A novel structure Ti/Fe2O3/Cu2S/Co(OH)x enhances the photoelectrochemical water splitting performance of iron oxide
Abstract
The slow oxygen evolution kinetics of iron oxide nanorod arrays have limited their applications in photocatalytic water splitting. Herein, we introduce p-type semiconductor cuprous oxide and further cover cobalt hydroxide ultrathin nanosheets on the surface of both by electrochemical deposition; these methods obviously enhanced the photoelectrochemical (PEC) water splitting performance of iron oxide nanorods on titanium sheet substrate. The photocurrent of this heterostructure reached 4.8 mA/cm2 at 1.23 V (vs. reversible hydrogen electrode) in a 1 M KOH aqueous solution under AM 1.5G illumination, which is much higher than the currently reported photocatalytic water splitting performance of iron oxide nanoarrays. The construction of Fe2O3/Cu2S p-n heterojunction accelerates the separation of photogenerated carriers in the main body of Fe2O3 nanorod arrays; as an excellent oxygen evolution catalyst (OEC), the introduction of Co(OH)x accelerates the kinetic process of interfacial water oxidation leading to the rapid depletion of photogenerated holes, which further improves the charge separation on the photoanode surface. Thus, the synergistic effect between Fe2O3/Cu2S p-n heterojunctions and oxygen evolution catalysts enhanced the iron oxide nanorod array photoanodes.
Keywords
INTRODUCTION
With economic and social development, the energy and environmental crises are increasingly becoming a constraint on human progress[1], but the photoelectrochemical (PEC) water splitting into hydrogen[2] offers a promising solution that exploits nature’s most abundant solar energy to decompose the earth’s vastly more abundant water resources into clean, environmentally friendly, and highly energy-dense hydrogen[3]. In 1972, Fujishima and Honda demonstrated the potential of titanium dioxide (TiO2)[4] semiconductor materials in splitting water into hydrogen and oxygen in photochemical cells[5].
Many highly active photoanodes have been reported, such as a monoclinic tungsten trioxide (WO3) nanowire by Nayak et al.[6]. The PEC activity of this nanowire was about 21 times higher than that of
Among the numerous semiconductor photoelectric anode materials, hematite[7] (α-Fe2O3) has become one of the most widely applied photoanode materials at this stage because of its excellent stability and environmental friendliness[8,9]. Fe2O3 has a band gap (Eg) of 1.9 to 2.2 eV[10]. Therefore, its light absorption range exceeds 600 nm, and it has sufficient visible light absorption[11]; theoretical calculations show that an ideal Fe2O3 photoanode can achieve up to 12.6 mA/cm2 at 1.23 V [vs. reversible hydrogen electrode (RHE)] under 100 mW/cm2 (AM 1.5G) of solar radiation[12]. However, the actual performance of Fe2O3 in PEC cells falls significantly below the theoretical value due to fast carrier complexation and a short hole diffusion length (only 2-4 nm), which severely affects its practical application[8]. Various modification strategies have been used to alleviate the shortcomings of hematite, such as doping[13], homojunction[14]/heterojunction[15] formation, and surface modification[16].
Cu2S is a widely utilized p-type semiconductor commonly employed in solar energy conversion[17]. It has an excellent ability to absorb light utilizing a wide range of wavelengths[18], while its high conductivity permits efficient photogenerated charge transfer[19]. A recent report has shown that the heterostructure formed by Fe2O3 and Cu2S exhibits enhanced photocatalytic activity under oxygen evolution reaction (OER). The enhanced activity is attributed to the formation of covalent bonds between Cu2S and Fe2O3, particularly the S–O bonds[20].
The construction of p-n junctions has been shown to optimize the PEC performance of photoanode arrays[21]. The built-in electric field facilitates the rapid separation of photogenerated carriers at the p-n junction[22] interface. It reduces bulk phase complexation, thereby enhancing the PEC water-splitting performance of the photoanode[23]. For instance, using the Ar plasma-assisted strategy, Meng et al. fabricated a SnS2 nanosheet array with numerous surface holes[24]. The reduction property of Ar atoms led to a partial reduction of Sn4+ to Sn2+, forming a SnS/SnS2 p-n junction of the nanosheet array. This facilitates carrier separation and prolongs carrier lifetime. As a result, the photocurrent density of this nanosheet array was 2.15 mA/cm2. In addition, Wu et al. also synthesized atomic sandwiched nitrogen-doped SnS2 nanosheets (p-n-p-SnS2) by controlled NH3 treatment[25]. The best sample achieved an optical current density of 3.28 mA/cm2. The outstanding performance can be attributed to the formation of p-n-p junctions that facilitate electron-hole transfer and optimize the kinetics of the oxygen precipitation reaction. Notably, loaded oxygen precipitation catalysts [oxygen evolution catalysts (OECs)] are considered a particularly effective method for enhancing the surface reaction kinetics of α-Fe2O3 membranes[26]. The OECs used in PEC systems are usually derived from superior electrocatalysts, and many of these low-cost transition-metal-based OECs have been shown to be able to achieve low overpotentials for electrochemical OER[27].
The construction of ternary photocatalytic or PEC materials has been widely studied. For example, Yang
However, simultaneous improvement in charge separation and surface oxygen precipitation kinetics at
Herein, we grew an array of Fe2O3 nanorods on titanium sheets using a hydrothermal method, which was used as a photoanode named Ti/Fe2O3. Further, we introduced a p-type semiconductor cuprous sulfide to form a p-n heterojunction photoanode (named Ti/Fe2O3/Cu2S), which significantly enhanced the PEC water-oxidation performance of the photoanode. In addition, the surfaces of both were further covered with ultrathin nanosheets of cobalt hydroxide by electrochemical deposition, which also significantly improved the PEC water decomposition performance of the Ti/Fe2O3/Cu2S photoanode. The photocurrent of this heterostructure [named Ti/Fe2O3/Cu2S/Co(OH)x] reached 4.8 mA/cm2 at 1.23 V (vs. RHE) in 1 M KOH aqueous solution at AM 1.5G, which is much higher than the PEC water splitting performance of the Fe2O3 nanoarrays reported so far. The substantial improvement of the catalytic performance is mainly attributed to two points: one is that the introduction of Fe2O3/Cu2S p-n heterojunction accelerates the separation of photogenerated carriers in the Fe2O3 nanorod arrays; the other is that the addition of OEC on the Co(OH)x surface accelerates the kinetics of the interfacial water oxidation, and the rapid depletion of the photogenerated holes further improves the photo-anodic surface charge separation. Therefore, the synergistic effect between Fe2O3/Cu2S p-n heterojunction and OEC significantly enhances the PEC water decomposition performance of the iron oxide nanorod array photoanode.
