Research progress of photoelectrochemical conversion of CO2 to C2+ products
Abstract
The reduction of CO2 to C2+ products using photoelectrochemistry (PEC) is significant and highly challenging. However, systematic summaries on PEC CO2 conversion into C2+ products are lacking. Therefore, this paper systematically reviews the current research status of the PEC CO2 conversion for the preparation of C2+ products, including the pathways of C2+ products, the usage of catalysts and reactors, and methods for improving C2+ product selectivity. Besides, the deficiencies in current research are analyzed, and future developments are discussed.
Keywords
INTRODUCTION
The hazards of climate change to humans and the environment necessitate transitioning from our current fossil fuel economy to an economy based on renewable and carbon-neutral energy technologies. The urgency to reduce primary greenhouse gas (CO2) emissions while continuing to provide fuel for the growing global population remains one of the most significant technological challenges of our time. Photoelectrochemical technology not only maximizes the advantages of photocatalysis and electrocatalysis, but their synergy also sparks a different kind of brilliance. Utilizing photoelectrochemistry (PEC) technology to convert CO2 into fuel represents a new, clean, and green approach to using CO2[1-3], which is increasingly attracting the interest of researchers [Figure 1]. Similar to other types of catalysis, the PEC conversion of CO2 also involves multiple-step processes, resulting in a wide range of products such as C1 (CO, CH4, HCOOH, etc.) and C2+ products (C2H4, C2H6, CH3COOH, etc.). Currently, most reported CO2 conversion products are C1 products because the generation of C2+ products involves a C-C coupling process, which is more difficult to obtain than C1 products. But, compared with C1 products, C2+ products have higher added value, and research on directly converting CO2 into C2+ products holds significant significance. To date, several review articles have covered the overall research status on PEC CO2 conversion[1-7], the influence of cocatalysts[8-10], the design of the PEC cathodes[11-15], the impact of PEC parameters[16,17], and the study of catalysts[18-20]. However, no individual summaries have been on converting CO2 into C2+ products using the PEC technology. Hence, firstly, this paper aims to briefly introduce the basic principles of CO2 conversion into C2+ products. Secondly, it summarizes the catalysts and reactor types reported thus far for obtaining C2+ products. Thirdly, it emphasizes the design strategies of catalysts currently available for generating or enhancing C2+ selectivity. Finally, it concludes with a summary of the current work and prospects for future research.
FUNDAMENTAL OF PHOTOELECTROCHEMICAL CO2 REDUCTION
The PEC reduction of CO2 involves light absorption, charge carrier transfer, CO2 adsorption and activation, surface reactions, and product desorption. The energy input for PEC catalysis is provided by light and electricity. Light plays a role in generating high-energy photoelectrons for CO2 reduction, generating photoelectric voltage to reduce energy consumption to some extent and lower economic costs, and producing heat to promote the progress of the reaction. Electricity plays a role in introducing an additional bias voltage to accelerate charge carrier separation and increase local charge density. By adjusting the bias voltage, the CO2 reduction pathway can be altered to enhance selectivity.
Additionally, the supplementation of voltage can enable semiconductor materials that were originally not viable for photocatalytic CO2 conversion to become feasible [Figure 1]. The synergistic effects of light and electricity can effectively reduce the activation energy for CO2 reduction, but the study of the photoelectric synergistic effect is still lacking[21,22]. Furthermore, the contribution and distinction between photogenerated and voltage-provided electrons have not yet been reported.
Based on current reports, the mechanism for converting CO2 into C2+ involves first converting CO2 into *CO intermediates, followed by the generation of various intermediates of C2+ products through C-C coupling and multi-proton-coupled electron transfer (PCET), as shown by the equations for different pathways (Route 1-6)[23]. However, the competitive adsorption of *Had and *CO on monocomponent catalysts hinders the enhancement of PCET and C-C reaction kinetics. In order to break the linear scaling relationship between the adsorption of *Had/*CO intermediates and improve water dissociation on auxiliary sites or CO2-to-CO production, tandem catalysts consisting of multicomponents have been developed. These catalysts can achieve higher coverage of *Had and *CO, which is more conducive to the generation of C2+ products. However, to our knowledge, while there are detailed reports on achieving C2+ products through serial pathways in the electrocatalytic conversion of CO2[24], their application in the field of photoelectrocatalytic CO2 conversion has not been observed.
Route 1:
Route 2:
Route 3:
Route 4:
Route 5:
Route 6:
CATALYSTS AND REACTORS OF PHOTOELECTROCHEMICAL CO2 UPGRADING TO C2+
Hitherto, catalyst research for the PEC conversion of CO2 into C2+ products is focused on photocathodes, primarily using Cu-based catalysts[21,24-29] [Table 1]. Cu-based species have a high binding affinity for *CO intermediates, making them active sites for CO2 reduction and the generation of C2+ chemical species. However, the high energy barriers associated with C-C coupling and PCET processes still pose challenges for Cu-based catalysts regarding high overpotential and low selectivity towards C2+ products. In addition to Cu-based catalysts, reasonable structural design of some non-Cu-based catalysts enables higher selectivity towards C2+ products. Table 2 lists the selectivity of some non-Cu-based catalysts in photoelectrocatalytic CO2 conversion. It shows that the non-Cu-based catalysts are mainly composite materials, including TiO2, ZnO, WO3, etc., with more negative potentials compared to Cu-based catalysts. Regardless of Cu-based or non-Cu-based catalysts, ethanol, acetic acid, and ethylene are the main C2+ products; the yield of C2+ products is relatively low (typically at the μmol level); the Faradaic efficiencies cover a wide range, and the faradaic efficiency for a single C2+ product can reach up to 80%. The selectivity of C2+ products is primarily enhanced by adjusting the synthesis process of materials, doping with heteroatoms, and compositing with other metals/metal oxides/non-metallic materials to alter the morphology, band structure, and local electron density of the electrode materials.
