Fluorinated porous organic frameworks for C2F6/CF4 gases separation
Abstract
As an indispensable raw material for silicon-based semiconductor industry, carbon tetrafluoride (CF4) is widely used as plasma etching and cleaning gas in the manufacture of semiconductors. How to efficiently remove the C2F6 impurity during the CF4 production process is a challenging task as semiconductor industry requires high-purity CF4 gas. Herein, two fluorine-functionalized porous organic frameworks (F-POFs) named SPPOF-4F and SPPOF-8F were synthesized and used for separation of C2F6/CF4 gases. Single-component gas adsorption experiments and ideal adsorbed solution theory (IAST) calculations indicate that two porous organic frameworks can selectively adsorb C2F6 from C2F6/CF4 mixture. Molecular simulations have further complemented these experimental findings by revealing F-induced host-guest interactions between F-POFs and C2F6 at a molecular level. Additionally, dynamic breakthrough experiments verified that the F-POFs can capture C2F6 in C2F6/CF4 mixture at practical conditions. These results indicate that F-POFs have great potential for application in the separation and purification of CF4 electronic special gases.
Keywords
INTRODUCTION
Carbon tetrafluoride (CF4) is a perfluoro-electronic specialty gas that plays an indispensable role in the production of semiconductor electronics[1-5]. The direct fluorination of carbon using F2 gas is the most dominant method to produce CF4 due to the advantages of easily obtainable raw materials, a controllable process, and high yield[6-8]. In the direct fluorination of carbon, other fluorinated hydrocarbon impurities including C2F6 are also obtained[9]. Removing C2F6 impurities is a key issue to obtaining high-purity CF4.
Facing this challenge, various methods have been employed for CF4 purification including distillation, adsorption and membrane separation[10-12]. Cryogenic distillation is presently the predominant method for gas separation; however, its substantial investment and energy costs may not be sustainable for the removal of trace C2F6 impurities from CF4[13,14]. Adsorption separation is regarded as a sustainable and eco-friendly option for the purification of gases, offering potential energy savings[15-25]. A variety of porous adsorbents, including activated carbon (AC), zeolites, porous alumina, metal-organic frameworks (MOFs), and some porous organic frameworks (POFs)[26-30], have been documented for the adsorption and separation of perfluorinated gases[30-39]. Compared to traditional porous adsorbents such as zeolite and MOFs[40-43], POFs with strong covalent bonds show excellent acid resistance[44-47], which are more suitable used in CF4 purification as fluorination of carbon using F2 usually accompanied by HF gas impurities[9].
Herein, we designed and synthesized two fluorine-functionalized POFs (F-POFs), namely SPPOF-4F and SPPOF-8F, to selectively remove C2F6 impurities from CF4. Single-component adsorption experiments and dynamic breakthrough experiments demonstrated that SPPOF-4F and SPPOF-8F were able to capture C2F6 from C2F6/CF4 gas mixtures, and in particular, SPPOF-8F showed an ideal adsorbed solution theory (IAST) selectivity of 8.26 when C2F6 : CF4 is 1:99. In addition, density-functional theory (DFT)-based theoretical calculations provide insights into the internal mechanism of selective adsorption of C2F6 in F-POFs at the molecular level.
EXPERIMENTAL
Chemicals
Chemicals 2,2’,7,7’-Tetrabromo-9,9’-spirobifluorene (referred to as SP-4Br), 1,2,4,5-tetrafluorobenzene (referred to as Benz-4F), 4h,4’h-octafluorobiphenyl (referred to as Biph-8F) and Pd(OAc)2 were procured from Aladdin. The compound Di-tert-butyl(methyl)phosphonium tetrafluoroborate was obtained from Bidepharm. Anhydrous K2CO3 was acquired from Macklin. High-purity (99.99%) C2F6 and CF4 were sourced from Wuhan Newradar Special Gas Co. Ltd. Unless specifically specified, all chemicals involved in this study are readily available for commercial purchase and utilized without additional purification.