EXPERIMENTAL
Materials and synthesis methods
All chemical reagents used were analytically pure and underwent no further refinement during the experiment, including titanium foil, concentrated hydrochloric acid, acetone (C3H6O), ethanol absolute
Preparation of Ti/Fe2O3 photoanode
The Ti/Fe2O3 nanorod photoelectrode was prepared by a simple hydrothermal method[7] [Figure 1]. Firstly, 182.4 mg of FeCl3·6H2O and 383 mg of NaNO3 were dissolved in 4.5 mL of ultrapure water, and the resulting solution was stirred continuously for 2 h before adding 20 μL concentrated hydrochloric acid. Next, the prepared mixed solution of 0.15 M FeCl3 and 1 M NaNO3 was transferred to the stainless steel autoclave lined with polytetrafluoroethylene, and the titanium sheet was also immersed in the solution. After that, the autoclave was put into the oven and heated at 100 °C (1 °C/min) for 5 h. After the reaction, the titanium sheet was deposited with a uniform yellow β-FeOOH layer. Finally, the substrate was washed with deionized water to remove residual FeOOH and calcined at 550 °C (2 °C/min) for 2 h to obtain a sample called Ti/Fe2O3. The titanium sheets used in the hydrothermal reaction were ultrasonically cleaned in advance with a mixture of hydrochloric acid, ethanol acetone, and deionized water for 0.5 h to remove organic pollutants and dust.
Preparation of Ti/Fe2O3/Cu2S photoanode
According to previous reports, Ti/Fe2O3/Cu2S heterostructures were synthesized using the successive ion layer adsorption and reaction (SILAR) method[18] [Figure 1]. The α-Fe2O3 nanorod photoelectrode was first immersed in a CuCl-saturated solution for 60 s, which allowed Cu+ to adsorb on the surface of the α-Fe2O3 nanorod photoelectrode. Then, the photoelectrode was rinsed in deionized water for 60 s to prevent excessive Cu2S precipitation. Afterward, the photoelectrode was immersed in 5 mM Na2S anion solution for 60 s to react S2- with adsorbed Cu+ to produce Cu2S. Finally, the photoelectrode was rinsed with deionized water for 60 s. These steps constitute a cycle count. The photoelectrode was immersed in the solution for different cycles (3, 5, 10, 15) to control the deposition amount of Cu2S. Finally, the photoelectrode synthesized by the SILAR method was annealed in an argon atmosphere at 200 °C (5 °C/min) for 2 h, resulting in a sample called Ti/Fe2O3/Cu2S.
Preparation of Ti/Fe2O3/Cu2S/Co(OH)x photoanode
According to previous reports[27], the photoelectrode Ti/Fe2O3/Cu2S/Co(OH)x was synthesized through a simple electrochemical deposition method [Figure 1]. Using a 1 mA/cm2 cathode current density, Ti/Fe2O3/Cu2S photoelectrode was deposited onto ultra-thin Co(OH)x nanosheets in a solution containing 2 mM Co(NO3)2. The best performance of the photoelectrode could be achieved by selecting different deposition times (30, 60, 90, and 120 s). The final sample obtained was called Ti/Fe2O3/Cu2S/Co(OH)x.
Preparation of Cu2S nanoparticles
The saturated CuCl and 5 mM Na2S solutions were mixed with thorough stirring, and the Cu2S nanoparticles were obtained after pumping and filtering. After acid washing and centrifugation, the obtained Cu2S nanoparticles were deposited on fluorine-doped tin oxide (FTO) by spin coating for electrochemical testing.
Materials characterizations
The microscopic morphology was evaluated using a scanning electron microscope (SEM, Apreo S LoVac, Thermo Fisher Scientific) with an operating voltage of 10 kV. The TF20 (FEI), operated at 200 kV, was used to perform transmission electron microscopy (TEM) and selected area electron diffraction (SAED) techniques to characterize the detailed morphology, size, and element composition of the samples. The crystal structure of the sample was described using X-ray diffraction (XRD) data obtained from the Miniflex 600 X-ray diffractometer. The measurement range was 20°-80° with CuK radiation. The light absorption ability of the sample was evaluated by collecting the ultraviolet-visible (UV-vis) absorption spectrum using the Cary 5000 spectrophotometer (Agilent). X-ray photoelectron spectroscopy (XPS) was performed with Al Ka rays.
The bandgap energy (Eg) is determined from UV-vis diffuse reflectance spectroscopy (UV-vis-DRS), specifically utilizing the Tauc diagram. Eg is calculated using:
Which represents Tauc plotting, where a is the direct light absorption coefficient, h is Planck’s constant, v is the frequency of the incident light, A is the proportionality constant, and Eg is the energy gap of the semiconductor photocatalyst. For direct bandgap semiconductors, x equals 2; for indirect bandgap semiconductors, x equals 1/2.