Selected Cu-based catalyst for photoelectrochemical conversion of CO2 to C2+
Photoelectrode | Electrolyte | Light source1111 | Potential | C2+ products | Ref. |
Cu@porphyrin-COFs | KHCO3 (0.5 M) | 300 W Xenon lamp (200 mW·cm-2) | -1.0 V vs. SCE | Ethanol + acetic acid + acetone (~27 μmol·h-1·cm-2) | [30] |
Zn0.2:Co1@Cu | KHCO3 (0.1 M), Eosin Y (0.01 M) | AM 1.5G (200 mW·cm-2) | -0.4 V vs. SCE | Paraffin (325 μg·h-1) | [31] |
CuBi2O4/TiO2-NTs | NaHCO3 (0.1 M) | 250 W Xenon lamp (200 mW·cm-2) | -0.6 V vs. SCE | Ethanol (1.32 μmol·h-1) (FE 73%) | [32] |
6 wt% Re-doped CuO/ TiO2-NTs | NaHCO3 (0.1 M) | 300 W Xenon lamp | -0.5 V vs. SCE | Ethanol (1.9 μmol·h-1) | [33] |
CZTS/CdS | KHCO3 (0.1 M) | AM 1.5G (100 mW·cm-2) | -0.4 V vs. RHE | Ethanol (~2.5 μmol·h-1·cm-2) | [34] |
TiO2-NT/GNR/Cu/Pd-Pt | Na2SO4 (0.1 M) | 150 W Xenon lamp | -0.7 V vs. Ag/AgCl | Ethanol (4.3 mmol·g-1·h-1·cm-2) | [35] |
GO/CuxO/Cu-BTC | KHCO3 (0.1 M), Na2SO4 (0.1 M) | AM 1.5G (100 mW·cm-2) | -0.5 V vs. Ag/AgCl | Ethanol (40.5 μmol·h-1·cm-2) | [36] |
Cu | KOH (1 M) | UV LED lights (365 nm, 100 mW·cm-2) | -1.8 V vs. Ag/AgCl | Ethylene (4.7 μmol·s-1·m-2) (FE 46.6%) | [37] |
CuNx/CuO | KHCO3 (0.1 M) | AM 1.5G (100 mW·cm-2) | 0.2V vs. RHE | Ethanol (13.7 μmol·h-1·cm-2) (FE 15.2%) | [38] |
CuO-CuFeO2 | H2O: triethanolamine (volume ratio of 9:1) | 300 W Xenon lamp (100 mW·cm-2) | -0.6 V vs. Ag/AgCl | Ethanol (FE 66.73%) | [39] |
Cu/TiO2/p-Si | CsHCO3 (0.1 M) | 300 W Xenon lamp, AM 1.5G (100 mW·cm-2) | -0.9 V vs. RHE | Ethylene (FE ~23%) | [40] |
The selected non-Cu-based catalyst for photoelectrochemical conversion of CO2 to C2+
Photoelectrode | Electrolyte111 | Light source | Potential | C2+ products | Ref. |
Ti/TiO2NT-ZIF-8 | Ascorbic acid (0.2 M), sodium sulfate (0.1 M) | 125 W high pressure mercury vapor lamp | 0.1 V vs. Ag/ AgCl (Eapp) | Ethanol (10.5 mmol·L-1) | [41] |
CsPbBr3/graphite (C) | KHCO3 (0.1 M) | - | -0.8 V vs. Ag/AgCl | Oxalic acid (3.44 × 10-5 mmol) (FE 44%) | [42] |
Dye/Pd/N-TiO2 | KHCO3 (0.1 M), Eosin Y (1 mM) | 300 W Xenon lamp (200 mW·cm-2) | -1.0 V | Ethanol (26.8 μmol·h-1·cm-2) (FE 28.4%) | [43] |
ZnO/Ni-30 | KHCO3 (0.1 M), Eosin Y (1 mM) | 300 W Xenon lamp (100 mW·cm-2) | -0.6 V | Ethanol + acetic acid (12.5 μmol·h-1·cm-2) (FE 100%) | [44] |
Pd-TiO2@ZnO | KHCO3 (0.1 M), Eosin Y (1 mM) | 300 W Xenon lamp (200 mW·cm-2) | -1.0 V | Ethanol + acetic acid (~30 μmol·h-1·cm-2) | [45] |
Bi2WO6/BiOCl | KHCO3 (0.1 M), Eosin Y (1 mM) | 300 W Xenon lamp | -1.0 V | Ethanol (11.4 μmol·h-1·cm-2) (FE 80%) | [46] |
S-TiO2@GS | KHCO3 (0.1 M), Eosin Y (1 mM) | 300 W Xenon lamp (200 mW·cm-2) | -0.9 V vs. SCE | Ethanol (20.7 μmol·h-1·cm-2) | [47] |
P-WO3 | KHCO3 (0.1 M), Eosin Y (1 mM) | 300 W Xenon lamp (200 mW·cm-2) | -0.8 V vs. SCE | Acetic acid (4.7 μmol·L-1·h-1·cm-2) | [48] |
CdS/VS-MoS2-PS | KHCO3 (0.1 M), Na2SO3 (0.1 M), Eosin Y (1 mM) | 200 mW·cm-2 | -0.9 V vs. SCE | Ethanol + ethylene glycol (350 μmol·h-1·cm-2) (FE 67%) | [49] |
Pd@TiO2/Ti3CN | KHCO3 (0.1 M) | 300 W Xenon lamp | -0.8 V | Ethanol (14 μmol·h-1·cm-2) | [50] |
Pd/R-TiO2/TiN-30 | KHCO3 (0.1 M), Eosin Y (1 mM) | 300 W Xenon lamp (200 mW·cm-2) | -0.8 V vs. SCE | Ethanol + acetic acid + glycolaldehyde (39.41 μmol·L-1·h-1·cm-2) (FE 65.69%) | [51] |
NiMoO4/ZnO-3 | Na2SO3 (0.1 M) | Xenon lamp (100 mW·cm-2) | -0.8 V vs. SCE | Ethanol + acetic acid + glycolaldehyde + ethylene glycol (29.2 μmol·h-1·cm-2) (FE 72.6%) | [52] |
