Microwave-pulse assisted synthesis of tunable ternary-doped 2D molybdenum carbide for efficient hydrogen evolution
Abstract
Amidst the urgent demand for carbon-neutral strategies, electrocatalytic hydrogen evolution reaction (HER) has garnered significant attention as an efficient and environmentally friendly energy conversion pathway. Non-precious metal layered transition metal carbides, particularly various modified two-dimensional molybdenum carbides (2D Mo2C), have emerged as promising HER catalysts due to their superior intrinsic catalytic activity. While common non-metal doping strategies have been widely employed to enhance the electronic configuration and bulk/interface activity, the mechanism of HER performance dependence on the doping-induced electronic configuration in 2D Mo2C remains unclear, especially for more complex binary or ternary doping configurations. To address the issue of uncontrollable doping atom percentages in conventional methods, herein, we propose a strategy for rapidly synthesizing highly tunable non-metal multielement-doped 2D Mo2C using microwave pulse-assisted synthesis. By designing doping configurations with similar atomic ratios, we delve into the impact mechanisms of various doping configurations on the HER performance of 2D Mo2C, with phosphorus doping potentially exerting the most significant positive influence. Furthermore, leveraging the unique thermodynamic and kinetic advantages of microwaves, this approach efficiently prevents potential side reactions associated with multi-element doping, enabling the rapid and precise synthesis of binary and ternary-doped 2D Mo2C. The synthesized ternary-doped 2D Mo2C with the same doping atomic ratios (2D P,N,S-Mo2C) exhibits outstanding HER performance. This method not only offers a novel approach for precisely designing non-metallic atomic doping configurations in 2D TMCs but also provides insights into the theoretical structure-activity mechanism for other carbides with unique structures.
Keywords
INTRODUCTION
Electrocatalytic hydrogen evolution reaction (HER), as an efficient and environmentally friendly energy conversion method, has gradually become one of the practical carbon-neutral strategies[1-3]. The widespread application of traditional high-activity Pt-group metal-based electrocatalysts is severely constrained by their high cost and low reserves[4]. Recently, non-precious metal two-dimensional (2D) layered transition metal carbides (TMCs), known as MXenes (Mn+1XnTx:M = early transition metal; X = C or N; Tx = surface termination), and their derivatives have been considered promising HER catalysts[5,6]. Especially 2D molybdenum carbide (2D Mo2C), with its high-density electronic states on the Fermi level d orbitals akin to Pt, can effectively enhance excellent intrinsic catalytic activity, while the 2D structure also exposes abundant active sites and facilitates rapid ion transport[7,8]. In order to effectively advance the practical hydrogen production application of 2D Mo2C, it is necessary to further modify and enhance its intrinsic catalytic activity and cycling performance while delving deeper into elucidating the structure-performance relationship in the context of structural engineering [Figure 1A]. Currently, non-metal doping, such as nitrogen doping (N-)[9,10], sulfur doping (S-)[11,12], phosphorus doping (P-)[13,14], fluorine doping (F-)[15,16], etc., as a common, straightforward, and efficient strategy to enhance the electronic configuration and bulk/interface activity of energy materials, is ubiquitously employed in various electrocatalytic applications[17-22]. This is primarily because the changes induced by doping, such as alterations in electronegativity, ionization potential, and electron affinity, significantly influence the electronic density and the interactions between molecular and atomic orbitals, thereby shaping the adsorption/desorption behavior of intermediates on the catalyst surface from both electronic configuration and crystal structure perspectives [Figure 1B and C]. For instance, Lv et al. demonstrated that N doping greatly altered the electronic configuration of hydrogen-substituted graphdiyne material, contributing to enhanced oxygen reduction reaction (ORR) performance[23]. Zheng et al. successfully introduced P doping to CoSe2 material, leading to a phase transition and improved HER performance[24]. Despite the effective alteration of material electronic configuration and interface properties through these non-metal doping processes, there remains a scarcity of reports on the specific impact mechanisms of non-metal doping on the electron configuration-dependent electrocatalytic performance, which holds true for 2D Mo2C. This challenge is primarily constrained by the difficulty of achieving precise control over the doping process using conventional methods. The slow entropy-change reaction processes, represented by solvothermal and high-temperature annealing approaches, make it challenging to attain transient on-off doping reactions, resulting in a random distribution of atomic content of non-metal elements in the final product. The uncontrollable nature of this electronic configuration scenario will significantly impede the elucidation of the structure-performance relationship mechanisms for HER characteristics in structural engineering.
Figure 1. Research significance. (A) Mechanism of 2D Mo2C hydrogen evolution reaction; (B) The reason of doping 2D Mo2C with P, N and S as an example; (C) Factors affecting electronic structure; (D) The inadequacy of traditional strategy. 2D Mo2C: Two-dimensional molybdenum carbides.
Unfortunately, compared to other HER electrocatalysts with lower synthesis temperatures, such as oxides, sulfides, and phosphides, TMCs typically undergo high-temperature reactions, making it even more challenging to achieve a delicate balance between one-step realization of 2D structural design and precise control of non-metal doping processes. Presently, mainstream methods such as etching MAX [Mn+1AXn
Actually, achieving a balance between the 2D structural design of molybdenum carbide and the precise control of non-metal doping involves a trade-off between the thermodynamics of 2D oriented growth and the kinetics of transient doping processes. Interestingly, over the past decade, advanced non-liquid-phase microwave technology has been able to fully utilize the dipolar, conduction, and magnetic losses to achieve rapid and ultra-high-energy conversion[33,34]. For example, Wan et al. demonstrated the rapid synthesis of high-quality graphene using microwave-assisted methods within tens of seconds[35]. Xie et al. showed a controllable thermal shock synthesis method based on microwave radiation, enabling the rapid preparation of uniform nanoparticles on graphene[36]. Hu et al. used microwave-assisted methods to achieve the 2D structure evolution of TMCs within 3 min[37]. Subsequently, they achieved precise control of metal element doping using microwave discharge[38-40]. These microwave techniques undoubtedly hold significant potential to balance the thermodynamics of 2D oriented growth of Mo2C with the kinetics of transient doping processes, successfully enabling the fabrication of various highly tunable non-metal-doped 2D Mo2C and comprehensively revealing its structure-performance relationship in the context of HER.