PEC measurements
The PEC test was conducted using a PEC2000 PEC test system (Beijing Perfectlight Technology Co., Ltd., Beijing, China), which comprises a Shanghai Chenhua electrochemical workstation (CHI760e), a xenon lamp (PLS-FX300HU, Beijing Perfectlight) with an AM 1.5G filter (100 mW/cm2), a chopper module (PFS40A shutter actuator), and a single-room electrochemical cell. The PEC tests were conducted under no light, light, or alternating light conditions. Linear sweep voltammetry (LSV) typically measured the potential range between -0.31 and 0.61 V (vs. Ag/AgCl), with light intermittently blocked by a shutter toggling every
Where
The applied bias photon-to-current efficiency (ABPE) with external bias can be calculated using:
Where Jph denotes the photocurrent density (mA/cm2) measured by the electrochemical workstation. Eapp signifies the relationship between the applied bias voltage and ERHE (V), and Plight represents the total light intensity of AM 1.5G (100 mW/cm2).
The incident monochromatic photon electron conversion efficiency (IPCE) and Mott-Schottky (MS) measurements were conducted in the IPCE1000 PEC testing system (Beijing Perfectlight Technology Co., Ltd., Beijing, China). The instrument is equipped with an electrochemical workstation (CS 350H, Wuhan Kotex Instruments Co., Ltd., China). IPCE can be calculated by:
Where Iph (A/cm2) refers to the output photocurrent density at each wavelength (λ, nm), and Pin represents the incident light power density. Usually, the IPCE spectrum is calculated based on the photocurrent density recorded by the chronoamperometric method. The incident monochromatic light of 300-600 nm is separated by a monochromator using a xenon lamp (PLS-FX300HU, Beijing Perfectlight).
The MS measurement results were used to illustrate the flat band potentials. The frequency range of the electrochemical analyzer is 0.1~100,000 Hz. The working electrodes were measured at 500, 1,000, 1,500, and 2,000 Hz, respectively. Based on the MS plot, the flat band potential of the photoelectrode can be determined according to:
Where C is the space charge capacitance, e is the electron charge, ε is the vacuum permittivity
The carrier concentration (ND) of the obtained photoanode can be roughly estimated by:
Electrochemical impedance spectroscopy (EIS) was performed using a Squidstat Plus electrochemical workstation (Admiral Instruments, Tempe, Arizona, USA).
PEC H2 evolution experiments were conducted using an all-glass automatic online trace gas analysis system (Labsolar-6A, Beijing Perfectlight). The system utilized a xenon lamp (Microsolar 300, Beijing Perfectlight). This setup was interfaced with a commercial electrochemical workstation (Squidstat Plus, Admiral Instruments, Arizona) for bias voltage application (0.23 V vs. Ag/AgCl) and photocurrent data collection. The electrolyte used was a 1 M KOH solution (75 mL). Periodic analysis of PEC-generated H2 was performed using a FULI-GC-9790II gas chromatograph (GC) (Zhejiang Fuli) equipped with a thermal conductivity detector (TCD), with argon (Ar) serving as the carrier gas at a flow rate of 30 mL/min.
RESULTS AND DISCUSSION
Morphology and structure
Ti/Fe2O3, Ti/Fe2O3/Cu2S, and Ti/Fe2O3/Cu2S/Co(OH)x photoanodes exhibit a semi-collapsed rod-shaped structure [Figure 2A-C], belonging to one-dimensional nanomaterials. The side view shows that the length of these rods is approximately 700 nm. Compared to Ti/Fe2O3, there is no obvious Cu2S and Co(OH)x on the surface of Ti/Fe2O3/Cu2S and Ti/Fe2O3/Cu2S/Co(OH)x photoanodes, which may be due to the relatively small loading amount of Cu2S and the deposition amount of Co(OH)x. By increasing the loading of Cu2S, it was demonstrated that Cu2S nanoparticles were synthesized, as detailed in Supplementary Figure 1. The rod-like structure of the Ti/Fe2O3/Cu2S/Co(OH)x photoanode is observed by TEM [Figure 2D]; in Figure 2E, the calculated lattice spacing is compared with the XRD standard spectrum, demonstrating that the (102) crystal plane of Cu2S is on the left of the figure[30], while the (202) crystal plane of Fe2O3 is on the right. In the high-resolution mode[31], a phase interface between Fe2O3 and Cu2S is observed, with a heterojunction structure forming between the two. In addition, an ultra-thin Co(OH)x film outside the Fe2O3 and Cu2S heterojunction structure wraps both, forming a Co(OH)x-covered p-Cu2S/n-Fe2O3 structure.
Figure 2. (A-C) SEM images of Ti/Fe2O3, Ti/Fe2O3/Cu2S, and Ti/Fe2O3/Cu2S/Co(OH)x photoanodes; (D and E) HRTEM images of Ti/Fe2O3/Cu2S/Co(OH)x photoanode; (F) SAED images of Ti/Fe2O3/Cu2S/Co(OH)x photoanode; (G) STEM image of Ti/Fe2O3/
The SAED characterization results are shown in Figure 2F. The SAED profiles correspond to the (001) and (002) crystal faces in Cu2S and (010) crystal face in Co(OH)x, which are the main peaks of Cu2S and
Figure 3A shows the XRD results of three types of photoanodes. By comparing them with standard cards (PDF#44-1294) and (PDF#33-0664), it is proven that they are the characteristic XRD peaks of metal Ti and Fe2O3. The XRD results showed that the structure of Fe2O3 remained unchanged during the preparation and electrochemical deposition of SILAR. At the same time, no XRD peaks of Cu2S and Co(OH)x were observed, indicating a relatively low content of Cu2S and Co(OH)x. Additionally, the XRD fine maps of these three photoanodes from 32.5° to 34.0° [Figure 3B] reflected that the introduction of a small amount of Cu2S and Co(OH)x did not shift the X-ray characteristic diffraction peaks of Fe2O3 and metal Ti, which indicated that Cu2S and Co(OH)x did not alter the physical phases of Fe2O3 and metal Ti. They were only loaded or encapsulated on the Fe2O3 and metal Ti.
