2O3 films to boost NO2 detection. ACS. Appl. Mater. Interfaces. 2021, 13, 39621-32.
19. Fu, Q.; Han, J.; Wang, X.; et al. 2D transition metal dichalcogenides: design, modulation, and challenges in electrocatalysis. Adv. Mater. 2021, 33, e1907818.
20. Wang, L.; Xu, D.; Jiang, L.; et al. Transition metal dichalcogenides for sensing and oncotherapy: status, challenges, and perspective. Adv. Funct. Mater. 2021, 31, 2004408.
21. Wang, L.; Liu, D.; Jiang, L.; et al. Advanced 2D–2D heterostructures of transition metal dichalcogenides and nitrogen-rich nitrides for solar water generation. Nano. Energy. 2022, 98, 107192.
22. Wu, X.; Zhang, H.; Zhang, J.; Lou, X. W. D. Recent advances on transition metal dichalcogenides for electrochemical energy conversion. Adv. Mater. 2021, 33, e2008376.
23. Xiao, Y.; Xiong, C.; Chen, M. M.; Wang, S.; Fu, L.; Zhang, X. Structure modulation of two-dimensional transition metal chalcogenides: recent advances in methodology, mechanism and applications. Chem. Soc. Rev. 2023, 52, 1215-72.
24. Zhao, B.; Shen, D.; Zhang, Z.; et al. 2D metallic transition-metal dichalcogenides: structures, synthesis, properties, and applications. Adv. Funct. Mater. 2021, 31, 2105132.
25. Lee, E.; Yoon, Y. S.; Kim, D. J. Two-dimensional transition metal dichalcogenides and metal oxide hybrids for gas sensing. ACS. Sens. 2018, 3, 2045-60.
26. Annanouch, F. E.; Alagh, A.; Umek, P.; Casanova-Chafer, J.; Bittencourt, C.; Llobet, E. Controlled growth of 3D assemblies of edge enriched multilayer MoS2 nanosheets for dually selective NH3 and NO2 gas sensors. J. Mater. Chem. C. 2022, 10, 11027-39.
27. Singh, S.; Sharma, S. Temperature dependent selective detection of ethanol and methanol using MoS2/TiO2 composite. Sens. Actuators. B. 2022, 350, 130798.
28. Tang, S. Y.; Yang, C. C.; Su, T. Y.; et al. Design of core-shell quantum dots-3D WS2 nanowall hybrid nanostructures with high-performance bifunctional sensing applications. ACS. Nano. 2020, 14, 12668-78.
29. Zhou, Q.; Song, H.; Sun, T.; Zhang, L.; Lv, Y. Cataluminescence on 2D WS2 nanosheets surface for H2S sensing. Sens. Actuators. B. 2022, 353, 131111.
30. Zheng, W.; Liu, X.; Xie, J.; Lu, G.; Zhang, J. Emerging van der Waals junctions based on TMDs materials for advanced gas sensors. Coord. Chem. Rev. 2021, 447, 214151.
31. Jariwala, D.; Sangwan, V. K.; Lauhon, L. J.; Marks, T. J.; Hersam, M. C. Emerging device applications for semiconducting two-dimensional transition metal dichalcogenides. ACS. Nano. 2014, 8, 1102-20.
32. Prabhu, P.; Jose, V.; Lee, J. Design strategies for development of TMD-based heterostructures in electrochemical energy systems. Matter 2020, 2, 526-53.
33. Radisavljevic, B.; Radenovic, A.; Brivio, J.; Giacometti, V.; Kis, A. Single-layer MoS2 transistors. Nat. Nanotechnol. 2011, 6, 147-50.
34. Wang, H.; Chen, J.; Lin, Y.; et al. Electronic modulation of non-van der Waals 2D electrocatalysts for efficient energy conversion. Adv. Mater. 2021, 33, 2008422.