Si@WO3-NS | KHCO3 (0.1 M) | 300 W Xenon lamp (100 mW·cm-2) | -1.0 V vs. SCE | Acetone + ethanol + acetic acid + glycolaldehyde (~43 μmol·h-1·cm-2) (FE 62.7%) | [53] |
In addition to catalysts, the selection of reactors also significantly influences the generation of C2+ products. The light source, membrane characteristics, electrolyte, manufacturing materials, wall thickness, operational modes (batch or flow), heat exchange, phase requirements (gas-solid, liquid-solid, or gas-liquid-solid), CO2 solubility, and flow characteristics of feed gases and electrolytes can affect catalytic performance[54,55]. Currently, reactors used for CO2 PEC conversion are primarily modified from electrochemical CO2 reduction reactors and can be divided into H-type and flow electrolysis cells [Figure 2][56-58]. The H-type electrolysis cell is a relatively simple device that belongs to a batch reactor. It separates the anode and cathode using ion exchange membranes. However, its significant Ohmic resistance between the electrodes and limited CO2 solubility have hindered its development. The low current density (< 100 mA·cm-2) achieved in H-type cells does not meet industrial requirements. Furthermore, alkaline electrolytes are more conducive to suppressing H2 reduction and enhancing C-C coupling. However, alkaline electrolytes are not feasible in aqueous CO2 reduction conducted in H-type electrolysis cells. Nevertheless, the gas diffusion electrode (GDE) of the flow electrolysis cell separates the CO2 flow field from the liquid electrolyte, allowing the use of high-concentration alkaline electrolytes.
Figure 2. PEC reactor for CO2 conversion (A) H-type cell (batch reactor)[54]; (B) flow cell (continuous reactor). PEC: Photoelectrochemistry.
The flow electrolysis cell is a continuous operation reactor where gases and electrolytes can be continuously supplied to the electrolytic cell. GDEs are used as the electrodes, which greatly overcome the limitations of CO2 solubility. However, a certain distance between the anode and cathode still exists, resulting in significant Ohmic resistance. The membrane-electrode reactor integrates the anode, cathode, and membrane assembly, significantly reducing the distance between the electrodes and exhibiting improved catalytic performance. However, it lacks a reference electrode, making it challenging to obtain accurate working electrode potentials. Although membrane-electrode reactors have found wide applications in electrochemical CO2 reduction, there are few reports on their use in photoelectrochemical CO2 conversion[37]. In addition, the state-of-the-art electrocatalytic CO2 reduction performance achieved from flow electrolysis cells and membrane-electrode electrolyzers can almost produce CO and formic acid at industrial current densities. However, producing single high-value C2+ products, such as ethylene and ethanol, remains challenging. As for photoelectrochemical CO2 reduction, the current densities used in the electrolytic cells have a significant gap compared to industrial current densities. It is worth mentioning that most of the recent research employs self-designed, assembled, or customized CO2 photoelectrochemical reactors. No universally recognized standard exists for the configuration and operation of CO2 electrolyzers. Different studies use distinct reactor structures, components, and catalysts, making it difficult to directly compare results and select optimal cathodic catalysts.