Surface analysis and elemental composition
XPS was performed on Ti/Fe2O3, Ti/Fe2O3/Cu2S, and Ti/Fe2O3/Cu2S/Co(OH)x photoanodes to confirm the formation of heterostructures and analyze the chemical states of elements. The XPS full spectrum [Figure 4A] reveals that the Ti/Fe2O3/Cu2S/Co(OH)x photoanode comprises Fe, O, Cu, S, and Co. Similarly, the other two photoanodes also exhibit the presence of these elements. In the Fe 2p region [Figure 4B], the XPS spectra reveal two peaks at approximately 724 and 711 eV, characteristic of Fe3+, along with two satellite peaks observed at 732.74 and 718.35 eV. It can be seen from the figure that the two main peaks at about 724 and 711 eV confirm the presence of Fe3+ as the main component on these three photoanodes; these main peaks are also accompanied by satellite peaks at about 733 and 718 eV, also indicative of Fe3+ presence; the XPS peaks of these three photoanodes show no shifts[32,33]. As can be seen from Figure 4C, the peak of the
Figure 4. XPS spectra of Ti/Fe2O3, Ti/Fe2O3/Cu2S, and Ti/Fe2O3/Cu2S/Co(OH)x photoanodes. (A) the survey spectra; (B) Fe 2p; (C) O 1s; (D) Cu 2p; (E) S 2p; and (F) Co 2p. XPS: X-ray photoelectron spectroscopy.
As shown in Figure 5A, the trend of the curves of the UV-vis absorption spectra of these three photoanodes is consistent, and the test results illustrate that the absorption of these three samples is relatively strong in the UV region and in the visible region from 420 to 600 nm. The bandgap can be estimated from the Tauc plot [Equation (1)][42], so that the bandgap of Fe2O3, the main semiconductor material of the three photoanodes, can be deduced from the test results of the UV-Vis diffuse reflectance spectra to be 1.98 eV [Figure 5B].
PEC and EC analysis
PEC measurement
A series of PEC tests[43] were used to characterize the iron oxide nanorod photoanode[44]. The LSV results
Figure 6. PEC performance of Ti/Fe2O3, Ti/Fe2O3/Cu2S, and Ti/Fe2O3/Cu2S/Co(OH)x photoanodes. (A) Chopped LSV curves under AM 1.5G (100 mW/cm2); (B) Chopped LSV curves under visible light (λ > 420 nm, 66.7 mW/cm2); (C) ABPE curves under AM 1.5G
Supplementary Table 1 shows the PEC performance of the Ti/Fe2O3/Cu2S/Co(OH)x photoanodes in this study compared to other recently reported Fe2O3-based photoanodes. The final comparison results show the excellent performance of our prepared photoanode.
The ABPE [Figure 6C] of each photoanode below the water oxidation thermodynamic potential (1.23 V vs. RHE) was calculated based on the LSV results[45]. Figure 6C illustrates that the ABPE efficiency of the Ti/
I-t and measurement of light-assisted H2 production
To verify the stability of the prepared photoanode, chronoamperometry (I-t) was conducted [Figure 6E]. Under continuous illumination for 1 h, the Ti/Fe2O3/Cu2S/Co(OH)x (I-t, 1.23 V vs. RHE) photoanode showed good stability, and the photocurrent remained almost unchanged. To further demonstrate the stability of the synthesized samples, a 19-hour stability test [Supplementary Figure 4] was performed on top of the original 1-hour test [Figure 6E]. The results indicated that the samples exhibited enhanced stability. Following the stability tests, the samples were subjected to a series of characterizations [Supplementary Figures 5-11].
The actual H2 generation performance of various samples at 1.23 V (vs. RHE) was recorded to investigate the relevance of the observation of photocurrent to the solar-assisted hydrolysis H2 generation reaction. Photoanodes with high photocurrent density often exhibit higher hydrogen production rates under optoelectronic conditions. The 3-hour measurement period [Figure 6F] showed a linear increase in the amount of H2 generation over time, indicating a stable H2 generation rate for the sample and reaffirming the stability of the Ti/Fe2O3/Cu2S/Co(OH)x photoanode.
IPCE measurement
In order to explore the response of the photoanode to different wavelengths of light, the prepared photoanode was used to conduct the IPCE test. During the test, the light source irradiates from 300 nm, changes to 600 nm at a constant speed, and ends at 600 nm; the wavelength resolution of the spectral analysis is 1 nm. It can be seen from Figure 7A that under the electrochemical noise mode, the pristine Ti/Fe2O3 photoanode has photocurrent performance in the wavelength region of 300-600 nm, which indicates that Fe2O3 material has a wide light absorption range and an excellent visible light response. Compared with Ti/Fe2O3, the photocurrent densities and IPCE values of Ti/Fe2O3/Cu2S, Ti/Fe2O3/Co(OH)x, and Ti/Fe2O3/Cu2S/Co(OH)x photoanodes gradually increase, which shows that the introduction of Cu2S and Co(OH)x improves the photoelectric performance of Fe2O3; this improvement covers the entire wavelength range from 300 to 600 nm. Under the constant potential polarization mode with different applied bias voltages [Figure 7B], the photocurrent density and IPCE value of these photoanodes gradually increase with higher bias voltages, which shows that increasing the applied bias voltage is helpful for photogenerated current carrying. The separation of electrons strengthens the photoelectric properties of the sample. Under the conditions of the electrochemical noise test [Figure 7C] and constant potential test [Figure 7D], the IPCE peaks of Ti/Fe2O3, Ti/Fe2O3/Cu2S, Ti/Fe2O3/Co(OH)x, and Ti/Fe2O3/Cu2S/Co(OH)x photoanodes are all at 357 nm place. Under electrochemical noise test conditions, at 1.23 (vs. RHE) and
MS analysis
To investigate the energy band structure information of the Ti/Fe2O3/Cu2S/Co(OH)x photoanode, key parameters such as the conduction band (CB), flat band potential, and carrier concentration were determined. The effects of the introduction of Cu2S and Co(OH)x on the Ti/Fe2O3 photoanode were also examined. Therefore, Ti/Fe2O3, Ti/Fe2O3/Cu2S, Ti/Fe2O3/Co(OH)x and Ti/Fe2O3/Cu2S/Co(OH)x photoanodes were all subjected to MS tests.