Synthesis
SPPOF-4F was synthesized following previously reported literature methods with minor modifications[48]. In a typical synthesis route, SP-4Br (316.0 mg,0.5 mmol), Benz-4F (111.6 µL, 1.0 mmol), anhydrous K2CO3 (276.4 mg, 2.0 mmol) and P(t-Bu)2Me-HBF4 (24.7 mg, 0.1 mmol) were dissolved in anhydrous dimethylacetamide (DMAc, 15 mL). Pd(OAc)2 (11.2 mg, 0.05 mmol) was introduced into the mixture in a nitrogen-filled environment following degassing through freeze-pump-thaw cycles three times. The reaction mixture was agitated for 48 hours in a nitrogen environment at 120 °C. After the reaction had been cooled to room temperature, the resultant mixture was subsequently transferred into
The synthesis of SPPOF-8F was carried out following the identical procedure used for SPPOF-4F. Initially, SP-4Br (316.0 mg, 0.5 mmol), Biph-8F (298.2 mg, 1.0 mmol), P(t-Bu)2Me-HBF4 (24.7 mg, 0.1 mmol), and
Analytical characterization
The Fourier transform infrared (FT-IR) spectra were acquired using a Nicolet iS50 attenuated total reflectance IR spectrometer. The powder X-ray diffraction (PXRD) patterns of the samples were obtained with a Bruker D8 Advance instrument. Scanning electron microscopy (SEM) images were captured using an MAIA3LMH microscope. Elemental analysis was conducted via Aztec X-maxN 50mm2 energy dispersive spectroscopy (EDS) analyzer. The 13C solid-state cross polarization/magic angle spinning nuclear magnetic resonance (CP/MAS NMR) spectra are collected by Bruker 400M. The X-ray photoelectron spectroscopy (XPS) tests were conducted by Thermo Scientific K-Alpha+. The thermogravimetric analysis (TGA) of two F-POFs was carried out using a TGA instrument (type: NETZSCH TG 209F3) under an air atmosphere and a temperature range from 35 to 800 °C at a heating rate of 10 °C/min. All samples were weighed within the range (5~6 mg). Nitrogen adsorption/desorption isotherms at 77 K were obtained using a Micromeritics ASAP 2460 analyzer. C2F6 and CF4 isotherms at various temperatures were recorded with a BSD-PM2 gas adsorption Analyzer from Beishide Instrument Technology (Beijing) Co., LTD. Before each measurement, the samples (approximately 150 mg) were subjected to heating at 393.15 K under dynamic vacuum conditions for a duration of 12 h. High-purity (≥ 99.99%) gases (C2F6 and CF4) were utilized for the isotherm measurements.
Computational details
Isotherms fitting
Single-component isotherms of C2F6 and CF4 were modeled using a dual-site Langmuir-Freundlich model across the entire pressure range (0~1 bar).
Here, N is molar loading of gas (mmol·g-1), A is saturation capacity (mmol·g-1), B is Langmuir constant (kPa-1 or kPa-C), C is Freundlich constant, and P represents the equilibrium pressure of the bulk gas in contact with the adsorbed phase (bar).
Isosteric heat of adsorption
The adsorption data for C2F6 and CF4 were modeled using a virial-type expression consisting of temperature-independent parameters ai and bi:
where P represents pressure in millimeters of mercury (mmHg), N denotes the amount adsorbed in milligrams per gram (mg/g), Ti stands for temperature in Kelvin (K), and m and n are the respective parameters ai and bi.
The isosteric heat of adsorption, Qst, is formally defined as:
where R is the gas constant, 8.314 J·mol-1·K-1.
IAST calculations
The selectivity of C2F6/CF4 in a mixture gas can be defined as:
where x1 and y1 (x2 and y2) represent the molar fractions of C2F6 and CF4 in the adsorbed and bulk phases, respectively. The values of x1 and x2 were determined using the IAST developed by Myers and Prausnitz.
Theoretical simulation calculation
Simulation calculations were conducted to investigate the adsorption and separation mechanism of C2F6 and CF4 in the POFs. The interaction energy (EInt) distribution and the energetic minimal configuration are simulated using the Sorption module of Materials Studio. The Mulliken atomic charge and the electrostatic potential distribution of gases, POFs and POFs@gas were calculated by the DMol3 module in Materials Studio.