STRATEGIES TO IMPROVE THE SELECTIVITY OF C2+ PRODUCTS
The photoelectrochemical conversion of CO2 into C2+ products is highly challenging. The entire process involves multiple aspects: absorption of light to obtain electron-hole pairs, followed by charge separation and transfer to the surface of the photocathode. At the same time, CO2 adsorption is necessary for reducing CO2 to form C1 radicals and intermediates, and the stability of intermediates and C-C coupling is crucial. Moreover, the production of C2+ products requires more electrons compared to C1 products. These processes indicate that improving light absorption, enhancing CO2 adsorption capacity, increasing charge separation and transfer efficiency, and constructing suitable active sites are essential for obtaining high-content C2+ products. In addition, the reactor configuration, operating conditions, and electrode assembly also significantly influence catalytic performance. A continuous flow PEC reactor can produce more C2-C3 products compared to batch reactors[58], and selecting an appropriate voltage is beneficial for the generation of C2+ products[17]; appropriate electrode assembly can increase CO2 adsorption, suppress hydrogen production, and facilitate C2+ product generation[40]. However, there is a lack of research on the impact of reactor configuration, operating conditions, and electrode assembly on the obtained C2+ products. Besides, the current yield of C2+ products from CO2 conversion via photoelectrocatalysis is generally low (often below 50 µmol·cm-2·h-1), with correspondingly low current densities (below 10 mA·cm-2); many studies do not report CO2 conversion rates or product yields[25]. According to the reaction process, conversion rates and yields can be enhanced by adjusting reactor and reaction parameters (such as applying pressure) and modifying the properties of the photoelectrode. Specifically, improving light absorption capacity (through morphology control and elemental doping, etc.), enhancing charge carrier separation (via auxiliary metal addition and defect or heterojunction construction, etc.), and promoting surface reactions (by depositing a thin bilayer of Sustainion/Nafion, etc.) can contribute to these enhancements[4]. Therefore, this section mainly summarizes the research progress in improving the selectivity of C2+ products from four aspects: electrode material morphology design, adjustment of band structure, construction of heterojunctions, and design of active sites.
Morphology
The morphology significantly influences the selectivity of CO2 PEC reduction products [Figure 3]. Current research suggests that C2+ products can be acquired by constructing specific spatial structures. Wang et al. found that three-dimensional (3D) nanorod-shaped zinc oxide exhibited the optimal spatial environment compared to amorphous block-, mushroom-, and ball cactus-like structures, facilitating C-C coupling to produce C2 products (ethanol and acetic acid)[44]. Furthermore, their research group discovered that porous PEC based on a 3D hierarchical structure of covalent organic frameworks (COFs) could effectively enhance CO2 adsorption and the rate of C-C coupling to generate C2+ products[30]. Hollow catalysts exhibited different selectivity on the inner and outer surfaces due to the increased collision probability of intermediates within the closed cavity, achieving a high selectivity of C2+ products (67.0%) on the inner surface[49]. In addition, by simulating the morphology of thylakoids in plant cells, the Si@WO3-nanosheet (NS) catalyst with a 2D-3D structure is advantageous for achieving C-C coupling[53].
Figure 3. (A) SEM image of 3D nanorod ZnO[44]. Copyright 2018 Springer; (B) COFs-based porous photoelectrochemical with 3D hierarchical structure for CO2 reduction[30]. Copyright 2020 Elsevier; (C) TEM image of CdS/VS-MoS2 and (D) catalytic performance of CdS/VS-MoS2-PS[49]. Copyright 2023 Elsevier. SEM: Scanning electron microscopy; 3D: three-dimensional; COFs: covalent organic frameworks; TEM: transmission electron microscopy.
Band structure
The band structure of electrode materials significantly influences light utilization and the types of CO2 reduction products. A narrower band gap is beneficial for broadening the absorption range of light. In contrast, adjustable conduction band positions facilitate the control of product selectivity [Figure 4]. The position of the conduction band is related to the reduction capability of the catalyst, playing a crucial role in the photoelectrocatalytic reduction of CO2 and serving as a thermodynamic factor influencing the availability of C2+ products. Adjusting the conduction band to the appropriate position is beneficial for improving the acquisition of certain C2+ products. Han et al. achieved improved selectivity of CO2 reduction products by depositing metals such as Pd, Pt, Ni, Au, etc., to modulate the conduction band potentials of the TiO2@ZnO/FTO[45]. Besides doping with metals, doping with non-metals can alter the conduction band position. Yu et al. designed and prepared a novel P-doped WO3 catalyst where P doping elevated the conduction band position of the WO3 electrode from -0.13 to -0.54 V [V vs. normal hydrogen electrode (NHE)], achieving an acetic acid yield of 4.7 μmol/(L·h·cm2) at a conduction band position of -0.38 V (V vs. NHE)[48].
Figure 4. (A) Band structure of samples; (B) CO2 PEC performance of the samples[45]. Copyright 2018 Elsevier. PEC: Photoelectrochemistry.
Heterojunction
The built-in electric field of heterojunctions facilitates carrier separation, accelerates charge transfer, and enhances the velocity of intermediates, resulting in a high probability of C-C coupling and, consequently, higher C2+ product yields[31,53] [Figure 5]. Lu et al. constructed 0D/1D CuFeO2/CuO nanowire heterojunction arrays. They achieved a higher Faradaic efficiency (66.73%) for ethanol production at -0.6 V vs. Ag/AgCl, mainly attributed to the rapid separation of photogenerated electrons and holes in the heterojunction material and the transfer of electrons from CuFeO2 to CuO, enabling highly content of C2+ products (ethanol)[39]. Xu et al. fabricated 2D TiO2/Ti3CN MXene heterojunctions, where the Pd@TOCN-200 photoelectrode exhibited the highest ethanol production rate (14 µM·cm-2·h-1) due to the enhanced CO2 adsorption and favorable C-C coupling environment provided by the 2D TiO2/Ti3CN heterojunction structure[50].