The longest segment of the MS curve makes an extension line to get the intersection with the baseline for the flat-charged potential position. The intersection of the extension lines for the different frequencies of the four photoanodes is a single point[46]. The positive slopes of the tangent lines for all four samples indicate their n-type nature[20]. In both n- and p-type semiconductors, the flat band potential can be approximated as the CB potential. The results shown in Figure 8A-D indicate that the introduction of both Cu2S and Co(OH)x results in a more negative flat-band potential for the Ti/Fe2O3 photoanode. Compared to the Ti/Fe2O3 photoanode, the CB potential of the Ti/Fe2O3/Co(OH)x, Ti/Fe2O3/Cu2S, and Ti/Fe2O3/Cu2S/Co(OH)x photoanodes were reduced from 0.14 (vs. RHE for Ti/Fe2O3) to 0.10, -0.05, and -0.06 V (vs. RHE), respectively. A negative movement of the flat band potential suggests a reduced energy potential barrier for electron transfer at the interface, contributing to a reduction in the charge transfer resistance[44]. Therefore, compared with the other three, the Ti/Fe2O3/Cu2S/Co(OH)x photoanode has the most negative CB potential and the best photocurrent and photocoupling hydrogen production performance, corresponding to the previous results.
Figure 8. Mott-Schottky plots have frequencies from 500 to 2,000 Hz. (A) Ti/Fe2O3 photoanode; (B) Ti/Fe2O3/Cu2S photoanode; (C) Ti/Fe2O3/Co(OH)x photoanode; (D) Ti/Fe2O3/Cu2S/Co(OH)x photoanode; (E) Frequency (500 to 1,500 Hz) of the Mott-Schottky diagram of Cu2S nanoparticles; (F) The carrier concentrations of Ti/Fe2O3 photoanode (TF), Ti/Fe2O3/Cu2S photoanode (TFCu), Ti/Fe2O3/Co(OH)x photoanode (TFCo), and Ti/Fe2O3/Cu2S/Co(OH)x photoanode (TFCuCo).
Separately synthesized Cu2S powders were also tested for MS; the results are shown in Figure 8E. The intersection of the extension lines of the data curves for the three different frequencies is a point whose tangent line has a negative slope, which reveals that the Cu2S semiconductor has p-type properties[47] with a flat-band potential (approximated as the conduction-band potential) of 0.14 V (vs. RHE). The carrier concentrations of these four photoanodes were calculated from the MS test data [Figure 8F]. The results show that the carrier concentration of the Ti/Fe2O3/Cu2S/Co(OH)x photoanode is one magnitude higher compared with that of the Ti/Fe2O3, Ti/Fe2O3/Cu2S, and Ti/Fe2O3/Co(OH)x, which is one of the reasons for its good performance.
EIS analysis
Frequency transmission characteristics and electrochemical properties of photoanodes were studied using EIS[48]. Nyquist plots were obtained for the samples under dark conditions at open-circuit potential [Figure 9A] and at 1.23 V (vs. RHE) [Figure 9B]. Measurements were conducted in the frequency range of 100 MHz to 100 kHz. The equivalent circuit [Figure 9] includes a resistor (R), a capacitor (C), a constant phase element (CPE), and a Warburg impedance element (W). From the small figure in Figure 9A, it can be seen (under open-circuit potential conditions) that the radii of the arcs of the EIS curves are smaller than those of Ti/Fe2O3/Cu2S and Ti/Fe2O3/Cu2S/Co(OH)x in the high-frequency region (0-5 KOhms); moreover, from the internal small figure in Figure 9B, it can be seen that the radii of the arcs of the EIS curves are smaller than those of Ti/Fe2O3 and Ti/Fe2O3/Cu2S/Co(OH)x in the high-frequency region (0-5 KOhms). Specifically, the arc radius of the EIS curve for Ti/Fe2O3/Cu2S/Co(OH)x is smaller than that of both Ti/Fe2O3 and Ti/
Mechanistic analysis and bandgap calculation
The Eg of the photoanode samples are calculated using Tauc plots, which depict [(IPCE% × hv)1/2 against photon energy (hv)] in the electrochemical noise mode[44] [Figure 10A]. The main component of all four photoanodes is α-Fe2O3, so they all reflect the Eg of α-Fe2O3 of 2.10 eV, which agrees with the previously reported results[50]. The Eg can be evaluated from the Tauc plot [Equation (1)]. The Eg [Figure 10B] of the Cu2S semiconductor nanoparticles alone was calculated by UV-vis spectroscopy test as 1.19 eV.
Figure 10. (A) The bandgap energy (Eg) Ti/Fe2O3 photoanode, Ti/Fe2O3/Cu2S photoanode, Ti/Fe2O3/ Co(OH)x photoanode and Ti/
Figure 11A and B shows the Eg structure map based on the IPCE electrochemical noise pattern, UV-vis spectroscopy, and MS measurements. The valence band (VB) of the photocatalytic sample is determined from the estimated CB and Eg. Results indicate that the VB and CB of n-Fe2O3 are 2.24 and 0.14 V, respectively, while those of p-Cu2S are 0.41 and 0.14 V, respectively.