Herein, we propose a method for rapidly synthesizing highly tunable non-metal multi-doped 2D Mo2C using microwave pulse-assisted synthesis. Utilizing the transient on-off characteristics of microwave pulses, controlled doping of molybdenum carbide was achieved by representing the simplest elements, nitrogen, sulfur, and phosphorus, which exhibit distinct extranuclear electron distributions, striking a balance between 2D structural design and precise doping. By designing doping configurations with the same atomic ratio, the impact mechanisms of different types of doping configurations on the HER performance of 2D Mo2C can be comprehensively elucidated from both electronic configuration and crystal structure aspects, with the positive influence of P doping potentially being the most significant. Moreover, the unique thermodynamic and kinetic advantages of microwaves can effectively prevent side reactions during the doping process. The equiatomic ternary-doped 2D P,N,S-Mo2C exhibits excellent HER performance (overpotential of 58 mV at a current density of 10 mA·cm-2, Tafel value of 48 mV·dec-1). The strategy of precisely designing non-metallic atomic doping configurations for 2D TMCs holds the potential to propel the theoretical structure-activity analysis for other carbides with unique structures in electrocatalytic applications.
EXPERIMENTAL
Chemicals
All chemicals were commercially acquired and utilized as received without additional purification. The chemical compounds Na2MoO4·2H2O (CAS: 10102-40-6, purity: 99%, analytical reagent grade) and
Synthesis of 2D P,N,S-Mo2C
Initially, 6 mg NaH2PO4 (phosphorus source) and 12 mg Na2S·9H2O (sulfur source) were added to 1 mL deionized water under the condition that the non-metallic atomic ratio P:N:S = 1:1:1. Then, 60 mg C6H12O6 (carbon source), 206 mg Na2MoO4·2H2O (molybdenum source), 60 mg H2C2O4 (blowing agent) and 1.5 mg CH4N2O (nitrogen source) were added, fully mixed, and natural dried for 6 h to obtain a very uniform mixture. The evenly mixed drugs were transferred into a quartz tube with an outer diameter of 16 mm, inner diameter of 12 mm, wall thickness of 2 mm, and length of 60 mm. The tube was placed in a Grant G90F25CN3LN microwave oven for the reaction. First, nitrogen was injected into the reaction chamber for 10 min at a flow rate of 0.8 L/min. Subsequently, the sample was pulse heated three times for 1 min each with an output power of 900 W, under continuous nitrogen flow. After heating, the nitrogen flow was maintained until the apparatus cooled naturally to room temperature. Finally, the samples were taken out, rinsed with distilled water, and desiccated in a vacuum oven at 80 °C for 24 h to obtain 2D P,N,S-Mo2C.
Synthesis of other doped samples
The same method was used for preparing other doped samples, with the following modifications based on the dopant: A nitrogen-doped 2D Mo2C (2D N-Mo2C) sample was obtained by adding 1.5 mg CH4N2O as a nitrogen source. A phosphorus-doped 2D Mo2C (2D P-Mo2C) sample was obtained by adding 6 mg of NaH2PO4 as a phosphorus source. A sulfur-doped 2D Mo2C (2D S-Mo2C) sample was obtained by adding 12 mg of Na2S·9H2O as a sulfur source. A nitrogen and phosphorus-doped 2D Mo2C (2D P,N-Mo2C) sample was obtained by adding 1.5 mg CH4N2O as a nitrogen source and 6 mg NaH2PO4 as a phosphorus source, respectively. A phosphorus and sulfur-doped 2D Mo2C (2D P,S-Mo2C) sample was obtained by adding 6 mg NaH2PO4 as a phosphorus source and 12 mg Na2S·9H2O as a sulfur source, respectively. A nitrogen and sulfur-doped 2D Mo2C (2D N,S-Mo2C) sample was obtained by adding 1.5 mg CH4N2O as a nitrogen source and 12 mg of Na2S·9H2O as a sulfur source, respectively. All steps are conducted under specific conditions to ensure the balance between the 2D structure and precise doping.
Material characterization
The structural composition of the samples was analyzed using X-ray diffraction (XRD) with Cu Kα radiation on an Ultima IV X-ray powder diffractometer and X-ray photoelectron spectroscopy (XPS) using a Thermo Scientific K-Alpha instrument. Their morphologies were examined using high-resolution field emission scanning electron microscopy (FESEM) on a JSM-7800 F instrument (Japan) and transmission electron microscopy (TEM) on a JEM 2100F instrument (Japan). The thickness of 2D P,N,S-Mo2C was determined by atomic force microscopy (AFM) using a Bruker Dimension Icon instrument (Germany).
Electrochemical performance
All electrochemical experiments are conducted using rotating disk electrodes (RDEs) of the AFMSRCE model from PINE Instruments in the United States. The electrochemical experiments employed a three-electrode setup with a CHI Electrochemical Station (Model CHI660E, China). As the working electrode, a mirror-polished glassy carbon electrode with a diameter of 0.2 cm-2 was utilized, while the reference electrodes included Hg/HgO (1 M KOH) and Ag/AgCl (0.5 M H2SO4), and the counter electrode was a carbon rod. In a solvent mixture comprising 0.2 mL of deionized water, 0.8 mL of isopropanol, and 20 μL of Nafion (5%, mass fraction), 5 mg of catalyst powder was dispersed. Following that, the mixture underwent ultrasonication in an ice bath for an hour to achieve a homogeneous ink. Afterward, a mirror-polished glassy carbon electrode was drop-cast with 20 μL of the ink, with a catalyst loading of approximately
The frequency range for electrochemical impedance spectroscopy (EIS) testing was 0.1 to 105 Hz with an amplitude of 10 mV. Long-term durability tests were performed at 10 mA·cm-2. The mass activity (MA, A·g-1) values of the catalysts were calculated from the catalyst loading (m) and measured current density (i). MA = i/m.
