Shining light on electrochemistry: a synchrotron-based X-ray spectroscopic interrogation
Abstract
Over the last few decades, synchrotron radiation has experienced a flourishing growth, fueled by cutting-edge spectroscopic techniques that have empowered its remarkable ability to probe down to the atomic level. Indeed, this advancement has been inspiring, unlocking powerful insights and capabilities in the realm of electrochemistry community. This perspective showcases recent ground-breaking efforts and remaining challenges with respect to X-ray spectroscopy, as well as their implications for ongoing research.
Keywords
Synchrotron radiation, electromagnetic emission from relativistic charged particles traveling along a circular trajectory, was first discovered in the late 1940s[1]. Once known as a notorious by-product in high-energy physics, synchrotron radiation has come a long way and is now on the cusp of a new era - an unprecedented brilliant, tunable and short pulsed light source[2,3]. Over the enduring decades, synchrotrons have revolutionized our ability to probe and understand the matter with the motto “making visible the invisible”[4,5]. This perspective aims to provide an overview of synchrotron-based X-ray spectroscopic interrogation in the realm of electrochemistry, which has inspired accumulative research outputs in this community spanning classical electrochemistry, electrocatalytic and spectroscopic aspects. An additional note is that we focus exclusively on the synchrotron-based studies here, and we refer the reader elsewhere for other independent excellent contributions.
CLASSICAL ELECTROCHEMISTRY: ELECTRICAL DOUBLE LAYER
In electrochemistry, a typical scenario involves an electrified surface in contact with an electrolyte solution [Figure 1A]. The electrical double layer (EDL), also known as the Stern layer and the diffuse layer, is a micro-region of a few nanometers in thickness and is spatially concealed between the two bulk phases of solid and liquid[6]. For the imbedded interface, there is increasing consciousness that its molecular-level characteristics, to a large extent, underpin the critical functions and properties relevant in many electrochemical processes, while these characteristics remain hitherto elusive. Electron-yield X-ray absorption (XAS) and X-ray photoemission spectroscopy (XPS) offer a promising solution to this old yet current enigma, benefiting from their inherent surface sensitivity. By virtue of instrumental innovation, Velasco-Velez et al. pioneered the extension of soft XAS (sXAS) to operando electrochemical measurements in 2014[7]. They applied a modulated (piezo-actuated chopped) incoming X-ray and lock-in amplifier to extract the neat total electron yield (TEY) signal under electrical bias and experimentally identified the evolved water orientation once the electrode potential deviates from the potential of zero (free) charge (pzfc) [Figure 1B and C]. This finding serves as a paradigm for the argument on the interfacial electrical field-driven water reorganization behavior, which accounts for large kinetic pH effect in hydrogen evolution reaction[8]. In alkaline media, the significant cathodic shift of electrode bias with respect to the pzfc leads to a stronger interfacial electric field and, therefore, an increased water reorganization barrier to accommodate charge transfer. The initial XPS studies were conducted using immersed electrodes under static conditions, with a particular focus on the specific adsorption of ions at the inner Helmholtz plane[9,10]. The investigation of bias-dependent probing of EDL was not accessible until the introduction of the “dip and pull” method
Figure 1. Imbedded interface-the electrical double layer. (A) schematic of the classical Gouy-Chapman-Stern model. The IHP, OHP and diffuse layer are indicated. The potential φ is shown with respect to the distance from the surface. The yellow and purple denote the cations and anions, respectively; (B) schematic of the modulation setup consisting of piezo-actuated chopped incoming X-ray and
MODERN ELECTROCATALYSIS
Recent decades have witnessed a remarkable flourishing of electrocatalysis that extends beyond classical electrochemistry. X-ray absorption fine structure (XAFS) spectroscopy, with its unique combination of both element specificity and local structure sensitivity[19], experiences highly productive advancements driven by the growing demands of nanocatalysts for electrochemical applications. It provides comprehensive insights into electronic state and local structure of reactive centers, including quantitative information at the atomic level with respect to the photo-absorbing atom, i.e., near-neighbor species (the accuracy of determining the atomic number Z, ±5 or so) and interatomic distance, symmetry and coordination number [Figure 2A]. Particularly, the spectral characteristics bring new opportunities to build on progressive guidelines for predictive activity and rational design of target catalysts, in striking contrast to the sophisticated theoretical ones. For instance, Huang et al. developed experimental Sabatier plot for Pt-alloy oxygen reduction reaction (ORR) catalysts based on tangible descriptors of strain and asymmetry factor[20]. Besides, the XAFS technique is completely compatible with in-situ/operando