RESULTS AND DISCUSSION
Structure and characterization
In a typical synthesis process, SPPOF-4F and SPPOF-8F were synthesized through the polycondensation of aryl fluorides with aryl bromide as per previously reported methods with minor adjustments[48] [Scheme 1]. In order to confirm the functional group composition of SPPOF-4F and SPPOF-8F, Fourier infrared transform (FT-IR) analysis was performed and the results were shown in Figure 1. In the FT-IR spectra, distinct C−F characteristic absorption peaks were observed for the aryl fluoride monomer, with a stretching vibration peak near 1,200 cm-1 corresponding to C−F. Furthermore, SP-4Br displayed a prominent peak at approximately 520 cm-1 associated with the stretching vibration of C−Br bonds. A comparison of the monomer spectra reveals the absence of characteristic absorption peaks attributed to C−Br bonds in the spectra of SPPOF-4F and SPPOF-8F, indicating successful condensation of aryl fluoride and aryl bromide. Additionally, the stretching vibration peaks related to C−F bonds are evident in the spectra of both products, suggesting successful preservation of C−F bonds during the polycondensation reaction[49]. The crystallinity of the POFs was assessed using PXRD, revealing amorphous patterns for both SPPOF-4F and SPPOF-8F polymers [Supplementary Figure 1].
Figure 1. FT-IR spectra of monomers and POFs: (A) SPPOF-4F; (B) SPPOF-8F; and XPS spectra enlarged views of C1s in (C) SPPOF-4F and (D) SPPOF-8F. FT-IR: Fourier transform infrared; POFs: porous organic frameworks; XPS: X-ray photoelectron spectroscopy.
The chemical bonds and elemental compositions of the two F-POFs were examined using XPS for analysis. As shown in Supplementary Figure 2, the XPS spectra of SPPOF-4F and SPPOF-8F revealed the existence of carbon and fluorine in their compositions, featuring binding energies of 285.1 and 687.1 eV for carbon and fluorine, respectively. The enlarged portions of the XPS spectra in the C1s segment are shown in Figure 1C and D.The findings suggest that the C−F bonding signals in SPPOF-8F are notably more pronounced than those in SPPOF-4F, likely due to the higher concentration of F atoms present in SPPOF-8F. To further validate the molecular structures of the two polymers, 13C solid-state CP/MAS NMR characterization was conducted. The 13C NMR spectra and corresponding C-resonance distributions of the two F-POFs and their synthetic monomers are depicted in Supplementary Figure 3. The results show that the resonance signals of the aromatic monomers and the carbon atoms on the F-substituted benzene rings were detected in both polymers. It is worth noting that SPPOF-8F exhibited a signal at approximately 111 ppm, corresponding to the carbon situated at the junction of the two fluorinated benzene rings in octafluorobiphenyl. In contrast, SPPOF-4F did not display such a signal in this specific region. The thermal stability of both POFs was assessed using TGA [Supplementary Figure 4], and the findings indicated that both SPPOF-4F and
SEM was utilized to examine the microscopic morphology of SPPOF-4F and SPPOF-8F. As depicted in Figure 2, both F-POFs exhibited irregular morphologies [Figure 2A and C]. Based on this, the composition and dispersibility of various elements within the F-POFs were examined using EDS analysis, with the results presented in Supplementary Figure 6A-D. It is clear that F is evenly distributed in both F-POFs. Additionally, the C/F atomic ratios of SPPOF-4F and SPPOF-8F are approximately 5.02 and 2.92 [Figure 2B and D], respectively, which closely align with the theoretical C/F atomic ratios for the two F-POFs
Figure 2. Morphology images of (A) SPPOF-4F and (B) SPPOF-8F obtained by SEM; EDS analysis curves for (C) SPPOF-4F and (D) SPPOF-8F. SEM: Scanning electron microscopy; EDS: energy dispersive spectroscopy.
N2 adsorption tests were conducted on SPPOF-4F and SPPOF-8F at 77 K, and the resulting adsorption-desorption isotherms are presented in Figure 3A and B. It can be seen that both F-POFs exhibit characteristic Type IV adsorption isotherms with hysteresis in the desorption. Based on the N2 adsorption isotherms at 77 K and the Brunauer-Emmett-Teller (BET) adsorption type, the specific surface areas of SPPOF-4F and SPPOF-8F were calculated to be 915 m2·g-1 and 971 m2·g-1, respectively. Meanwhile, the non-local density functional theory (NLDFT) model was employed to calculate the pore size distribution (PSD) of two F-POFs, as depicted in Figure 3C and D. It is evident that the main pore sizes of SPPOF-4F and SPPOF-8F are concentrated at 1.35 and 1.55 nm, respectively, with some mesopores in 2-10 nm, proving that both SPPOF-4F and SPPOF-8F are typical micro-mesoporous materials[50].