RESULTS AND DISCUSSION
Material preparation and characterization
Non-metallic atom P, N, and S doping is a common, simple, and effective strategy to explore the effects of different electronegativity and atomic radius on the band gap, bonding properties, strength, and electron affinity, thus improving the electronic configuration, active sites, and conductivity. The changes in ionic radius, electronegativity, electron affinity, and ionization potential enable adjustments to the electronic structure. Doping with non-metallic heteroatom strategy introduces non-metallic heteroatom, changes electronegativity, electron affinity and ionization potential, and significantly affects the electron structure and local spatial configuration. For example, The P, N and S atoms have larger radii than the C atom, which can promote electron delocalization due to the formation of weaker covalent bonds. Utilizing the transient high-energy and high-temperature advantages of microwave pulses allows for rapid initiation and termination of the reaction, thereby enabling precise doping of foreign atoms into the host material. The ten-second interpulse pause is consistent with the rapid quenching process, and it is this rapid temperature change that makes the precise doping of non-metallic heteroatoms possible. This approach efficiently mitigates side reactions caused by gradual temperature gradients through rapid heating and cooling performance. This occurs because the quick temperature changes prevent prolonged exposure to intermediate temperature ranges, where unwanted reactions could occur. As a result, the overall reaction proceeds more smoothly and yields the desired products with higher purity. This enhancement in reaction selectivity and efficiency is particularly advantageous in complex chemical processes where precise control over reaction conditions is crucial for the desired outcome. The instantaneous temperature change of microwaves makes it possible to regulate heteroatom doping and 2D structure simultaneously. Because of this property of microwaves, non-metallic elements, which are inherently prone to doping reactions, can almost entirely participate in the reaction at a certain doping level without leakage. Thus, the precise doping of binary/ternary is realized and the 2D structure is constructed in one step.
Considering these factors, the experiment was devised. Figure 2 illustrates the schematic of the microwave-pulse-assisted synthesis of tunable Single/Binary/ternary-doped 2D Mo2C. This setup can operate under various gas conditions, ensuring the requirements of diverse microwave experiments. The detailed configuration of the experimental apparatus is depicted in Supplementary Figure 1. Sodium molybdate tetrahydrate is used as the metal precursor, glucose as the carbon source, oxalic acid as the blowing agent, urea, sodium phosphate and sodium sulfide 9-hydrate as dopants, while ensuring that the atomic ratio about of P:N:S:Mo is 1:1:1:17. It is worth noting that weighing the proportion of metal and non-metal atoms is not strictly consistent with the proportion calculation, because non-metal atoms may partially generate gas outflow during the reaction process, but the microwave pulse method will minimize the extent of this process. The mixture is ground uniformly and placed in a quartz crucible, which is then transferred to a custom microwave reactor surrounded by carbon nanotubes (CNTs). Nitrogen gas is introduced into the system, with its flow meticulously regulated by a flow meter. Real-time monitoring of temperature fluctuations within the reaction zone is achieved using an infrared detector. Throughout the microwave-induced reaction process, the high dielectric constant of CNTs induces dielectric loss, facilitating the rapid and uniform generation of thermal energy within the CNT region. This uniform heating condition is conducive to rapidly introducing non-metal elements and the expedited formation of 2D structures. After the microwave operation, the temperature drops rapidly, resulting in rapid cooling of the reactants, ensuring precise control of the P, N and S atom content. After three microwave pulses, highly tunable non-metallic doped 2D Mo2C was synthesized in one step striking a balance between kinetics and thermodynamics. In terms of kinetics, the 2D Mo2C materials doped with single, binary and ternary elements were successfully synthesized using microwave pulses. Regarding thermodynamics, 2D nanosheets were also successfully generated through this method. Utilizing the attributes of real-time controllable microwave pulses facilitates identifying products generated at different stages during the reaction process. Moreover, this capability enables on-site analysis of microwave-induced reactions. Three-dimensional (3D) optical microscopy (OM) was used to characterize the samples with 1, 2, and 3 pulses (T1, T2, and T3, respectively). The results showed three stages of reactants: (1) Polymerization; (2) Bubbling; (3) Burst[37,41-43]. In the initial stage, glucose in the reactants undergoes thermal decomposition and polymerizes into a gel-like polymer during the first pulse. Simultaneously, due to the thermal decomposition of oxalic acid, gas is generated, forming bubbles within the resulting polymer. During the second stage, the temperature inside the reaction chamber remains high for a period, increasing bubbles within the polymer. The release of a large number of bubbles causes the polymer to be blown into a 3D structure. In the third stage, with the extension of reaction time, the gas released by the high-temperature reaction disrupts the 3D bubble structure, generating a large number of 2D nanosheets. The optical images in the figure show that the sample undergoes nearly a seven-fold volume expansion compared to the precursor material after the reaction. Detailed preparation steps are described in the experimental section.
Figure 2. Schematic diagram of the influence of microwave pulse strategy on the morphology evolution and doping regulation of 2D Mo2C. 2D Mo2C: Two-dimensional molybdenum carbides.
The temperature change during the reaction was monitored using an infrared detector. As shown in Figure 3A, the reaction zone temperature rapidly reached a relatively stable temperature of 872 °C within
Figure 3. Morphology characterization. (A) Real-time temperature changes of samples heated by microwave pulses. The inset image is 10 s microwave discharge diagram; (B) SEM images of 2D P,N,S-Mo2C; (C) TEM images of 2D P,N,S-Mo2C; (D) The HRTEM image of 2D P,N,S-Mo2C and the insert image is the corresponding SAED pattern; (E) EDS elemental mapping scanning from TEM of single doping 2D Mo2C; (F) EDS elemental mapping scanning from TEM of binary doping 2D Mo2C; (G) EDS elemental mapping scanning from TEM of ternary doping 2D Mo2C. 2D: Two-dimensional; Mo2C: molybdenum carbides; SEM: scanning electron microscopy; TEM: transmission electron microscopy; HRTEM: high-resolution transmission electron microscopy; SAED: selected area electron diffraction; EDS: energy-dispersive X-ray spectroscopy.