studies. Measurements under realistic working conditions empower us to monitor the potential-driven valence oscillation[21] and dynamic active sites (phase transformation[22], metal-ligand distortion/displacement[23], etc.), propelling the fundamental understanding to new heights. We refer the reader to excellent reviews on the utilization of various in-situ electrolyte cells[24], which represent an important technical aspect of the applicability of X-ray techniques and accurate data acquisition under working conditions. Achieving satisfactory signal-to-noise ratios in XAFS spectra often necessitates the use of high catalyst loadings, which, in turn, introduce significant limitations in terms of ion and mass transport[25]. In light of this, the author suggests an appropriate catalyst layer thickness while also adopting the common practice of multiple scans to improve data acquisition. Moreover, the author would like to reiterate that the behavior of reactive species on surfaces can hardly be captured by conventional XAFS measurements owing to their extremely short lifespan within the timescale. This argument is solidly supported by recent publications on prototypical single-atomic Co-N-C electrocatalysts[26,27]. The comparative in-situ Co K-edge X-ray absorption near-edge structure (XANES) spectra of CoNC (in-plane embedded Co sites) and CoNOC (edge-hosted cobalt sites) unequivocally reveal that the spectral change during ORR operation is primarily dictated by potential-driven structure evolution, whereas the adsorption of oxygenated intermediates exerts only a negligible effect [Figure 2B]. As far as we are concerned, the issue on a holistic view of the reaction process can be best addressed by the correlative infrared/Raman spectroscopy[28,29], which offers complementary information to XAFS study.
Figure 2. Hard XAS/XES for electrocatalysts characterization. (A and C) representative energy level diagrams depict the origin of the spectral features. The XAS involves the excitation of core-level electron to the unoccupied states or into the continuum, serving as a probe of the unoccupied orbitals of chemical species and local atomic structure. In contrast, the XES monitors the decay of core holes (fluorescent X-rays associated with electron from higher-lying orbitals decay to fill the core hole) and, therefore, probes the occupied valence states in an atom-specific projection; (B) in-situ Co K-edge XANES study on CoNC and CoNOC during oxygen reduction reaction operation. Comparative study reveals the stable and rigid in-plane embedded Co1Nx moiety and the flexible edge-hosted Co1N2 sites. The observed changes in the XANES spectra are primarily dictated by potential-driven structure evolution, whereas the adsorption of oxygenated intermediates exerts only a negligible effect. These figures are quoted with permission from Hu et al[27]; (D) comparison of in-situ Kβ mainline XES spectra recorded on DW21 catalyst in N2-saturated 0.5 M H2SO4 at OCV and 0.2 V vs. RHE, along with corresponding fit results. The inset shows a magnified view of the Kβ’ region; (E) the time course of applied bias in the in situ XES measurements, whereby each potential hold lasted 10 min; (F) Average spin states at each corresponding potential. These figures in
Commissioning third/fourth-generation synchrotrons demonstrate unique opportunities to advanced spatial and energy-resolved spectroscopic techniques. Heterogeneous electrocatalysts are inherently non-uniform, where the nanoscale variations in atomic structure, composition, and accessibility do impact their reactivity. We highlight recent insights into site-specific reactivity in electrocatalysis by conducting high spatial-resolved studies instead of ensemble-averaged measurements. Remarkably, Mefford et al. resort to operando scanning transmission X-ray microscopy (STXM) to disentangle the sequential dehydrogenation process from β-Co(OH)2 to β-CoOOH through the single platelet particle during the oxygen evolution reaction operation[30]. To date, the compositional encyclopedia of electrocatalysts mostly encompasses the transition metal-based systems. Direct probing of metal d-states is of immense significance and has long been plagued by either forbidden dipole electronic transition or core-hole lifetime broadening of conventional XANES. In contrast, X-ray emission spectroscopy (XES) emerges as a promising alternative to provide complementary information with respect to the electron structure (local charge- and spin-density) and local environment of the emitting species via monitoring the intensity of a fluorescence line associated with a specified excited state decay process using a narrow energy resolution [Figure 2C]. A notable application of this method is demonstrated by Saveleva et al. through operando Kβ1,3 XES, where they provide compelling evidence of the decrease in the average spin state of iron atoms during ORR
OUTLOOK
Modern electrochemical cells are increasingly dependent on the membrane electrode assembly-based electrodes, which no longer stick to the ubiquitous solid-liquid interface in the past. Instead, there is a growing demand for deep insight into the new-emerging solid/polymer/gas interface. Synchrotron-based
DECLARATIONS
Authors’ contributions
The author contributed solely to the article.