Single-component adsorption
As shown in Figure 4, the single-component adsorption isotherms of C2F6 and CF4 were tested at 273 K and 298 K for SPPOF-4F and SPPOF-8F, respectively. The adsorption capacities of SPPOF-4F and SPPOF-8F for C2F6 exhibited a significantly higher affinity compared to that for CF4. At 273 K, the adsorption capacities of C2F6 in SPPOF-4F and SPPOF-8F were found to be 39.0 and 40.9 mL/g, respectively. In comparison, the uptake of CF4 in SPPOF-4F and SPPOF-8F under identical conditions yielded adsorption capacities of 17.8 and 16.0 mL/g, respectively. Besides, the adsorption isotherms of C2F6 showed a rapid upward trend throughout the adsorption range, while the adsorption isotherms of CF4 were almost linear. Furthermore, it was observed that the adsorption of C2F6 by both F-POFs at 273 K exceeded that at 298 K, with complete desorption of C2F6 occurring upon pressure reduction, indicating a typical physical adsorption process for C2F6 in SPPOF-4F and SPPOF-8F. We also conducted a comparative analysis of the C2F6/CF4 separation performance of adsorbents reported in the existing literature. The findings indicate that while SPPOFs may not exhibit the highest adsorption capacity for C2F6, their separation selectivity for
Isosteric heat of adsorption
The Qst is widely used to evaluate the thermodynamic adsorption affinity of an adsorbent toward a gas molecule. Herein, the Qst of C2F6 and CF4 were determined using the Virial equation based on adsorption isotherms. The Qst curves of SPPOF-4F and SPPOF-8F for the two gases show different trends, as shown in Figure 5. Specifically, at near-zero coverage, the Qst values of SPPOF-4F for C2F6 and CF4 are 29.0 and
Adsorption selectivity
To predict the separation efficiency of SPPOF-4F and SPPOF-8F for gas mixtures (C2F6 and CF4), C2F6/CF4 ideal adsorption solution theory (IAST) selectivity was determined by fitting the adsorption isotherms with the Langmuir-Freundlich model. Figure 6A and B shows the IAST selectivity of F-POFs for C2F6/CF4 at different ratios (C2F6 gas content from 1% to 99%). At 298.15 K and 1 bar, SPPOF-4F and SPPOF-8F demonstrate maximum IAST selectivity for C2F6/CF4 at low C2F6 gas concentration (C2F6 : CF4 =1:99, v/v) with values of 6.72 and 8.26, respectively. As the percentage of C2F6 in the mixture increases to 99%, the IAST selectivity of C2F6/CF4 decreases to 4.90 and 5.98 for SPPOF-4F and SPPOF-8F, respectively. The IAST selectivity demonstrates a consistent decrease with the increase in the percentage of C2F6 in the gas mixture. It is worth mentioning that higher fluorine content of SPPOF-8F leads to a stronger F-induced interaction force between the F-POFs and C2F6, resulting in its higher IAST selectivity compared to that of SPPOF-4F for mixed gases across all compositions.
Time-dependent adsorption kinetics
In order to investigate the kinetic adsorption of gas in F-POFs, we conducted tests on the adsorption rates of C2F6 and CF4 in SPPOF-4F and SPPOF-8F at 298.15 K and 1 bar. As depicted in Figure 7, both SPPOF-4F and SPPOF-8F exhibit initial high adsorption rates for both gases, followed by a rapid decrease with increasing adsorption time. The maximum adsorption rates of SPPOF-4F for C2F6 and CF4 were 20.4 and
Dynamic breakthrough experiments
In order to assess the practical applicability of SPPOF-4F and SPPOF-8F, breakthrough experiments were conducted at 298.15 K to investigate the dynamic separation of C2F6/CF4 gas mixtures. The feed gas consisted of a 1:1 (v/v) volume ratio mixture of C2F6 and CF4, with a constant flow rate of 80 mL/min. Gas composition was monitored using an INFICON Mass spectrometer. In Figure 8, it is shown that during the test, CF4 initially passed through the packed column until the relative concentrations of both CF4 and C2F6 stabilized at around 1. For SPPOF-4F, CF4 reached adsorption saturation at 2.4 min/g after the start of the experiment, while the adsorption saturation time for C2F6 was 5.1 min/g [Figure 8A]. In contrast, for SPPOF-8F, CF4 reached adsorption saturation at 2.9 min/g after the beginning of the experiment, whereas C2F6 adsorption saturated at up to 6.3 min/g [Figure 8B]. The adsorption saturation time for C2F6 was significantly longer than that for CF4 for both polymers, which further confirms the potential of samples in separating C2F6. It is noteworthy that the time windows for the purification of CF4 by the two F-POFs were relatively limited, approximately 2.7 min/g for SPPOF-4F and 3.4 min/g for SPPOF-8F, which may be attributed to the utilization of a shorter packing column in this experimental setup.