Figure 11. (A) Photoanode Ti/Fe2O3/Cu2S/Co(OH)x photoelectric hydrogen production mechanism diagram; (B) Photoanode Ti/
By the observations of the above experiments, we present the charge separation mechanism of ultra-thin Co(OH)x package p-Cu2S/n-Fe2O3 to explain its strengthened PEC properties [Figure 9][19,51]. Before contact, the CB and VB energy levels of p-type Cu2S are higher than those of n-type Fe2O3, whereas the Fermi energy level (EF) of Cu2S is lower than that of Fe2O3. After forming the p-n junction heterostructure, the energy level of Cu2S rises while that of Fe2O3 decreases, leading to an equilibrium of their EFs. Consequently, an internal electric field is formed at the Fe2O3/Cu2S interface under thermodynamic equilibrium conditions. Due to the energy matching between Fe2O3 and Cu2S, their energy bands shift together until the Fermi levels align, resulting in band bending in the space charge region. Electrons generated and separated in the CB of Cu2S move to the CB of Fe2O3, while holes separated and retained in the VB of Fe2O3 flow to the VB of Cu2S to react with the electrolyte. Therefore, the p-Cu2S/n-Fe2O3 heterojunction photoanode enhances photogenerated electron-hole pair separation and improves light harvesting efficiency. After modification with the Co(OH)x co-catalyst on Fe2O3/Cu2S, photogenerated electrons are efficiently transferred from
CONCLUSIONS
In conclusion, we synthesized an ultrathin Co(OH)x-encapsulated p-Cu2S/n-Fe2O3, which enhances charge separation and surface oxygen evolution kinetics of the iron oxide nanorod array photoanode through the synergistic effect of p-n heterojunctions and OECs. In 1 M KOH solution [25 °C, AM 1.5G, 1.23 V (vs. RHE)], the photocurrent density of Ti/Fe2O3/Cu2S/Co(OH)x is about three times that of Ti/Fe2O3, reaching 4.8 mA/cm2. Under visible light irradiation [λ > 420 nm, AM 1.5G, 1.23 V (vs. RHE)], its photocurrent density also reaches 2.4 mA/cm2, which is half of the full spectrum light; in addition, the corresponding optical response limit is extended to 600 nm. Without sacrificial agents and photosensitizers, we observed a significant increase in the hydrogen evolution rate of Ti/Fe2O3/Cu2S/Co(OH)x, reaching 76.4 μmol/cm2. Our work provides a novel concept for photoanode PEC water splitting.
DECLARATIONS
Authors’ contributions
Prepared and revised the manuscript: Shi HY, Ji MH, Zhou QQ, Li KX, Wang HL, Chen R
Designed and revised the manuscript: Chen YX, Lin XM, Lu CZ
All authors contributed to the discussion and preparation of the manuscript.
Availability of data and materials
The authors confirm that the data supporting the findings of this study are available within its Supplementary Materials.
Financial support and sponsorship
Authors are thankful for the financial support of the Natural Science Foundation of Fujian Province (2023H0046), the XMIREM autonomously deployment project (2023CX10, 2023GG01), the National Natural Science Foundation of China (22275185), the Major Research Project of Xiamen (3502Z20191015), and the Fujian Science & Technology Innovation Laboratory for Optoelectronic Information of China (2021ZR132, 2021ZZ115).
Conflicts of interest
Chen YX is the Junior Editorial Board Member of Chemical Synthesis, while the other authors have declared that they have no conflicts of interest.
Ethical approval and consent to participate
Not applicable.
Consent for publication
Not applicable.
Copyright
© The Author(s) 2024.
Supplementary Materials
REFERENCES
2. Wang Z, Gu Y, Zheng L, et al. Machine learning guided dopant selection for metal oxide-based photoelectrochemical water splitting: the case study of Fe2O3 and CuO. Adv Mater 2022;34:e2106776.
3. Liu B, Wang S, Zhang G, et al. Tandem cells for unbiased photoelectrochemical water splitting. Chem Soc Rev 2023;52:4644-71.
4. Li Y, Zhang D, Qiao W, et al. Nanostructured heterogeneous photocatalyst materials for green synthesis of valuable chemicals. Chem Synth 2022;2:9.
5. Fujishima A, Honda K. Electrochemical photolysis of water at a semiconductor electrode. Nature 1972;238:37-8.
6. Nayak AK, Sohn Y, Pradhan D. Facile green synthesis of WO3·H2O nanoplates and WO3 nanowires with enhanced photoelectrochemical performance. Cryst Growth Des 2017;17:4949-57.
7. Zhao HP, Zhu ML, Shi HY, et al. Cerium-doped iron oxide nanorod arrays for photoelectrochemical water splitting. Molecules 2022;27:9050.
8. Li C, Luo Z, Wang T, Gong J. Surface, bulk, and interface: rational design of hematite architecture toward efficient photo-electrochemical water splitting. Adv Mater 2018;30:e1707502.
9. Tang P, Arbiol J. Engineering surface states of hematite based photoanodes for boosting photoelectrochemical water splitting. Nanoscale Horiz 2019;4:1256-76.
10. Gao L, Chai H, Niu H, Jin J, Ma J. Roles of cobalt-coordinated polymeric perylene diimide in hematite photoanodes for improved water oxidation. Small 2023;19:e2302665.
11. Wu J, Cheng Y, Lin J, Huang Y, Huang M, Hao S. Fabrication and photocatalytic properties of HLaNb2O7/(Pt, Fe2O3) pillared nanomaterial. J Phys Chem C 2007;111:3624-8.
12. Wang H, Hu Y, Song G, Zheng D. Intrinsic and extrinsic doping to construct hematite nanorod p-n homojunctions for highly efficient PEC water splitting. Chem Eng J 2022;435:135016.
13. Fujimoto H, Nakayasu B, Tobisu M. Synthesis of γ-lactams from acrylamides by single-carbon atom doping annulation. J Am Chem Soc 2023;145:19518-22.
14. Li F, Yue X, Liao Y, Qiao L, Lv K, Xiang Q. Understanding the unique S-scheme charge migration in triazine/heptazine crystalline carbon nitride homojunction. Nat Commun 2023;14:3901.
15. Zhang M, Mao Y, Bao X, et al. Coupling benzylamine oxidation with CO2 photoconversion to ethanol over a black phosphorus and bismuth tungstate S-scheme heterojunction. Angew Chem Int Ed Engl 2023;62:e202302919.
16. Shen H, Qu F, Xia Y, Jiang X. Straightforward and ultrastable surface modification of microfluidic chips with norepinephrine bitartrate improves performance in immunoassays. Anal Chem 2018;90:3697-702.