35. Zhang, Y.; Yao, Y.; Sendeku, M. G.; et al. Recent progress in CVD growth of 2D transition metal dichalcogenides and related heterostructures. Adv. Mater. 2019, 31, e1901694.
36. Huang, Y.; Pan, Y. H.; Yang, R.; et al. Universal mechanical exfoliation of large-area 2D crystals. Nat. Commun. 2020, 11, 2453.
37. Xing, L.; Li, X.; Wu, Z.; et al. 3D hierarchical local heterojunction of MoS2/FeS2 for enhanced microwave absorption. Chem. Eng. J. 2020, 379, 122241.
38. Schmidt, H.; Giustiniano, F.; Eda, G. Electronic transport properties of transition metal dichalcogenide field-effect devices: surface and interface effects. Chem. Soc. Rev. 2015, 44, 7715-36.
39. Yue, Q.; Shao, Z.; Chang, S.; Li, J. Adsorption of gas molecules on monolayer MoS2 and effect of applied electric field. Nanoscale. Res. Lett. 2013, 8, 425.
40. Bui, V. Q.; Pham, T. T.; Le, D. A.; Thi, C. M.; Le, H. M. A first-principles investigation of various gas (CO, H2O, NO, and O2) absorptions on a WS2 monolayer: stability and electronic properties. J. Phys. Condens. Matter. 2015, 27, 305005.
41. Wang, T.; Zhao, R.; Zhao, X.; An, Y.; Dai, X.; Xia, C. Tunable donor and acceptor impurity states in a WSe2 monolayer by adsorption of common gas molecules. RSC. Adv. 2016, 6, 82793-800.
42. Lin, L.; Feng, Z.; Dong, Z.; Hu, C.; Han, L.; Tao, H. DFT study on the adsorption of CO, NO2, SO2 and NH3 by Te vacancy and metal atom doped MoTe2 monolayers. Physica. E. 2023, 145, 115489.
43. Castellanos-Gomez, A.; Poot, M.; Steele, G. A.; van der Zant, H. S.; Agraït, N.; Rubio-Bollinger, G. Elastic properties of freely suspended MoS2 nanosheets. Adv. Mater. 2012, 24, 772-5.
44. Wang, X.; Wang, Y.; Tian, F.; et al. From the surface reaction control to gas-diffusion control: the synthesis of gierarchical porous SnO2 microspheres and their gas-sensing mechanism. J. Phys. Chem. C. 2015, 119, 15963-76.
45. Zhao, Y.; Chang, K.; Gu, Q.; et al. Noble metal-free 2D 1T-MoS2 edge sites boosting selective hydrogenation of maleic anhydride. ACS. Catal. 2022, 12, 8986-94.
46. Wang, Z.; Li, Q.; Xu, H.; et al. Controllable etching of MoS2 basal planes for enhanced hydrogen evolution through the formation of active edge sites. Nano. Energy. 2018, 49, 634-43.
47. Xu, T.; Liu, Y.; Pei, Y.; et al. The ultra-high NO2 response of ultra-thin WS2 nanosheets synthesized by hydrothermal and calcination processes. Sens. Actuators. B. 2018, 259, 789-96.
48. Duan, Y.; Feng, S.; Zhang, K.; Qiu, J.; Zhang, S. Vertical few-layer WSe2 nanosheets for NO2 sensing. ACS. Appl. Nano. Mater. 2021, 4, 12043-50.
49. Hu, B.; Wang, B.; Bai, Z.; et al. Regulating MoS2 edge site for photocatalytic nitrogen fixation: a theoretical and experimental study. Chem. Eng. J. 2022, 442, 136211.
50. Zhuang, M.; Xu, G.; Gan, L.; et al. Sub-5 nm edge-rich 1T’-ReSe2 as bifunctional materials for hydrogen evolution and sodium-ion storage. Nano. Energy. 2019, 58, 660-8.
51. Zavala-Sanchez, L.; Khalil, I.; Oliviero, L.; Paul, J.; Maugé, F. Structure and quantification of edge sites of WS2/Al2O3 catalysts coupling IR/CO spectroscopy and DFT calculations. ChemCatChem 2020, 12, 2066-76.