Leveraging the unique attributes of microwave pulse assistance, we can characterize the structural evolution of the samples at different stages of the reaction. The XRD data were refined using General Structure Analysis System (GSAS) software [Figure 4A]. The parameters of the synthesized catalyst were calculated, and the changes related to atomic doping were revealed. As shown in Supplementary Table 1, the lattice parameters increase from 3.0124 Å, 3.0124 Å, 4.7352 Å to 3.0150 Å, 3.0150 Å and 4.7476 Å, respectively. The lattice size of 2D P,N,S-Mo2C is larger than pristine Mo2C. This is because the atomic radius of C is different from that of P, N, and S atoms, suggesting that various types of non-metallic heteroatoms have been successfully incorporated into the lattice of Mo2C. As shown in Supplementary Figure 5, the XRD data for samples subjected to one, two, and three microwave pulses clearly demonstrate the structural evolution of 2D P,N,S-Mo2C. After the first pulse, the XRD spectrum between 15° and 30° shows a broad diffraction peak, possibly due to the thermal decomposition of glucose resulting in a large amount of carbon. Following the second pulse, the XRD features are predominantly those of monoclinic MoO2 (PDF: 01-076-1807)[44]. The formation of Mo2C single crystal phase is observed after the third pulse. The diffraction peaks of the obtained 2D P,N,S-Mo2C at 34.3, 37.9, 39.3, 52.1, 61.5, 69.5, 72.3, 74.6, and 75.5, when compared with the collection code (023823) from the Inorganic Crystal Structure Database (ICSD), correspond to the hexagonal phase Mo2C (PDF: 00-035-0787) planes of (100), (002), (101), (102), (110), (103), (200), (112), and (201), respectively[45]. Doping does not cause obvious change of crystal structure, suggesting that the microwave-pulse-assisted synthesis of 2D P,N,S-Mo2C does not produce any by-products.
Figure 4. Structural characterization. (A) Difference patterns for the Rietveld refinement from the XRD of 2D P,N,S-Mo2C; (B-E) High-resolution XPS spectra of Mo 3d, p 2p, N 1s and S 2p of 2D P,N,S-Mo2C; (F) Summary of the proportion of each heteroatom in 2D doped-Mo2C. XRD: X-ray diffraction; 2D: two-dimensional; Mo2C: molybdenum carbides; XPS: X-ray photoelectron spectroscopy.
Subsequently, XPS was employed to investigate the oxidation states of elements in the non-metal-doped 2D Mo2C samples and the bonding of surface elements in depth. As depicted in Figure 4B, the high-resolution Mo 3d spectrum can be resolved into four doublets. The two prominent peaks at 228.6 and 232.0 eV correspond to Mo2+ (Mo 3d3/2 and Mo 3d5/2), indicating Mo2C bonds. Concurrently, the presence of Mo3+ peaks at 229.0 and 232.8 eV suggests potential P−Mo and N−Mo bonding. The presence of Mo3+ indicates that P or N occupies part of the C position in Mo2C, which may optimize its electronic properties and reduce the desorption energy of the Mo−H bond. The valence states of Mo4+ (230.4 and 233.8 eV) and Mo6+ (233.1 and 236.2 eV) may be associated with the inevitable surface oxidation of Mo exposed to air[46]. In the C 1s spectrum, the peak at 284.0 eV, attributed to the Mo−C bond, further confirms the formation of the Mo2C phase. The peaks at 288.8, 286.6, 285.4, and 284.7 eV correspond to O−C=O, C=O, C−O and C−C bonds, respectively[47,48] [Supplementary Figure 6]. The presence of oxygen groups (O−C=O) is beneficial for enhancing the wettability of the catalyst to the electrolyte[49]. The peaks at 129.4 and 130.4 eV in the P 2p spectrum correspond to the P 2p3/2 and p 2p1/2, respectively. [Figure 4C]; all these peaks are associated with the P−Mo bonds after doping. The peak at 133.3 eV corresponds to the P−C bond, while the peak at
Hydrogen evolution performance test
By designing a similar doping atom ratio, the influence of P, N, S doping on electron configuration of non-metallic atoms with different electronegativity and atomic radii can be analyzed. Electrochemical testing can systematically investigate the HER performance mechanism dependent on various electron configurations. In acidic media, HER can be divided into two steps[52]: firstly, the adsorption of H+, known as the Volmer reaction (H3O+ + e- → H*ads + H2O), followed by the desorption step, known as the Heyrovsky reaction (H*ads + H3O+ + e- → H2 + H2O), or the recombination step, known as the Tafel reaction (H*ads + H*ads → H2). Therefore, a large amount of electron and ion transfer is involved, and 2D materials can provide an abundant active site for HER reaction and rapid electron and ion transfer speed [Figure 5A].
Figure 5. Synthesis of doped 2D Mo2C in 0.5 M H2SO4 electrocatalytic HER by microwave pulse method. (A) Schematic diagram of HER reaction for rapid electron and ion transport 2D P,N,S-Mo2C nanosheets; (B) Polarization curve; (C) Overpotentials at 10 mA·cm-2; (D) The corresponding Tafel slope; (E) Plotting current density variation against scan rate to fit linear regression allows for the estimation of Cdl; (F) Mass activity of various spinels calculated at -0.1 V vs. RHE; (G) Summary of statistical electrochemical properties; (H) Chronopotentiometry test at the current density of 10 mA·cm-1; (I) The accelerated durability tests of 2D P,N,S-Mo2C. 2D: Two-dimensional; Mo2C: molybdenum carbides; HER: hydrogen evolution reaction; RHE: reversible hydrogen electrode.