Figure 5. (A) Band structure of CuO-CuFeO2; (B) CO2 PEC performance of samples at -1.0 V vs. Ag/AgCl[39]. Copyright 2023 Elsevier; (C) Band structure of Pd@TOCN; (D) CO2 PEC performance of samples[50]. Copyright 2023 Elsevier; (E) Band structure of Si@WO3 and (F) CO2 PEC performance of samples[53]. Copyright 2023 Elsevier. PEC: Photoelectrochemistry.
Active site
The active site is a critical part of catalytic reactions and directly determines the distribution of products. They change product distribution by affecting the adsorption strength of products and intermediates and promote the coupling of *CO with *CO by adjusting the local electron density gradient, facilitating C-C coupling, and enhancing the acquisition of C2+ products. In the PEC CO2 reduction reaction, active sites composed of multimetallic species[31,33,35,36,52], oxygen vacancies/sulfur vacancies[34,51], certain specific functional groups (such as adjacent imine groups)[47], and metal-nonmetal junctions[38] are favorable for CO2 adsorption and C-C coupling, promoting the generation of C2+ products [Figure 6]. Among them, Lu et al. doped Re into CuO and combined it with Ti nanotubes. Under solar light and -0.5 V vs. saturated calomel electrode (SCE) conditions, the product was almost entirely ethanol (1.9 µM·h-1). The above results were attributed to high-valence rhenium as an active site for hydrogenation reactions. Its doping promoted the alcoholization process and facilitated C-C coupling[33]. Zhou et al. adjusted the surface sulfur vacancies of Cu2ZnSnS4 (CZTS)/CdS by annealing in different atmospheres, which were active sites for CO adsorption and followed C-C coupling. By rational tuning, they achieved higher ethanol selectivity[34]. In addition, Wang et al. introduced N into CuO to obtain Cu-N active components. These components possessed asymmetric d-p orbitals, which could reduce the activation energy of C-C coupling and induce local charge redistribution, thereby improving the material’s conductivity. They achieved a 15.2% C2 product selectivity in a water solution at 0.2 V vs. reversible hydrogen electrode (RHE)[38].
Figure 6. (A) Schematic mechanism of Re-doped CuO/TiO2-NTs for CO2 reduction[33]. Copyright 2021 Elsevier; (B) The scheme for product generation of TiO2-NT/GNR-metal electrodes[35]. Copyright 2021 Elsevier; (C) The corresponding CO2 conversion yields of CZTS/CdS (HA) and CZTS/CdS (HN)[34]. Copyright 2021 Wiley; (D) CO2 PEC performance of Pd/R-TiO2/TiN-x[51]. Copyright 2023 Elsevier; (E) Schematic mechanism of EY+S-TiO2@GS for CO2 reduction[47]. Copyright 2020 Elsevier; and (F) Schematic mechanism of CuNx/CuO for the CO2 PEC reduction[38]. Copyright 2022 Elsevier. PEC: Photoelectrochemistry.
CONCLUSION AND PROSPECTS
Currently, research on PEC conversion of CO2 to C2+ products primarily focuses on developing photoelectrodes. The catalysts used are mainly Cu-based, and the major C2+ products obtained are ethanol, acetic acid, and ethylene. The involved reactors are predominantly flow reactors. Adjusting the catalyst morphology, band structure, and active sites can improve light absorption, enhance charge carrier transfer, strengthen CO2/CO* adsorption, and provide a favorable C-C coupling environment, achieving high selectivity for C2+ products. Although some progress has been made in the research on PEC CO2 conversion to C2+ products, there is currently limited reporting on products containing three or more carbon atoms. The types of catalysts used are also limited, and their yields are relatively low (typically at the level of μmol). The reactors used in studies are mostly self-designed, assembled, or customized. There is no recognized standard for the configuration and operation of CO2 reactors, and it is difficult to selectively optimize cathode catalysts by directly comparing different research reports. The current density employed in electrolytic cells differs significantly from industrial-scale requirements, making industrialization challenging. The present reaction pathway is not yet clear, and there is still insufficient research on the specific reaction mechanisms involved in catalysts. Additionally, research on photoelectric synergies is lacking. Therefore, increasing efforts in reactor development and establishing design standards, exploring and developing cost-effective and high-performing catalysts, and conducting in-depth research to enhance catalytic performance and understand reaction mechanisms are the main directions for future investigations.
DECLARATIONS
Authors’ contributions
Prepared and revised the manuscript: Jiang X, Chen R
Designed and revised the manuscript: Chen YX, Lu CZ
All authors contributed to the discussion and preparation of the manuscript.
Availability of data and materials
Not applicable.
Financial support and sponsorship
Authors are thankful for the financial support of the Natural Science Foundation of Fujian Province (2023H0046), the XMIREM autonomously deployment project (2023CX10, 2023GG01), the National Natural Science Foundation of China (22275185), the Major Research Project of Xiamen (3502Z20191015), and the Fujian Science & Technology Innovation Laboratory for Optoelectronic Information of China (2021ZR132, 2021ZZ115).
Conflicts of interest
Chen YX is the Junior Editorial Board Member of Chemical Synthesis , while the other authors have declared that they have no conflicts of interest.
Ethical approval and consent to participate
Not applicable.
Consent for publication
Not applicable.
Copyright
© The Author(s) 2024.
REFERENCES
1. Zhou W, Guo JK, Shen S, et al. Progress in photoelectrocatalytic reduction of carbon dioxide. Acta Phys Chim Sin 2020;36:1906048.