52. Kumar, A.; Sharma, N.; Gutal, A. P.; et al. Growth and NO2 gas sensing mechanisms of vertically aligned 2D SnS2 flakes by CVD: experimental and DFT studies. Sens. Actuators. B. 2022, 353, 131078.
53. Bisht, P.; Kumar, A.; Ghosh, A.; et al. Tailoring the vertical and planar growth of 2D WS2 thin films using pulsed laser deposition for enhanced gas sensing properties. ACS. Appl. Mater. Interfaces. 2022, 14, 36789-800.
54. Alagh, A.; Annanouch, F. E.; Sierra-Castillo, A.; Haye, E.; Colomer, J. F.; Llobet, E. Three-dimensional assemblies of edge-enriched WSe2 nanoflowers for selectively detecting ammonia or nitrogen dioxide. ACS. Appl. Mater. Interfaces. 2022, 14, 54946-60.
55. Zhou, Q.; Zhu, L.; Zheng, C.; Wang, J. Nanoporous functionalized WS2/MWCNTs nanocomposite for trimethylamine detection based on quartz crystal microbalance gas sensor. ACS. Appl. Mater. Interfaces. 2021, 13, 41339-50.
56. Xu, Y.; Xie, J.; Zhang, Y.; et al. Edge-enriched WS2 nanosheets on carbon nanofibers boosts NO2 detection at room temperature. J. Hazard. Mater. 2021, 411, 125120.
57. Alagh, A.; Annanouch, F. E.; Umek, P.; et al. CVD growth of self-assembled 2D and 1D WS2 nanomaterials for the ultrasensitive detection of NO2. Sens. Actuators. B. 2021, 326, 128813.
58. Liu, D.; Tang, Z.; Zhang, Z. Comparative study on NO2 and H2S sensing mechanisms of gas sensors based on WS2 nanosheets. Sens. Actuators. B. 2020, 303, 127114.
59. Li, Y.; Song, Z.; Li, Y.; et al. Hierarchical hollow MoS2 microspheres as materials for conductometric NO2 gas sensors. Sens. Actuators. B. 2019, 282, 259-67.
60. Koo, W. T.; Cha, J. H.; Jung, J. W.; et al. Few-layered WS2 nanoplates confined in Co, N-doped hollow carbon nanocages: abundant WS2 edges for highly sensitive gas sensors. Adv. Funct. Mater. 2018, 28, 1802575.
61. Cho, S. Y.; Kim, S. J.; Lee, Y.; et al. Highly enhanced gas adsorption properties in vertically aligned MoS2 layers. ACS. Nano. 2015, 9, 9314-21.
62. Cho, D.; Lee, G.; Lee, Y. L.; et al. Ultrathin copper monosulfide films for an optically semitransparent, highly selective ammonia chemosensor. ACS. Appl. Mater. Interfaces. 2024, 16, 60530-40.
63. Kim, Y. B.; Jung, S. H.; Kim, D. S.; et al. Progressive NO2 sensors with rapid alarm and persistent memory-type responses for wide-range sensing using antimony triselenide nanoflakes. Adv. Funct. Mater. 2021, 31, 2102439.
64. Hu, Z.; Liu, X.; Hernández-Martínez, P. L.; et al. Interfacial charge and energy transfer in van der Waals heterojunctions. InfoMat 2022, 4, e12290.
65. Choi, M. S.; Ali, N.; Ngo, T. D.; et al. Recent progress in 1D contacts for 2D-material-based devices. Adv. Mater. 2022, 34, 2202408.
66. Chung, Y.; Yang, C.; Lee, J.; Wu, G. H.; Wu, J. M. Coupling effect of piezo–flexocatalytic hydrogen evolution with hybrid 1T- and 2H-phase few-layered MoSe2 nanosheets. Adv. Energy. Mater. 2020, 10, 2002082.