Theoretical simulation and calculation of gas adsorption mechanism
Theoretical calculations employing DFT were conducted to enhance our comprehension of the adsorption mechanism of C2F6 and CF4 in F-POFs. Repetitive structural unit fragment models of the polymers were constructed using Material Studio Visualizer, and calculations were performed with the DMol3 module. The electrostatic potential distribution of the gas molecules and POFs structural units was computed using Mulliken analysis, as depicted in Figure 9A-D. The blue region represents areas of low potentials, while the red-covered region indicates high potentials. The intensity of the color is indicative of the magnitude of the electrostatic potentials. Notably, a dark blue color is observed in the region where the F atoms are located, signifying a strong negative potential. Simultaneously, the center of the benzene ring and the H atoms are depicted in red, indicating high positive potential. The introduction of F atoms apparently induces polarization in the porous polymer, resulting in a potential gradient on the pore surface. This enhances the electrostatic force exerted by the polymer on the C2F6 molecule due to the presence of positive potential centers[51,52]. Additionally, C2F6 molecules with more F atoms have a stronger negative electric field than CF4, so theoretically F-POFs have a stronger affinity for C2F6.
Figure 9. Simulated structures and the electrostatic potential maps of (A) CF4, (B) C2F6, (C) SPPOF-4F and (D) SPPOF-8F; The EInt between the POFs and gas molecules: (E) SPPOF-4F@CF4, (F) SPPOF-4F@C2F6, (G) SPPOF-8F@CF4 and (H) SPPOF-8F@C2F6. POFs: Porous organic frameworks.
Based on the above results, we constructed adsorption models for the two F-POFs with C2F6 and CF4, as illustrated in Figure 9E-H. The electrostatic potential simulation shows that gas molecules preferentially adsorb near the benzene ring with a positive potential [Supplementary Figure 7]. The simulation results of Mulliken atomic charge indicate that the charge transfer induced by SPPOF-8F with a higher F content to C2F6 is 0.0046 (a.u.), which is significantly greater than the 0.0016 (a.u.) observed for SPPOF-4F
Evaluation of reusability and stability
The practical application requires crucial considerations of the reusability and stability of adsorbents; as illustrated in Figure 10A, it is evident that the adsorption capacity of SPPOF-8F for C2F6 and CF4 remained relatively constant after six consecutive cycling tests at 298 K, demonstrating excellent reusability and stability of both F-POFs. In addition, we soaked the two POFs in 1 M hydrochloric acid and 1 M NaOH solutions at room temperature for two days to evaluate their chemical stability. The treated samples were subsequently gathered, analyzed using FT-IR, and exposed to C2F6 adsorption trials at 298 K and 1 bar. Additionally, the C2F6 adsorption isotherms [Figure 10B and C] and FT-IR spectra
CONCLUSIONS
In this study, two F-POFs were synthesized and exhibited high adsorption selectivity for C2F6/CF4 separation. The adsorption capacities of SPPOF-8F for C2F6 and CF4 were 40.9 and 16.0 mL/g at 273 K and
DECLARATIONS
Authors’ contributions
Designed the research, wrote the paper: Ma H, Li Y
Carried out the experiments and tests: Li Y
Assisted in data analysis and theoretical calculations: Wu Y, Zhang W
Programming calculations for Qst and IAST selectivity: Fu Y
Participated in the characterization of materials: Wu Y, Wang S, Li X, Zeng J
Supervised the project: Ma H
Availability of data and materials
The authors confirm that the data supporting the findings of this study are available within the article and its Supplementary Materials (testing and characterization of SPPOFs and supporting figures). If there is any uncertainty, we kindly advise reaching out to the corresponding author for additional clarification.