Figure 5B shows the polarization curves of 2D Mo2C, 2D P-Mo2C, 2D N-Mo2C, 2D S-Mo2C, 2D P,N-Mo2C, 2D P,S-Mo2C, 2D N,S-Mo2C and 2D P,N,S-Mo2C s in 0.5 M H2SO4. To provide a benchmark for comparison, the electrocatalytic activity of a commercial Pt/C catalyst was measured under identical conditions. The HER potential corresponding to a current density of 10 mA·cm-2 is plotted in Figure 5C. Notably, At a current density of 10 mA·cm-2, the overpotential of 2D P,N,S-Mo2C is 58 mV, which is close to that of Pt/C (50 mV) and surpasses 2D P-Mo2C (86 mV), 2D N-Mo2C (88 mV), 2D S-Mo2C (90 mV), 2D P,N-Mo2C (67 mV), 2D P,S-Mo2C (74 mV) , 2D N,S-Mo2C (83 mV) and much better than 2D Mo2C
Additionally, the electrocatalytic HER performance of Pt/C, 2D Mo2C, 2D P-Mo2C, 2D N-Mo2C,
Figure 6. Synthesis of doped 2D Mo2C in 1 M KOH electrocatalytic HER by microwave pulse method. (A) Polarization curve; (B) The corresponding Tafel slope; (C) Plotting current density variation against scan rate to fit linear regression allows for the estimation of Cdl; (D) Mass activity of various spinels calculated at -0.1 V vs. RHE; (E) Summary of statistical electrochemical properties; (F) Chronopotentiometry test at the current density of 10 mA·cm-2; (G) The accelerated durability tests of 2D P,N,S-Mo2C; (H) The 2D P,N,S-Mo2C was compared with the overpotential and Tafel of other recently reported doped Mo2C-based electrocatalysts. 2D: Two-dimensional; Mo2C: molybdenum carbides; HER: hydrogen evolution reaction; RHE: reversible hydrogen electrode.
CONCLUSIONS
In summary, we propose a method for the one-step synthesis of highly tunable non-metal-doped 2D Mo2C using microwave pulses. This strategy utilizes the transient start-stop characteristics of microwave pulses to address the challenge of uncontrollable doping atom percentages in conventional approaches. Subsequently, the successful construction of 2D Mo2C doped with different typical non-metal elements at similar concentrations enabled a comprehensive elucidation of the specific impact mechanisms of non-metal doping on the electronic configuration-dependent electrocatalytic performance of 2D Mo2C. Among them, phosphorus doping configurations may have the greatest positive impact, and the equiatomic 2D
DECLARATIONS
Acknowledgments
The authors acknowledge financial support from the National Natural Science Foundation of China (No. 52203070) and the Open Fund of Hubei Key Laboratory of Biomass Fiber and Ecological Dyeing and Finishing (No. STRZ202203). Wan J expresses gratitude for the financial support provided by the China Scholarship Council (CSC) Visiting Scholar Program.
Authors’ contributions
Contributed equally to this work and conceptualization: Fan M, Guo J, Fang G
Data curation and Writing - original draft: Fan M, Guo J
Formal analysis: Fan M, Fang G, Tian H, You Y
Software: Fang G, Huang Z, Huang J
Funding acquisition: Wan J, Xu W, Jiang H
Supervision, validation and writing - review and editing: Wan J
Availability of data and materials
Supporting Information is available from the corresponding author upon reasonable request.
Financial support and sponsorship
This work was supported by the National Natural Science Foundation of China (No. 52203070) and the Open Fund of Hubei Key Laboratory of Biomass Fiber and Ecological Dyeing and Finishing (No. STRZ202203). Wan J expresses gratitude for the financial support provided by the China Scholarship Council (CSC) Visiting Scholar Program.
Conflicts of interest
All authors declared that there are no conflicts of interest.
Ethical approval and consent to participate
Not applicable.
Consent for publication
Not applicable.
Copyright
© The Author(s) 2024.
Supplementary Materials
REFERENCES
1. Yu H, Wan J, Goodsite M, Jin H. Advancing direct seawater electrocatalysis for green and affordable hydrogen. One Earth 2023;6:267-77.
2. Liu W, Niu X, Tang J, et al. Energy-efficient anodic reactions for sustainable hydrogen production via water electrolysis. Chem Synth 2023;3:44.
3. Wang J, Xu F, Jin H, Chen Y, Wang Y. Non-noble metal-based carbon composites in hydrogen evolution reaction: fundamentals to applications. Adv Mater 2017;29:1605838.
4. Pang J, Sun J, Zheng M, Li H, Wang Y, Zhang T. Transition metal carbide catalysts for biomass conversion: a review. Appl Catal B Environ 2019;254:510-22.
5. VahidMohammadi A, Rosen J, Gogotsi Y. The world of two-dimensional carbides and nitrides (MXenes). Science 2021;372:eabf1581.
6. Jin H, Song T, Paik U, Qiao S. Metastable two-dimensional materials for electrocatalytic energy conversions. Acc Mater Res 2021;2:559-73.
7. Peng X, Chen L, Liu Y, et al. Strain engineering of two-dimensional materials for energy storage and conversion applications. Chem Synth 2023;3:47.
8. Jin H, Gu Q, Chen B, et al. Molten salt-directed catalytic synthesis of 2d layered transition-metal nitrides for efficient hydrogen evolution. Chem 2020;6:2382-94.
9. Lu C, Tranca D, Zhang J, et al. Molybdenum carbide-embedded nitrogen-doped porous carbon nanosheets as electrocatalysts for water splitting in alkaline media. ACS Nano 2017;11:3933-42.
10. Peng F, Zhang L, Jiang B, et al. In-situ synthesis of microflower composed of N-doped carbon films and Mo2C coupled with Ni or FeNi alloy for water splitting. Chem Eng J 2022;427:131712.
11. Ma G, Ning G, Wei Q. S-doped carbon materials: synthesis, properties and applications. Carbon 2022;195:328-40.
12. Ma X, Ning G, Wang Y, et al. S-doped mesoporous graphene microspheres: a high performance reservoir material for Li S batteries. Electrochim Acta 2018;269:83-92.
13. Niu J, Dai P, Qi G, et al. Preparation of P doped TiO2 nanotubes and its photocatalytic activity. Integr Ferroelectr 2016;176:150-9.
14. Li C, Jang H, Liu S, et al. P and Mo dual doped Ru ultrasmall nanoclusters embedded in P-doped porous carbon toward efficient hydrogen evolution reaction. Adv Energy Mater 2022;12:2200029.
15. Wan J, Zhang G, Jin H, et al. Microwave-assisted synthesis of well-defined nitrogen doping configuration with high centrality in carbon to identify the active sites for electrochemical hydrogen peroxide production. Carbon 2022;191:340-9.