17. Li Z, Zhang Z. Tetrafunctional Cu2S thin layers on Cu2O nanowires for efficient photoelectrochemical water splitting. Nano Res 2018;11:1530-40.
18. Guo K, Liu Z, Zhou C, et al. Fabrication of TiO2 nano-branched arrays/Cu2S composite structure and its photoelectric performance. Appl Catal B Environ 2014;154-5:27-35.
19. He B, Wang Y, Liu X, et al. Spatial engineering of a Co(OH)x encapsulated p-Cu2S/n-BiVO4 photoanode: simultaneously promoting charge separation and surface reaction kinetics in solar water splitting. J Mater Chem A 2019;7:6747-52.
20. Zhang Y, Huang Y, Zhu SS, et al. Covalent S–O bonding enables enhanced photoelectrochemical performance of Cu2S/Fe2O3 heterojunction for water splitting. Small 2021;17:e2100320.
21. Wu Y, Yao S, Lv G, et al. Construction of p-n junctions in single-unit-cell ZnIn2S4 nanosheet arrays toward promoted photoelectrochemical performance. J Catal 2021;401:262-70.
22. Li Z, Li J, Wang W, et al. Near zero-threshold voltage P-N junction diodes based on super-semiconducting nanostructured Ag/Al arrays. Adv Mater 2023;35:e2210612.
23. Zhao Y, Westerik P, Santbergen R, Zoethout E, Gardeniers H, Bieberle-hütter A. From geometry to activity: a quantitative analysis of WO3/Si micropillar arrays for photoelectrochemical water splitting. Adv Funct Mater 2020;30:1909157.
24. Meng L, Zhou X, Wang S, et al. A plasma-triggered O-S bond and P-N junction near the surface of a SnS2 nanosheet array to enable efficient solar water oxidation. Angew Chem Int Ed Engl 2019;58:16668-75.
25. Wu Y, Liu X, Zhang H, et al. Atomic sandwiched p-n homojunctions. Angew Chem Int Ed Engl 2021;60:3487-92.
26. Mcdonald KJ, Choi KS. Photodeposition of Co-based oxygen evolution catalysts on α-Fe2O3 photoanodes. Chem Mater 2011;23:1686-93.
27. Yang G, Li Y, Pang H, Chang K, Ye J. Ultrathin cobalt–manganese nanosheets: an efficient platform for enhanced photoelectrochemical water oxidation with electron-donating effect. Adv Funct Mater 2019;29:1904622.
28. Yang Z, Jiang Y, Zhang W, et al. Solid-state, low-cost, and green synthesis and robust photochemical hydrogen evolution performance of ternary TiO2/MgTiO3/C photocatalysts. iScience 2019;14:15-26.
29. An Y, Lin C, Dong C, et al. Scalable photoelectrochemical cell for overall solar water splitting into H2 and H2O2. ACS Energy Lett 2024;9:1415-22.
30. Cao Q, Che R, Chen N. Scalable synthesis of Cu2S double-superlattice nanoparticle systems with enhanced UV/visible-light-driven photocatalytic activity. Appl Catal B Environ 2015;162:187-95.
31. Li J, Li J, Yuan H, Zhang W, Jiao Z, Song Zhao X. Modification of BiVO4 with partially covered α-Fe2O3 spindles serving as hole-transport channels for significantly improved photoelectrochemical performance. Chem Eng J 2020;398:125662.
32. Yi S, Wulan B, Yan J, Jiang Q. Highly efficient photoelectrochemical water splitting: surface modification of cobalt-phosphate-loaded Co3O4/Fe2O3 p–n heterojunction nanorod arrays. Adv Funct Mater 2019;29:1801902.
33. Gota S, Guiot E, Henriot M, Gautier-soyer M. Atomic-oxygen-assisted MBE growth of α-Fe2O3 on α-Al2O3 (0001): metastable FeO(111)-like phase at subnanometer thicknesses. Phys Rev B 1999;60:14387-95.
34. Liu P, Hensen EJ. Highly efficient and robust Au/MgCuCr2O4 catalyst for gas-phase oxidation of ethanol to acetaldehyde. J Am Chem Soc 2013;135:14032-5.
35. Wang G, Ling Y, Wheeler DA, et al. Facile synthesis of highly photoactive α-Fe2O3-based films for water oxidation. Nano Lett 2011;11:3503-9.
36. Abraham KM, Chaudhri SM. The lithium surface film in the Li/SO2 cell. J Electrochem Soc 1986;133:1307-11.
37. Benoist L, Gonbeau D, Pfister-Guillouzo GP, Schmidt E, Meunier G, Levasseur A. XPS analysis of oxido-reduction mechanisms during lithium intercalation in amorphous molybdenum oxysulfide thin films. Solid State Ionics 1995;76:81-9.
38. Liang Y, Wang H, Zhou J, et al. Covalent hybrid of spinel manganese-cobalt oxide and graphene as advanced oxygen reduction electrocatalysts. J Am Chem Soc 2012;134:3517-23.
39. Xu Y, Wang X, Chen H, Kuang D, Su C. Toward high performance photoelectrochemical water oxidation: combined effects of ultrafine cobalt iron oxide nanoparticle. Adv Funct Mater 2016;26:4414-21.
40. Nie R, Shi J, Du W, Ning W, Hou Z, Xiao F. A sandwich N-doped graphene/Co3O4 hybrid: an efficient catalyst for selective oxidation of olefins and alcohols. J Mater Chem A 2013;1:9037-45.
41. Kim H, Park J, Park I, et al. Coordination tuning of cobalt phosphates towards efficient water oxidation catalyst. Nat Commun 2015;6:8253.
42. He B, Jia S, Zhao M, et al. General and robust photothermal-heating-enabled high-efficiency photoelectrochemical water splitting. Adv Mater 2021;33:e2004406.
43. Zhang Y, Liu Y, Gong X, et al. Construction of piezoelectric photocatalyst Au/BiVO4 for efficient degradation of tetracycline and studied at single-particle level. Chem Synth 2024;4:21.