Financial support and sponsorship
The authors gratefully acknowledge the financial support of the Natural Science Foundation of China (Grant No. 22178280), the Shaanxi Province Qin Chuangyuan “Scientist + Engineer” Team Construction Project (Grant No. 2023KXJ-058), and the Fundamental Research Funds for the Central Universities (Grant No. xzy012023171). Ma H also thanks the Fundamental Research Funds for “Young Talent Support Plan” of Xi’an Jiaotong University (HG6J001) and “1000-Plan program” of Shaanxi province. We also extend our gratitude to the Instrument Analysis Center of Xi’an Jiao Tong University for the assistance test. Additionally, we gratefully acknowledge Prof. Huanyu Zhao at Jilin University for help in getting access to mass spectrometry (MS).
Conflicts of interest
All authors declared that there are no conflicts of interest.
Ethical approval and consent to participate Consent for publication
Not applicable.
Consent for publication
Not applicable.
Copyright
© The Author(s) 2024.
Supplementary Materials
REFERENCES
1. Chang MB, Chang JS. Abatement of PFCs from semiconductor manufacturing processes by nonthermal plasma technologies: a critical review. Ind Eng Chem Res 2006;45:4101-9.
2. Donnelly VM. Review article: reactions of fluorine atoms with silicon, revisited, again. J Vac Sci Technol A Vac Surf Films 2017;35:05C202.
3. Vorotyntsev AV, Petukhov AN, Trubyanov MM, et al. Progress and perspectives in high-purity substance production for semiconductor industry. Rev Chem Eng 2021;37:125-61.
4. Illuzzi F, Thewissen H. Perfluorocompounds emission reduction by the semiconductor industry. J Integr Environ Sci 2010;7:201-10.
5. World Semiconductor Trade Statistics. WSTS semiconductor market forecast fall 2023. Available from: https://www.wsts.org/76/103/WSTS-Semiconductor-Market-Forecast-Fall-2023. [Last accessed on 24 Jul 2024].
6. Tang Z. The synthesis technology of high purity tetrafluoromethane by fluorine and carbon. Cryo Spec Gases 2013;31:32-4. (in Chinese).
7. Pashkevich DS, Mukhortov DA, Petrov VB, Alekseev YI, Asovich VS, Barabanov VG. Synthesis of tetrafluoromethane by graphite fluorination with elemental fluorine. Russian J Appl Chem 2004;77:92-7.
8. Pashkevich DS, Shelopin GG, Mukhortov DA, Petrov VB, Alekseev YI, Asovich VS. Synthesis of perfluoroalkanes by high-temperature reaction of graphite with fluorine in a fluidized bed. Russ J Appl Chem 2004;77:1847-53.
9. Chen G, Ni Z. CN101298318A Method and apparatus for preparing high-purity carbon tetrafluoride gas. 2008. Available from: https://worldwide.espacenet.com/patent/search/family/040078304/publication/CN101298318A?q=CN101298318A. [Last accessed on 24 Jul 2024].
10. Bao H, Timothy A. US5779863A Perfluoro compound (PFC) separation and purification method and system. 1997. Available from: https://worldwide.espacenet.com/patent/search/family/025129273/publication/US5779863A?q=US5779863A. [Last accessed on 24 Jul 2024].
11. Asensio-Delgado S, Pardo F, Zarca G, Urtiaga A. Absorption separation of fluorinated refrigerant gases with ionic liquids: equilibrium, mass transport, and process design. Sep Purif Technol 2021;276:119363.
12. Vorotyntsev VM, Drozdov PN, Vorotyntsev IV, Anikin AE, Beljaev EM, Soboleva YA. The physico-chemical bases of separation and high purification of fluorocarbons and simple gases. Pet Chem 2011;51:492-5.
13. Battisti R, Machado RA, Marangoni C. A background review on falling film distillation in wetted-wall columns: from fundamentals towards intensified technologies. Chem Eng Process Process Intensif 2020;150:107873.