16. Lu F, Zhou Y, Liu J, Pan Y. Enhancement of F-doping on the electrochemical behavior of carbon-coated LiFePO4 nanoparticles prepared by hydrothermal route. Electrochim Acta 2011;56:8833-8.
17. Joucken F, Tison Y, Le Fèvre P, et al. Charge transfer and electronic doping in nitrogen-doped graphene. Sci Rep 2015;5:14564.
18. Wang Y, Niu Y, Pu Y, Li S, Liu Y, Zhang B. Revealing the dynamic formation mechanism of porous Mo2C: an in-situ TEM study. Chem Synth 2023;3:42.
19. Jia Y, Zhang L, Zhuang L, et al. Identification of active sites for acidic oxygen reduction on carbon catalysts with and without nitrogen doping. Nat Catal 2019;2:688-95.
20. de la Torre B, Švec M, Hapala P, et al. Non-covalent control of spin-state in metal-organic complex by positioning on N-doped graphene. Nat Commun 2018;9:2831.
21. Hasegawa G, Deguchi T, Kanamori K, et al. High-level doping of nitrogen, phosphorus, and sulfur into activated carbon monoliths and their electrochemical capacitances. Chem Mater 2015;27:4703-12.
22. Liang HW, Zhuang X, Brüller S, Feng X, Müllen K. Hierarchically porous carbons with optimized nitrogen doping as highly active electrocatalysts for oxygen reduction. Nat Commun 2014;5:4973.
23. Lv Q, Si W, He J, et al. Selectively nitrogen-doped carbon materials as superior metal-free catalysts for oxygen reduction. Nat Commun 2018;9:3376.
24. Zheng YR, Wu P, Gao MR, et al. Doping-induced structural phase transition in cobalt diselenide enables enhanced hydrogen evolution catalysis. Nat Commun 2018;9:2533.
25. Jin S, Guo Y, Wang J, Wang L, Hu Q, Zhou A. Carbon dioxide adsorption of two-dimensional Mo2C MXene. Diam Relat Mater 2022;128:109277.
26. Tao Q, Dahlqvist M, Lu J, et al. Two-dimensional Mo1.33C MXene with divacancy ordering prepared from parent 3D laminate with in-plane chemical ordering. Nat Commun 2017;8:14949.
27. Khazaei M, Arai M, Sasaki T, Estili M, Sakka Y. The effect of the interlayer element on the exfoliation of layered Mo2AC (A = Al, Si, P, Ga, Ge, As or In) MAX phases into two-dimensional Mo2C nanosheets. Sci Technol Adv Mater 2014;15:014208.
28. Buke GC, Caylan OR, Ogurtani OT. Growth mechanism of 2D Mo2C on Cu via CVD. Cryst Growth Des 2023;23:5462-8.
29. Geng D, Zhao X, Chen Z, et al. Direct synthesis of large-area 2D Mo2C on in situ grown graphene. Adv Mater 2017;29:1700072.
30. Xu C, Wang L, Liu Z, et al. Large-area high-quality 2D ultrathin Mo2C superconducting crystals. Nat Mater 2015;14:1135-41.
31. Xie H, Feng Y, He X, et al. Construction of nitrogen-doped biphasic transition-metal sulfide nanosheet electrode for energy-efficient hydrogen production via urea electrolysis. Small 2023;19:e2207425.
32. Sun A, Shen Y, Wu Z, Wang D. N-doped MoP nanoparticles for improved hydrogen evolution. Int J Hydrogen Energy 2017;42:14566-71.
33. Jiang H, Xian J, Hu R, et al. Microwave discharge for rapid introduction of bimetallic-synergistic configuration to conductive catecholate toward long-term supercapacitor. Chem Eng J 2023;455:140804.
34. Jiang H, Li J, Xiao Z, et al. The rapid production of multiple transition metal carbides via microwave combustion under ambient conditions. Nanoscale 2020;12:16245-52.
35. Wan J, Huang L, Wu J, et al. Microwave combustion for rapidly synthesizing pore-size-controllable porous graphene. Adv Funct Mater 2018;28:1800382.
36. Xie H, Liu N, Zhang Q, et al. A stable atmospheric-pressure plasma for extreme-temperature synthesis. Nature 2023;623:964-71.
37. Hu R, Jiang H, Xian J, et al. Microwave-pulse sugar-blowing assisted synthesis of 2D transition metal carbides for sustainable hydrogen evolution. Appl Catal B Environ 2022;317:121728.
38. Xian J, Jiang H, Wu Z, et al. Microwave shock motivating the Sr substitution of 2D porous GdFeO3 perovskite for highly active oxygen evolution. J Energy Chem 2024;88:232-41.
39. Fang G, Liu K, Fan M, et al. Unveiling the electron configuration-dependent oxygen evolution activity of 2D porous Sr-substituted LaFeO3 perovskite through microwave shock. Carbon Neutralization 2023;2:709-20.
40. Hu R, Wei L, Xian J, et al. Microwave shock process for rapid synthesis of 2D porous La0.2Sr0.8CoO3 perovskite as an efficient oxygen evolution reaction catalyst. Acta Phys Chim Sin 2023;39:2212025.
41. Han K, Liu Z, Li P, et al. High-throughput fabrication of 3D N-doped graphenic framework coupled with Fe3C@porous graphite carbon for ultrastable potassium ion storage. Energy Storage Mater 2019;22:185-93.
42. Luo Y, Liu K, Jin H, Wang Z, Dai S, Huang L. Blowing ultrathin 2D materials. Adv Mater Inter 2023;10:2202239.
43. Wang X, Zhang Y, Zhi C, et al. Three-dimensional strutted graphene grown by substrate-free sugar blowing for high-power-density supercapacitors. Nat Commun 2013;4:2905.
44. Zhou E, Wang C, Shao M, Deng X, Xu X. MoO2 nanoparticles grown on carbon fibers as anode materials for lithium-ion batteries. Ceram Int 2017;43:760-5.