67. Liu, L.; Wu, J.; Wu, L.; et al. Phase-selective synthesis of 1T’ MoS2 monolayers and heterophase bilayers. Nat. Mater. 2018, 17, 1108-14.
68. Sokolikova, M. S.; Mattevi, C. Direct synthesis of metastable phases of 2D transition metal dichalcogenides. Chem. Soc. Rev. 2020, 49, 3952-80.
69. Yoo, Y.; DeGregorio, Z. P.; Su, Y.; Koester, S. J.; Johns, J. E. In-plane 2H-1T’ MoTe2 homojunctions synthesized by flux-controlled phase engineering. Adv. Mater. 2017, 29, 1605461.
70. Voiry, D.; Mohite, A.; Chhowalla, M. Phase engineering of transition metal dichalcogenides. Chem. Soc. Rev. 2015, 44, 2702-12.
71. Wang, Q. H.; Kalantar-Zadeh, K.; Kis, A.; Coleman, J. N.; Strano, M. S. Electronics and optoelectronics of two-dimensional transition metal dichalcogenides. Nat. Nanotechnol. 2012, 7, 699-712.
72. Wang, L.; Liu, X.; Luo, J.; et al. Self-optimization of the active site of molybdenum disulfide by an irreversible phase transition during photocatalytic hydrogen evolution. Angew. Chem. Int. Ed. Engl. 2017, 56, 7610-4.
73. Eda, G.; Fujita, T.; Yamaguchi, H.; Voiry, D.; Chen, M.; Chhowalla, M. Coherent atomic and electronic heterostructures of single-layer MoS2. ACS. Nano. 2012, 6, 7311-7.
74. Gan, X.; Zhao, H.; Quan, X. Two-dimensional MoS2: a promising building block for biosensors. Biosens. Bioelectron. 2017, 89, 56-71.
75. Mak, K. F.; Lee, C.; Hone, J.; Shan, J.; Heinz, T. F. Atomically thin MoS2: a new direct-gap semiconductor. Phys. Rev. Lett. 2010, 105, 136805.
76. Gao, G.; Jiao, Y.; Ma, F.; Jiao, Y.; Waclawik, E.; Du, A. Charge mediated semiconducting-to-metallic phase transition in molybdenum disulfide monolayer and hydrogen evolution reaction in new 1T’ phase. J. Phys. Chem. C. 2015, 119, 13124-8.
77. Huang, H.; Cui, Y.; Li, Q.; et al. Metallic 1T phase MoS2 nanosheets for high-performance thermoelectric energy harvesting. Nano. Energy. 2016, 26, 172-9.
78. Wang, D.; Zhang, X.; Bao, S.; Zhang, Z.; Fei, H.; Wu, Z. Phase engineering of a multiphasic 1T/2H MoS2 catalyst for highly efficient hydrogen evolution. J. Mater. Chem. A. 2017, 5, 2681-8.
79. Kappera, R.; Voiry, D.; Yalcin, S. E.; et al. Phase-engineered low-resistance contacts for ultrathin MoS2 transistors. Nat. Mater. 2014, 13, 1128-34.
80. Acerce, M.; Voiry, D.; Chhowalla, M. Metallic 1T phase MoS2 nanosheets as supercapacitor electrode materials. Nat. Nanotechnol. 2015, 10, 313-8.
81. Deng, Q.; Li, X.; Si, H.; et al. Strong band bowing effects and distinctive optoelectronic properties of 2H and 1T’ phase-tunable MoxRe1-xS2 alloys. Adv. Funct. Mater. 2020, 30, 2003264.
82. Lai, Z.; Yao, Y.; Li, S.; et al. Salt-assisted 2H-to-1T’ phase transformation of transition metal dichalcogenides. Adv. Mater. 2022, 34, e2201194.
83. Yu, Y.; Nam, G. H.; He, Q.; et al. High phase-purity 1T’-MoS2- and 1T’-MoSe2-layered crystals. Nat. Chem. 2018, 10, 638-43.