14. Ma H, Sun J, Li Z, Liu X. Research progress and optimization prospect of constant boiling distillation technology. E3S Web Conf 2021;290:03025.
15. Wang H, Shi Z, Yang J, et al. Docking of CuI and AgI in metal-organic frameworks for adsorption and separation of xenon. Angew Chem Int Ed Engl 2021;60:3417-21.
16. Wu D, Zhang P, Yang G, et al. Supramolecular control of MOF pore properties for the tailored guest adsorption/separation applications. Coord Chem Rev 2021;434:213709.
17. Mukherjee S, Sensharma D, Qazvini OT, et al. Advances in adsorptive separation of benzene and cyclohexane by metal-organic framework adsorbents. Coord Chem Rev 2021;437:213852.
18. Mohammed N, Lian H, Islam MS, et al. Selective adsorption and separation of organic dyes using functionalized cellulose nanocrystals. Chem Eng J 2021;417:129237.
19. Ding Y, Alimi LO, Moosa B, et al. Selective adsorptive separation of cyclohexane over benzene using thienothiophene cages. Chem Sci 2021;12:5315-8.
20. Lv D, Zhou P, Xu J, et al. Recent advances in adsorptive separation of ethane and ethylene by C2H6-selective MOFs and other adsorbents. Chem Eng J 2022;431:133208.
21. Gong W, Xie Y, Pham TD, et al. Creating optimal pockets in a clathrochelate-based metal-organic framework for gas adsorption and separation: experimental and computational studies. J Am Chem Soc 2022;144:3737-45.
22. Brandt P, Nuhnen A, Öztürk S, Kurt G, Liang J, Janiak C. Comparative evaluation of different MOF and non-MOF porous materials for SO2 adsorption and separation showing the importance of small pore diameters for low-pressure uptake. Adv Sustain Syst 2021;5:2000285.
23. Rehman A, Nazir G, Yop Rhee K, Park S. A rational design of cellulose-based heteroatom-doped porous carbons: promising contenders for CO2 adsorption and separation. Chem Eng J 2021;420:130421.
24. Xie W, Yang L, Zhang J, Zhao X. The adsorptive separation of ethylene from C2 hydrocarbons by metal-organic frameworks. Chemistry 2023;29:e202300158.
25. Guo Y, Su C, Chen H, et al. Hierarchical porous carbon with tunable apertures and nitrogen/oxygen heteroatoms for efficient adsorption and separation of VOCs. Chem Eng J 2023;471:144558.
26. Ryu U, Jee S, Rao PC, et al. Recent advances in process engineering and upcoming applications of metal-organic frameworks. Coord Chem Rev 2021;426:213544.
28. Ma Y, Cui F, Rong H, et al. Continuous porous aromatic framework membranes with modifiable sites for optimized gas separation. Angew Chem Int Ed Engl 2022;61:e202113682.
29. Yuan Y, Zhu G. Porous aromatic frameworks as a platform for multifunctional applications. ACS Cent Sci 2019;5:409-18.
30. Das S, Heasman P, Ben T, Qiu S. Porous organic materials: strategic design and structure-function correlation. Chem Rev 2017;117:1515-63.
31. Bai R, Song X, Yan W, Yu J. Low-energy adsorptive separation by zeolites. Natl Sci Rev 2022;9:nwac064.
32. Peng X, Vicent-Luna JM, Jin Q. Separation of CF4/N2, C2F6/N2, and SF6/N2 mixtures in amorphous activated carbons using molecular simulations. ACS Appl Mater Interfaces 2020;12:20044-55.
33. Sosa JE, Malheiro C, Ribeiro RP, et al. Adsorption of fluorinated greenhouse gases on activated carbons: evaluation of their potential for gas separation. J Chem Tech Biotech 2020;95:1892-905.
34. Choi SW, Yoon HJ, Lee HJ, Lee E, Lim D, Lee KB. CF4 adsorption on porous carbon derived from silicon carbide. Microporous Mesoporous Mater 2020;306:110373.
35. Zhu J, Hu J, Xiao H, et al. Aluminum-based metal organic frameworks for greenhouse gases CF4 and C2F6 capture with excellent capacity and selectivity. Sep Purif Technol 2024;331:125614.