45. Sun J, Chen X, Mao Z, Liu B, Zhao R, Du J. Fabrication of Mo2C nanoparticles on N-doped carbon nanosheets as high-performance electrocatalyst. J Alloys Compd 2023;934:167931.
46. Wang D, Liu T, Wang J, Wu Z. N, P (S) Co-doped Mo2C/C hybrid electrocatalysts for improved hydrogen generation. Carbon 2018;139:845-52.
47. Yu B, Yang D, Hu Y, He J, Chen Y, He W. Mo2C nanodots anchored on N-doped porous CNT microspheres as electrode for efficient Li-ion storage. Small Methods 2019;3:1800287.
48. Fan J, Wu X, Piñeiro-García A, et al. β-Mo2C nanoparticles produced by carburization of molybdenum oxides with carbon black under microwave irradiation for electrocatalytic hydrogen evolution reaction. ACS Appl Nano Mater 2021;4:12270-7.
49. Zuo P, Liu Y, Liu X, Jiao W, Wang R. N, P-codoped molybdenum carbide nanoparticles loaded into N, P-codoped graphene for the enhanced electrocatalytic hydrogen evolution. Int J Hydrogen Energy 2022;47:29730-40.
50. Geng W, Han H, Liu F, Liu X, Xiao L, Wu W. N,P,S-codoped C@nano-Mo2C as an efficient catalyst for high selective synthesis of methanol from CO2 hydrogenation. J CO2 Util 2017;21:64-71.
51. Wang Q, Yu R, Shen D, et al. One-pot synthesis of Mo2C&MoS2 loaded on N/S co-doped carbon materials as the electrocatalyts for hydrogen evolution reaction. Fuel 2022;318:123615.
52. Wan J, Wu J, Gao X, et al. Structure confined porous Mo2C for efficient hydrogen evolution. Adv Funct Mater 2017;27:1703933.
53. Zhao S, Lu X, Wang L, Gale J, Amal R. Carbon-based metal-free catalysts for electrocatalytic reduction of nitrogen for synthesis of ammonia at ambient conditions. Adv Mater 2019;31:1805367.
54. Jiao Y, Zheng Y, Davey K, Qiao S. Activity origin and catalyst design principles for electrocatalytic hydrogen evolution on heteroatom-doped graphene. Nat Energy 2016;1:16130.
55. Zhang Y, Yun S, Dang J, et al. Defect engineering via ternary nonmetal doping boosts the catalytic activity of ZIF-derived carbon-based metal-free catalysts for photovoltaics and water splitting. Mater Today Phys 2022;27:100785.
56. Chang Y, Tseng C, Lee C, et al. N- and S-codoped graphene hollow nanoballs as an efficient Pt-free electrocatalyst for dye-sensitized solar cells. J Power Sources 2020;449:227470.
57. Pei Z, Li H, Huang Y, et al. Texturing in situ: N,S-enriched hierarchically porous carbon as a highly active reversible oxygen electrocatalyst. Energy Environ Sci 2017;10:742-9.
58. Wang X, Xia L, Guo C, et al. Interfacial engineering of N, S-doped Mo2C-Mo/C heterogeneous nanorods for enhanced alkaline hydrogen evolution. Appl Surf Sci 2023;614:156276.
59. Cai J, Song Y, Zang Y, et al. N-induced lattice contraction generally boosts the hydrogen evolution catalysis of P-rich metal phosphides. Sci Adv 2020;6:eaaw8113.
60. Xu J, Ge L, Zhou Y, et al. Insights into N, P, S multi-doped Mo2C/C composites as highly efficient hydrogen evolution reaction catalysts. Nanoscale Adv 2020;2:3334-40.
61. Ji L, Wang J, Teng X, Dong H, He X, Chen Z. N,P-doped molybdenum carbide nanofibers for efficient hydrogen production. ACS Appl Mater Interfaces 2018;10:14632-40.
62. Shi Z, Nie K, Shao Z, et al. Phosphorus-Mo2C@carbon nanowires toward efficient electrochemical hydrogen evolution: composition, structural and electronic regulation. Energy Environ Sci 2017;10:1262-71.
Cite This Article
How to Cite
Fan, M.; Guo J.; Fang G.; Tian H.; You Y.; Huang Z.; Huang J.; Jiang H.; Xu W.; Wan J. Microwave-pulse assisted synthesis of tunable ternary-doped 2D molybdenum carbide for efficient hydrogen evolution. Chem. Synth. 2024, 4, 36. http://dx.doi.org/10.20517/cs.2023.72
Download Citation
Export Citation File:
Type of Import
Tips on Downloading Citation
Citation Manager File Format
Type of Import
Direct Import: When the Direct Import option is selected (the default state), a dialogue box will give you the option to Save or Open the downloaded citation data. Choosing Open will either launch your citation manager or give you a choice of applications with which to use the metadata. The Save option saves the file locally for later use.
Indirect Import: When the Indirect Import option is selected, the metadata is displayed and may be copied and pasted as needed.