2. Tang B, Xiao FX. An overview of solar-driven photoelectrochemical CO2 Conversion to chemical fuels. ACS Catal 2022;12:9023-57.
3. Xu S, Shen Q, Zheng J, et al. Advances in biomimetic photoelectrocatalytic reduction of carbon dioxide (Adv. Sci. 31/2022). Adv Sci 2022;9:2270196.
4. Chen P, Zhang Y, Zhou Y, Dong F. Photoelectrocatalytic carbon dioxide reduction: fundamental, advances and challenges. Nano Mater Sci 2021;3:344-67.
5. Zhang N, Long R, Gao C, Xiong Y. Recent progress on advanced design for photoelectrochemical reduction of CO2 to fuels. Sci China Mater 2018;61:771-805.
6. Ochedi FO, Liu D, Yu J, Hussain A, Liu Y. Photocatalytic, electrocatalytic and photoelectrocatalytic conversion of carbon dioxide: a review. Environ Chem Lett 2021;19:941-67.
7. Kan M, Wang Q, Hao S, et al. System engineering enhances photoelectrochemical CO2 reduction. J Phys Chem C 2022;126:1689-700.
8. Chang X, Wang T, Yang P, Zhang G, Gong J. The development of cocatalysts for photoelectrochemical CO2 reduction. Adv Mater 2019;31:e1804710.
9. Dutta S, Patil R, Chongdar S, Bhaumik A. Dehydrogenase-functionalized interfaced materials in electroenzymatic and photoelectroenzymatic CO2 reduction. ACS Sustain Chem Eng 2022;10:6141-56.
10. Li Y, Li S, Huang H. Metal-enhanced strategies for photocatalytic and photoelectrochemical CO2 reduction. Chem Eng J 2023;457:141179.
11. Liu L, Zhang Y, Huang H. Junction engineering for photocatalytic and photoelectrocatalytic CO2 reduction. Solar RRL 2021;5:2000430.
12. Brillas E, Serrà A, Garcia-segura S. Biomimicry designs for photoelectrochemical systems: Strategies to improve light delivery efficiency. Curr Opin Electrochem 2021;26:100660.
13. Devi P, Verma R, Singh JP. Advancement in electrochemical, photocatalytic, and photoelectrochemical CO2 reduction: recent progress in the role of oxygen vacancies in catalyst design. J CO2 Util 2022;65:102211.
14. Putri LK, Ng BJ, Ong WJ, Chai SP, Mohamed AR. Toward excellence in photocathode engineering for photoelectrochemical CO2 reduction: design rationales and current progress. Adv Energy Mater 2022;12:2201093.
15. Li Y, Li S, Huang H. Defective photocathode: fundamentals, construction, and catalytic energy conversion. Adv Funct Mater 2023;33:2304925.
16. Pawar AU, Kim CW, Nguyen-le M, Kang YS. General review on the components and parameters of photoelectrochemical system for CO2 reduction with in situ analysis. ACS Sustainable Chem Eng 2019;7:7431-55.
17. King AJ, Bui JC, Bell AT, Weber AZ. Establishing the role of operating potential and mass transfer in multicarbon product generation for photoelectrochemical CO2 reduction cells using a Cu catalyst. ACS Energy Lett 2022;7:2694-700.
18. Verma S, Yadav A. Emerging single-atom catalysts and nanomaterials for photoelectrochemical reduction of carbon dioxide to value-added products: a review of the current state-of-the-art and future perspectives. Energy Fuels 2023;37:5712-42.
19. Hiragond CB, Kim J, Kim H, Bae D, In S. Elemental-doped catalysts for photoelectrochemical CO2 conversion to solar fuels. Solar RRL 2024;8:2400022.
20. Bienkowski K, Solarska R, Trinh L, et al. Halide perovskites for photoelectrochemical water splitting and CO2 reduction: challenges and opportunities. ACS Catal 2024;14:6603-22.
21. Li CF, Guo RT, Zhang ZR, Wu T, Pan WG. Converting CO2 into value-added products by Cu2O-based catalysts: from photocatalysis, electrocatalysis to photoelectrocatalysis. Small 2023;19:e2207875.
22. Zhang W, Jin Z, Chen Z. Rational-designed principles for electrochemical and photoelectrochemical upgrading of CO2 to value-added chemicals. Adv Sci 2022;9:e2105204.
23. Zheng Y, Vasileff A, Zhou X, Jiao Y, Jaroniec M, Qiao SZ. Understanding the roadmap for electrochemical reduction of CO2 to multi-carbon oxygenates and hydrocarbons on copper-based catalysts. J Am Chem Soc 2019;141:7646-59.
24. Zheng W, Yang X, Li Z, et al. Designs of tandem catalysts and cascade catalytic systems for CO2 upgrading. Angew Chem Int Ed Engl 2023;62:e202307283.
25. Han GH, Bang J, Park G, et al. Recent advances in electrochemical, photochemical, and photoelectrochemical reduction of CO2 to C2+ products. Small 2023;19:e2205765.
26. Otgonbayar Z, Yoon CM, Oh WC. Photoelectrocatalytic CO2 reduction with ternary nanocomposite of MXene (Ti3C2)-Cu2O-Fe3O4: comprehensive utilization of electrolyte and light-wavelength. Chem Eng J 2023;464:142716.