84. He, H.; Zhang, H.; Huang, D.; et al. Harnessing plasma-assisted doping engineering to stabilize metallic phase MoSe2 for fast and durable sodium-ion storage. Adv. Mater. 2022, 34, e2200397.
85. Wang, S.; Zhang, D.; Li, B.; et al. Ultrastable in-plane 1T–2H MoS2 heterostructures for enhanced hydrogen evolution reaction. Adv. Energy. Mater. 2018, 8, 1801345.
86. Duan, Y.; Zhang, S.; Yu, Y.; Qiu, J.; Feng, S. Facile microwave plasma driven 3D-WSe2 2H-1T phase modulation for improving NO2 gas sensing performance. Sens. Actuators. B. 2023, 387, 133822.
87. Duan, Y.; Sun, D.; Zhang, S.; Wang, S.; Qiu, J.; Feng, S. Multi-strategy coordination enables WSe2 to achieve high-performance real-world detection of NO2. Sens. Actuators. B. 2024, 403, 135183.
88. Chen, Y.; Lee, S.; Su, T.; Wu, S.; Chen, P.; Chueh, Y. Phase-modulated 3D-hierarchical 1T/2H WSe2 nanoscrews by a plasma-assisted selenization process as high performance NO gas sensors with a ppb-level detection limit. J. Mater. Chem. A. 2019, 7, 22314-22.
89. Zong, B.; Li, Q.; Chen, X.; et al. Highly enhanced gas sensing performance using a 1T/2H Heterophase MoS2 field-effect transistor at room temperature. ACS. Appl. Mater. Interfaces. 2020, 12, 50610-8.
90. Park, S.; Kim, C.; Park, S. O.; et al. Phase engineering of transition metal dichalcogenides with unprecedentedly high phase purity, stability, and scalability via molten-metal-assisted intercalation. Adv. Mater. 2020, 32, e2001889.
91. Zhu, J.; Wang, Z.; Yu, H.; et al. Argon plasma induced phase transition in monolayer MoS2. J. Am. Chem. Soc. 2017, 139, 10216-9.
92. Liu, F.; Zou, Y.; Tang, X.; et al. Phase engineering and alkali cation stabilization for 1T molybdenum dichalcogenides monolayers. Adv. Funct. Mater. 2022, 32, 2204601.
93. Chhowalla, M.; Shin, H. S.; Eda, G.; Li, L. J.; Loh, K. P.; Zhang, H. The chemistry of two-dimensional layered transition metal dichalcogenide nanosheets. Nat. Chem. 2013, 5, 263-75.
94. Tan, Y.; Liu, P.; Chen, L.; et al. Monolayer MoS2 films supported by 3D nanoporous metals for high-efficiency electrocatalytic hydrogen production. Adv. Mater. 2014, 26, 8023-8.
95. Liu, Y.; Xiao, C.; Li, Z.; Xie, Y. Vacancy engineering for tuning electron and phonon structures of two-dimensional materials. Adv. Energy. Mater. 2016, 6, 1600436.
96. Yuan, H.; Aljneibi, S. A. A. A.; Yuan, J.; et al. ZnO nanosheets abundant in oxygen vacancies derived from metal-organic frameworks for ppb-level gas sensing. Adv. Mater. 2019, 31, 1807161.
97. Lu, Q.; Yu, Y.; Ma, Q.; Chen, B.; Zhang, H. 2D transition-metal-dichalcogenide-nanosheet-based composites for photocatalytic and electrocatalytic hydrogen evolution reactions. Adv. Mater. 2016, 28, 1917-33.
98. Jia, Y.; Jiang, K.; Wang, H.; Yao, X. The role of defect sites in nanomaterials for electrocatalytic energy conversion. Chem 2019, 5, 1371-97.
99. Wang, X.; Wu, J.; Zhang, Y.; et al. Vacancy defects in 2D transition metal dichalcogenide electrocatalysts: from aggregated to atomic configuration. Adv. Mater. 2023, 35, e2206576.