About This Article
Copyright
Author Biographies
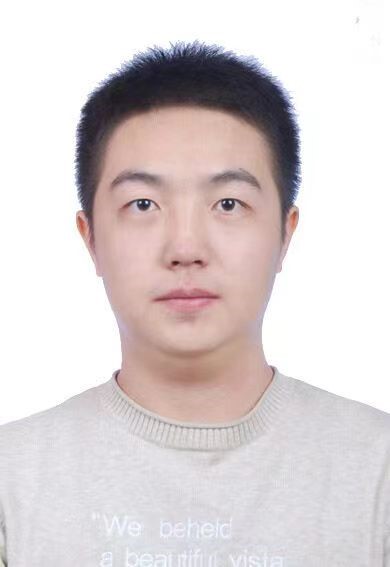
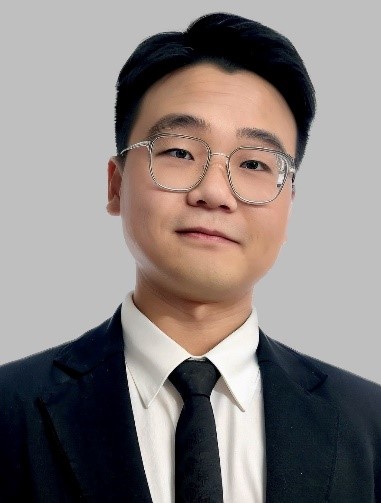
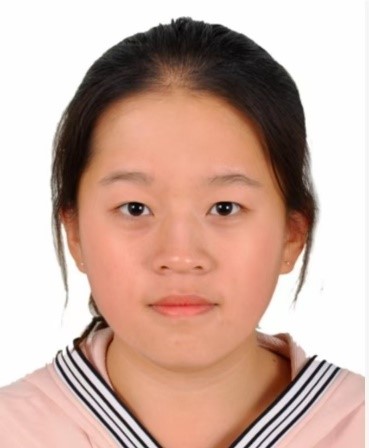
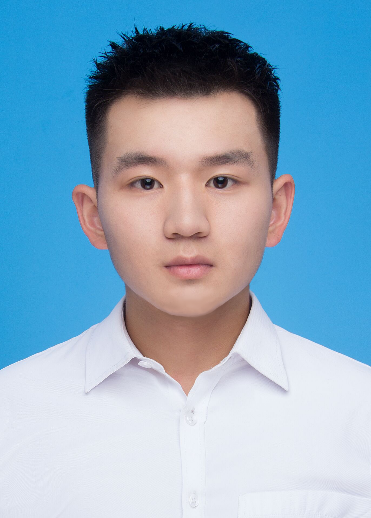
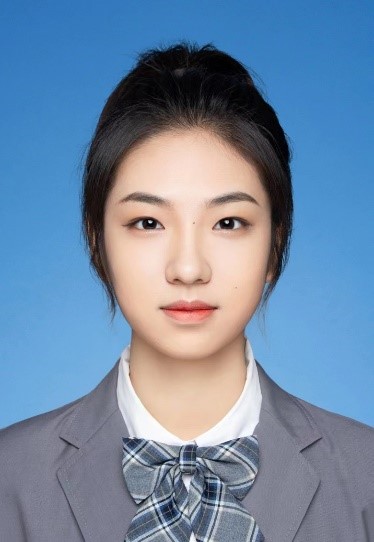
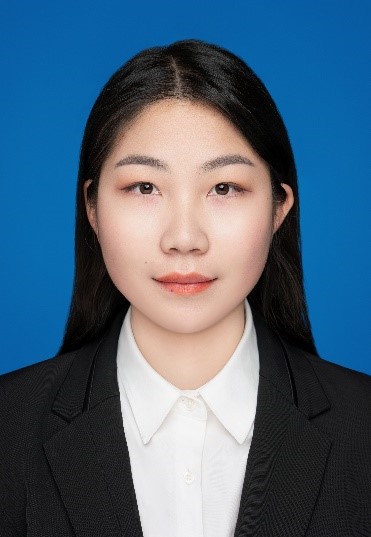
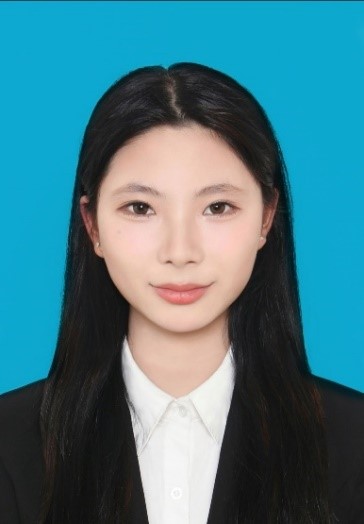
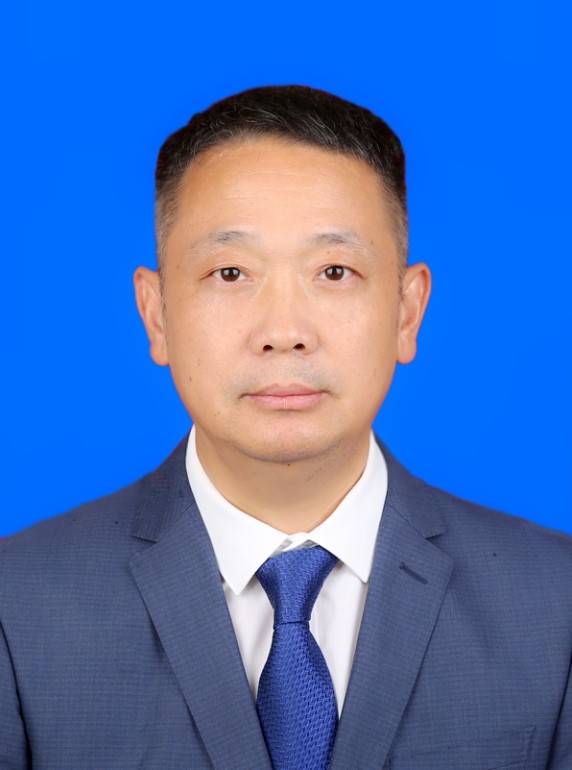
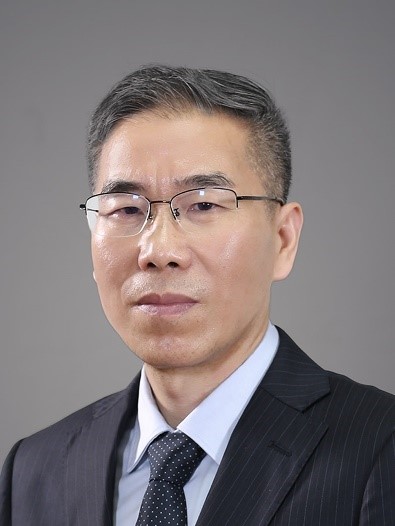
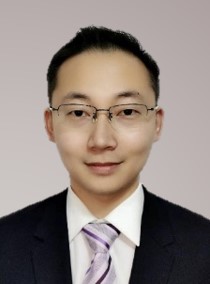
Comments
Comments must be written in English. Spam, offensive content, impersonation, and private information will not be permitted. If any comment is reported and identified as inappropriate content by OAE staff, the comment will be removed without notice. If you have any queries or need any help, please contact us at support@oaepublish.com.