27. Landaeta E, Kadosh NI, Schultz ZD. Mechanistic study of plasmon-assisted in situ photoelectrochemical CO2 reduction to acetate with a Ag/Cu2O nanodendrite electrode. ACS Catal 2023;13:1638-48.
28. Liang H, Li M, Li Z, Xie W, Zhang T, Wang Q. Photoelectrochemical CO2 reduction with copper-based photocathodes. J CO2 Util 2024;79:102639.
29. Guo X, Wang C, Yang Z, Yang Y. Boosting C2+ production from photoelectrochemical CO2 reduction on gallium doped Cu2O. Chem Eng J 2023;471:144539.
30. Wang B, Yang F, Dong Y, et al. Cu@porphyrin-COFs nanorods for efficiently photoelectrocatalytic reduction of CO2. Chem Eng J 2020;396:125255.
31. Wang J, Guan Y, Yu X, et al. Photoelectrocatalytic reduction of CO2 to paraffin using p-n heterojunctions. iScience 2020;23:100768.
32. Cao H, Yu H, Lu Y, et al. Photoelectrocatalytic reduction of CO2 over CuBi2O4/TiO2-NTs under simulated solar irradiation. ChemistrySelect 2020;5:5137-45.
33. Lu Y, Cao H, Xu S, et al. CO2 photoelectroreduction with enhanced ethanol selectivity by high valence rhenium-doped copper oxide composite catalysts. J Colloid Interface Sci 2021;599:497-506.
34. Zhou S, Sun K, Huang J, et al. Accelerating electron-transfer and tuning product selectivity through surficial vacancy engineering on CZTS/CdS for photoelectrochemical CO2 reduction. Small 2021;17:e2100496.
35. de Souza MKR, Cardoso EDSF, Fortunato GV, et al. Combination of Cu-Pt-Pd nanoparticles supported on graphene nanoribbons decorating the surface of TiO2 nanotube applied for CO2 photoelectrochemical reduction. J Environ Chem Eng 2021;9:105803.
36. Nandal N, Prajapati PK, Abraham BM, Jain SL. CO2 to ethanol: a selective photoelectrochemical conversion using a ternary composite consisting of graphene oxide/copper oxide and a copper-based metal-organic framework. Electrochim Acta 2022;404:139612.
37. Merino-garcia I, Castro S, Irabien A, et al. Efficient photoelectrochemical conversion of CO2 to ethylene and methanol using a Cu cathode and TiO2 nanoparticles synthesized in supercritical medium as photoanode. J Environ Chem Eng 2022;10:107441.
38. Wang K, Liu Y, Wang Q, et al. Asymmetric Cu-N sites on copper oxide photocathode for photoelectrochemical CO2 reduction towards C2 products. Appl Catal B Environ 2022;316:121616.
39. Lu M, Jia D, Xue H, Tian J, Jiang T. 0D/1D CuFeO2/CuO nanowire heterojunction arrays for improved photoelectrocatalytic reduction of CO2 to ethanol. J Alloys Compd 2023;960:170626.
40. Kim C, King AJ, Aloni S, Toma FM, Weber AZ, Bell AT. Codesign of an integrated metal-insulator-semiconductor photocathode for photoelectrochemical reduction of CO2 to ethylene. Energy Environ Sci 2023;16:2968-76.
41. Cardoso J, Stulp S, de Souza M, et al. The effective role of ascorbic acid in the photoelectrocatalytic reduction of CO2 preconcentrated on TiO2 nanotubes modified by ZIF-8. J Electroanal Chem 2020;856:113384.
42. Bergamini L, Sangiorgi N, Gondolini A, et al. CsPbBr3/platinum and CsPbBr3/graphite hybrid photoelectrodes for carbon dioxide conversion to oxalic acid. Solar Energy 2023;254:213-22.
43. Zhang Y, Han B, Xu Y, et al. Artificial photosynthesis of alcohols by multi-functionalized semiconductor photocathodes. ChemSusChem 2017;10:1742-8.
44. Wang J, Han B, Nie R, et al. Photoelectrocatalytic reduction of CO2 to chemicals via ZnO@Nickel foam: controlling C-C coupling by ligand or morphology. Top Catal 2018;61:1563-73.
45. Han B, Wang J, Yan C, et al. The photoelectrocatalytic CO2 reduction on TiO2@ZnO heterojunction by tuning the conduction band potential. Electrochim Acta 2018;285:23-9.
46. Wang J, Wei Y, Yang B, Wang B, Chen J, Jing H. In situ grown heterojunction of Bi2WO6/BiOCl for efficient photoelectrocatalytic CO2 reduction. J Catal 2019;377:209-17.
47. Wang L, Wei Y, Fang R, et al. Photoelectrocatalytic CO2 reduction to ethanol via graphite-supported and functionalized TiO2 nanowires photocathode. J Photochem Photobiol A Chem 2020;391:112368.
48. Yu X, Wei Y, Chen Y, Wang J, Jing H. P-doped WO3 semiconductor with enhanced conduction band on highly efficient photoelectrocatalytic reduction of CO2. Chinese Sci Bull 2020;66:825-32.
49. Liu C, Xiao Y, Wan W, et al. Different behaviors on the external and inner surface of hollow CdS/VS-MoS2 heterojunctions in photoelectrocatalytic CO2 reduction via SH-assisted mechanism. Appl Catal B Environ 2023;325:122394.