100. Li, F.; Shi, C. NO-sensing performance of vacancy defective monolayer MoS2 predicted by density function theory. Appl. Surf. Sci. 2018, 434, 294-306.
101. Ma, D.; Ma, B.; Lu, Z.; et al. Interaction between H2O, N2, CO, NO, NO2 and N2O molecules and a defective WSe2 monolayer. Phys. Chem. Chem. Phys. 2017, 19, 26022-33.
102. Cui, Z.; Yang, K.; Shen, Y.; et al. Toxic gas molecules adsorbed on intrinsic and defective WS2: gas sensing and detection. Appl. Surf. Sci. 2023, 613, 155978.
103. Qin, Z.; Xu, K.; Yue, H.; et al. Enhanced room-temperature NH3 gas sensing by 2D SnS2 with sulfur vacancies synthesized by chemical exfoliation. Sens. Actuators. B. 2018, 262, 771-9.
104. Qin, Z.; Song, X.; Wang, J.; et al. Development of flexible paper substrate sensor based on 2D WS2 with S defects for room-temperature NH3 gas sensing. Appl. Surf. Sci. 2022, 573, 151535.
105. Mao, S.; Chang, J.; Pu, H.; et al. Two-dimensional nanomaterial-based field-effect transistors for chemical and biological sensing. Chem. Soc. Rev. 2017, 46, 6872-904.
106. Meng, Z.; Stolz, R. M.; Mendecki, L.; Mirica, K. A. Electrically-transduced chemical sensors based on two-dimensional nanomaterials. Chem. Rev. 2019, 119, 478-598.
107. Minh, T. N.; Thai, D. L.; Hwang, B. U.; et al. High-performance schottky diode gas sensor based on the heterojunction of three-dimensional nanohybrids of reduced graphene oxide-vertical ZnO nanorods on an AlGaN/GaN layer. ACS. Appl. Mater. Interfaces. 2017, 9, 30722-32.
108. Jeong, S. Y.; Kim, J. S.; Lee, J. H. Rational design of semiconductor-based chemiresistors and their libraries for next-generation artificial olfaction. Adv. Mater. 2020, 32, e2002075.
109. Ghosh, K.; Pumera, M. MXene and MoS3-x coated 3D-printed hybrid electrode for solid-state asymmetric supercapacitor. Small. Methods. 2021, 5, e2100451.
110. Thakkar, H.; Eastman, S.; Al-Naddaf, Q.; Rownaghi, A. A.; Rezaei, F. 3D-printed metal-organic framework monoliths for gas adsorption processes. ACS. Appl. Mater. Interfaces. 2017, 9, 35908-16.
111. Liu, C.; Duan, Z.; Zhang, B.; et al. Local Gaussian process regression with small sample data for temperature and humidity compensation of polyaniline-cerium dioxide NH3 sensor. Sens. Actuators. B. 2023, 378, 133113.
Cite This Article
How to Cite
Download Citation
Export Citation File:
Type of Import
Tips on Downloading Citation
Citation Manager File Format
Type of Import
Direct Import: When the Direct Import option is selected (the default state), a dialogue box will give you the option to Save or Open the downloaded citation data. Choosing Open will either launch your citation manager or give you a choice of applications with which to use the metadata. The Save option saves the file locally for later use.
Indirect Import: When the Indirect Import option is selected, the metadata is displayed and may be copied and pasted as needed.
About This Article
Copyright
Data & Comments
Data
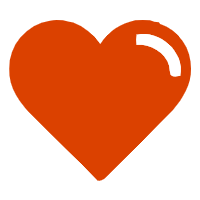
Comments
Comments must be written in English. Spam, offensive content, impersonation, and private information will not be permitted. If any comment is reported and identified as inappropriate content by OAE staff, the comment will be removed without notice. If you have any queries or need any help, please contact us at [email protected].