36. Chuah CY, Goh K, Bae TH. Hierarchically structured HKUST-1 nanocrystals for enhanced SF6 capture and recovery. J Phys Chem C 2017;121:6748-55.
37. Kim MB, Lee SJ, Lee CY, Bae YS. High SF6 selectivities and capacities in isostructural metal-organic frameworks with proper pore sizes and highly dense unsaturated metal sites. Microporous Mesoporous Mater 2014;190:356-61.
38. Senkovska I, Barea E, Navarro JAR, Kaskel S. Adsorptive capturing and storing greenhouse gases such as sulfur hexafluoride and carbon tetrafluoride using metal-organic frameworks. Microporous Mesoporous Mater 2012;156:115-20.
39. Kim M, Kim K, Kim T, et al. Highly selective adsorption of SF6 over N2 in a bromine-functionalized zirconium-based metal-organic framework. Chem Eng J 2018;339:223-9.
40. Guo Y, Tan C, Sun J, Li W, Zhang J, Zhao C. Porous activated carbons derived from waste sugarcane bagasse for CO2 adsorption. Chem Eng J 2020;381:122736.
41. Jiang N, Shang R, Heijman SGJ, Rietveld LC. High-silica zeolites for adsorption of organic micro-pollutants in water treatment: a review. Water Res 2018;144:145-61.
42. Xu X, Megarajan SK, Zhang Y, Jiang H. Ordered mesoporous alumina and their composites based on evaporation induced self-assembly for adsorption and catalysis. Chem Mater 2020;32:3-26.
43. Wu J, Xu F, Li S, et al. Porous polymers as multifunctional material platforms toward task-specific applications. Adv Mater 2019;31:e1802922.
44. Chaoui N, Trunk M, Dawson R, Schmidt J, Thomas A. Trends and challenges for microporous polymers. Chem Soc Rev 2017;46:3302-21.
45. Farha OK, Yazaydın AÖ, Eryazici I, et al. De novo synthesis of a metal-organic framework material featuring ultrahigh surface area and gas storage capacities. Nat Chem 2010;2:944-8.
46. Comotti A, Castiglioni F, Bracco S, et al. Fluorinated porous organic frameworks for improved CO2 and CH4 capture. Chem Commun 2019;55:8999-9002.
47. Wu AX, Drayton JA, Mizrahi Rodriguez K, et al. Elucidating the role of fluorine content on gas sorption properties of fluorinated polyimides. Macromolecules 2021;54:22-34.
48. Feng L, Guo J, Zhong X, Sun Z. Fluorinated porous poly(spirobifluorene) via direct C-H arylation: characterization, porosity, and gas uptake. J Macromol Sci Part A 2014;51:604-9.
49. Boopalachandran P, Laane J. Vibrational spectra, structure, and theoretical calculations of 2-fluoro- and 3-fluoropyridine. Spectrochim Acta A Mol Biomol Spectrosc 2011;79:1191-5.
50. Al-Ghouti MA, Da’ana DA. Guidelines for the use and interpretation of adsorption isotherm models: a review. J Hazard Mater 2020;393:122383.
51. Bai X, Wang X, Lu X, et al. A fluorine induced enhancement of the surface polarization and crystallization of g-C3N4 for an efficient charge separation. New J Chem 2021;45:9334-45.
Cite This Article
How to Cite
Download Citation
Export Citation File:
Type of Import
Tips on Downloading Citation
Citation Manager File Format
Type of Import
Direct Import: When the Direct Import option is selected (the default state), a dialogue box will give you the option to Save or Open the downloaded citation data. Choosing Open will either launch your citation manager or give you a choice of applications with which to use the metadata. The Save option saves the file locally for later use.
Indirect Import: When the Indirect Import option is selected, the metadata is displayed and may be copied and pasted as needed.
About This Article
Special Issue
Copyright
Author Biographies
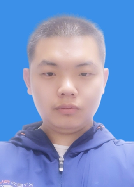
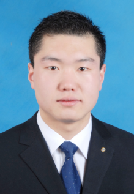
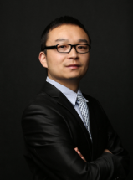
Comments
Comments must be written in English. Spam, offensive content, impersonation, and private information will not be permitted. If any comment is reported and identified as inappropriate content by OAE staff, the comment will be removed without notice. If you have any queries or need any help, please contact us at [email protected].