50. Xu Y, Wang F, Lei S, et al. In situ grown two-dimensional TiO2/Ti3CN MXene heterojunction rich in Ti3+ species for highly efficient photoelectrocatalytic CO2 reduction. Chem Eng J 2023;452:139392.
51. Wei Y, Duan R, Zhang Q, et al. Photoelectrocatalytic reduction of CO2 catalyzed by TiO2/TiN nanotube heterojunction: nitrogen assisted active hydrogen mechanism. Chinese J Catal 2023;47:243-53.
52. Cao Y, Wei Y, Wan W, et al. Photoelectrochemical reduction of CO2 catalyzed by a 3D core-shell NiMoO4@ZnO heterojunction with bicentre at the (111) plane and thermal electron assistance. J Mater Chem A 2023;11:4230-7.
53. Wan W, Zhang Q, Wei Y, et al. p-n heterojunctions of Si@WO3 mimicking thylakoid for photoelectrocatalytic CO2 reduction to C2+ products - morphology control. Chem Eng J 2023;454:140122.
54. Kumaravel V, Bartlett J, Pillai SC. Photoelectrochemical conversion of carbon dioxide (CO2) into fuels and value-added products. ACS Energy Lett 2020;5:486-519.
55. Chu S, Rashid RT, Pan Y, Wang X, Zhang H, Xiao R. The impact of flue gas impurities and concentrations on the photoelectrochemical CO2 reduction. J CO2 Util 2022;60:101993.
56. Jiang X, Chen R, Chen YX, Lu CZ. Proton exchange membrane fuel cells: application for value-added chemical productions. Chem Synth 2024;4:6.
57. Gao D, Wei P, Li H, Lin L, Wang G, Bao X. Designing electrolyzers for electrocatalytic CO2 reduction. Acta Phys Chim Sin 2021;37:2009021.
Cite This Article

How to Cite
Jiang, X.; Chen, R.; Chen, Y. X.; Lu, C. Z. Research progress of photoelectrochemical conversion of CO2 to C2+ products. Chem. Synth. 2024, 4, 46. http://dx.doi.org/10.20517/cs.2024.03
Download Citation
Export Citation File:
Type of Import
Tips on Downloading Citation
Citation Manager File Format
Type of Import
Direct Import: When the Direct Import option is selected (the default state), a dialogue box will give you the option to Save or Open the downloaded citation data. Choosing Open will either launch your citation manager or give you a choice of applications with which to use the metadata. The Save option saves the file locally for later use.
Indirect Import: When the Indirect Import option is selected, the metadata is displayed and may be copied and pasted as needed.
About This Article
Copyright
Author Biographies
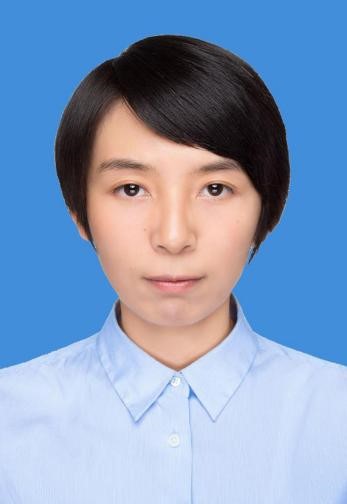
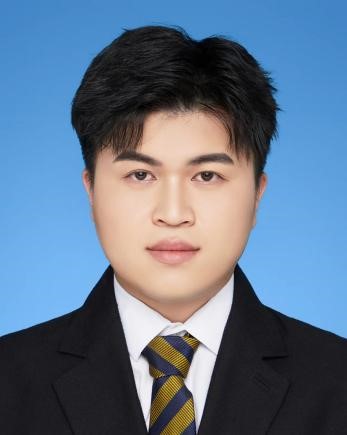
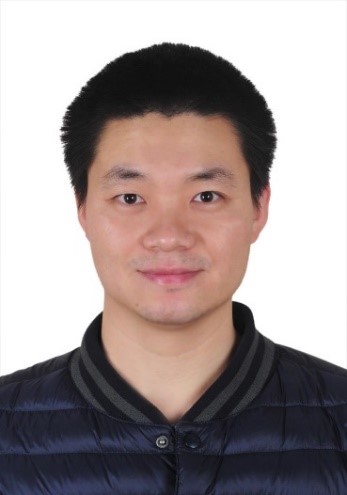
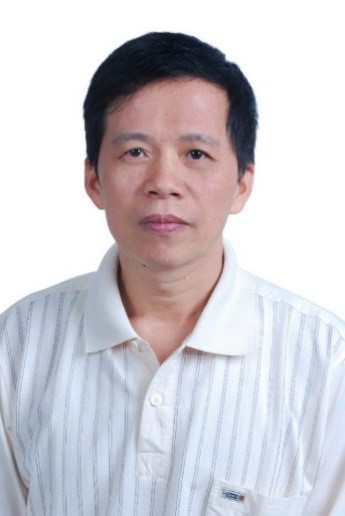
Comments
Comments must be written in English. Spam, offensive content, impersonation, and private information will not be permitted. If any comment is reported and identified as inappropriate content by OAE staff, the comment will be removed without notice. If you have any queries or need any help, please contact us at support@oaepublish.com.