44. Tong M, Wang T, Lin S, et al. Ultra-thin carbon doped TiO2 nanotube arrays for enhanced visible-light photoelectrochemical water splitting. Appl Surf Sci 2023;623:156980.
45. Tong MH, Chen YX, Wang TM, et al. Cerium synchronous doping in anatase for enhanced photocatalytic hydrogen production from ethanol-water mixtures. Molecules 2023;28:2433.
46. Jiang X, Zhou J, Liu H, Chen Y, Lu C. Lotus pollen-templated synthesis of C, N, P-self doped KTi2(PO4)3/TiO2 for sodium ion battery. Colloid Surface A 2022;650:129605.
47. Zhou Q, Chen Y, Shi H, et al. The construction of p/n-Cu2O heterojunction catalysts for efficient CO2 photoelectric reduction. Catalysts 2023;13:857.
48. Tong M, Chen Y, Lin S, et al. Synchronous electrochemical anodization: a novel strategy for preparing cerium doped TiO2 nanotube arrays toward visible-light PEC water splitting. Electrochim Acta 2023;463:142793.
49. Zhang Y, Xu L, Liu B, et al. Engineering BiVO4 and oxygen evolution cocatalyst interfaces with rapid hole extraction for photoelectrochemical water splitting. ACS Catal 2023;13:5938-48.
50. Xiao J, Fan L, Huang Z, et al. Functional principle of the synergistic effect of co-loaded Co-Pi and FeOOH on Fe2O3 photoanodes for photoelectrochemical water oxidation. Chinese J Catal 2020;41:1761-71.
Cite This Article

How to Cite
Download Citation
Export Citation File:
Type of Import
Tips on Downloading Citation
Citation Manager File Format
Type of Import
Direct Import: When the Direct Import option is selected (the default state), a dialogue box will give you the option to Save or Open the downloaded citation data. Choosing Open will either launch your citation manager or give you a choice of applications with which to use the metadata. The Save option saves the file locally for later use.
Indirect Import: When the Indirect Import option is selected, the metadata is displayed and may be copied and pasted as needed.
About This Article
Copyright
Author Biographies
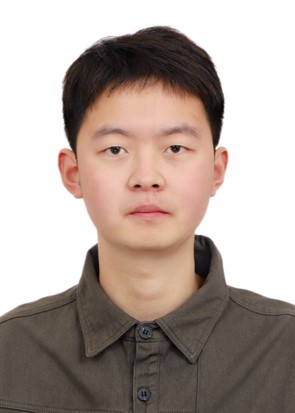
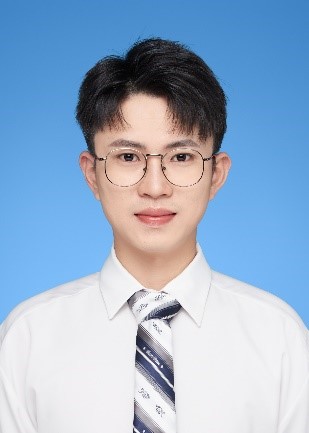
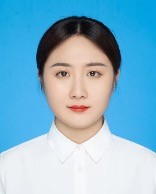
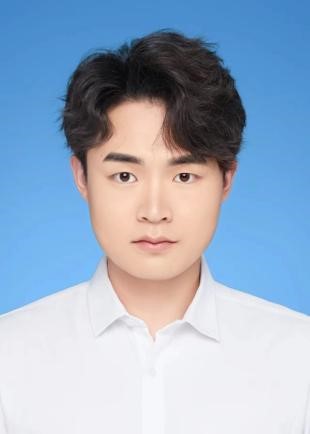
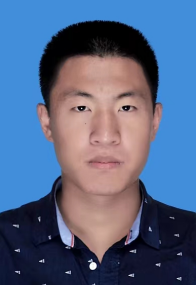
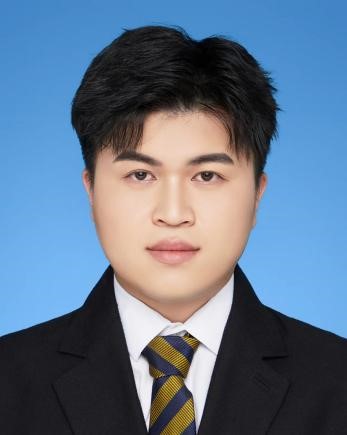
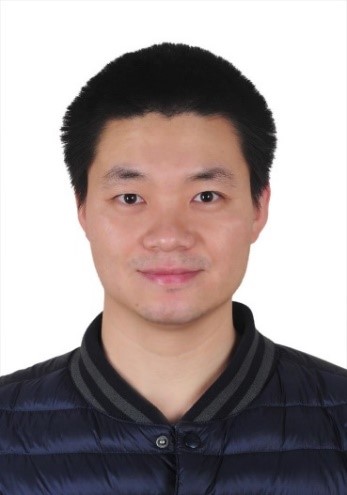
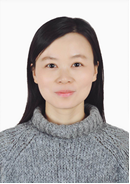
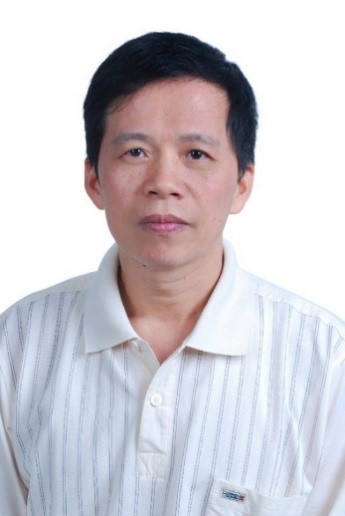
Comments
Comments must be written in English. Spam, offensive content, impersonation, and private information will not be permitted. If any comment is reported and identified as inappropriate content by OAE staff, the comment will be removed without notice. If you have any queries or need any help, please contact us at [email protected].