Availability of data and materials
Not applicable.
Financial support and sponsorship
This work was supported by the National Natural Science Foundation of China (No. 22002013).
Conflicts of interest
The author declared that there is no conflict of interest.
Ethical approval and consent to participate
Not applicable.
Consent for publication
Not applicable.
Copyright
© The Author(s) 2024.
REFERENCES
1. Elder FR, Gurewitsch AM, Langmuir RV, Pollock HC. Radiation from electrons in a synchrotron. Phys Rev 1947;71:829.
3. Ice GE, Budai JD, Pang JWL. The race to X-ray microbeam and nanobeam science. Science 2011;334:1234-9.
4. Zhang XW, Yan XJ, Zhou ZR, et al. Arsenic trioxide controls the fate of the PML-RARα oncoprotein by directly binding PML. Science 2010;328:240-3.
5. Kern J, Alonso-Mori R, Tran R, et al. Simultaneous femtosecond X-ray spectroscopy and diffraction of photosystem II at room temperature. Science 2013;340:491-5.
6. Wu J. Understanding the electric double-layer structure, capacitance, and charging dynamics. Chem Rev 2022;122:10821-59.
7. Velasco-Velez JJ, Wu CH, Pascal TA, et al. Interfacial water. The structure of interfacial water on gold electrodes studied by x-ray absorption spectroscopy. Science 2014;346:831-4.
8. Ledezma-Yanez I, Wallace WDZ, Sebastián-Pascual P, Climent V, Feliu JM, Koper MTM. Interfacial water reorganization as a pH-dependent descriptor of the hydrogen evolution rate on platinum electrodes. Nat Energy 2017;2:17031.
9. Kolb DM. Electrochemical surface science this manuscript is based on the Bonhoeffer-Eucken-Scheibe lectures of the Deutsche Bunsengesellschaft, given by the author at Erlangen, Berlin, and Leipzig in 1999/2000. Angew Chem Int Ed 2001;40:1162-81.
10. Brown MA, Goel A, Abbas Z. Effect of electrolyte concentration on the stern layer thickness at a charged interface. Angew Chem Int Ed Engl 2016;55:3790-4.
11. Axnanda S, Crumlin EJ, Mao B, et al. Using “Tender” X-ray ambient pressure X-ray photoelectron spectroscopy as a direct probe of solid-liquid interface. Sci Rep 2015;5:9788.
12. Favaro M, Jeong B, Ross PN, et al. Unravelling the electrochemical double layer by direct probing of the solid/liquid interface. Nat Commun 2016;7:12695.
13. Strmcnik D, Kodama K, van der Vliet D, Greeley J, Stamenkovic VR, Marković NM. The role of non-covalent interactions in electrocatalytic fuel-cell reactions on platinum. Nat Chem 2009;1:466-72.
14. Subbaraman R, Tripkovic D, Strmcnik D, et al. Enhancing hydrogen evolution activity in water splitting by tailoring Li+-Ni(OH)2-Pt interfaces. Science 2011;334:1256-60.
15. Goyal A, Koper MTM. The interrelated effect of cations and electrolyte pH on the hydrogen evolution reaction on gold electrodes in alkaline media. Angew Chem Int Ed Engl 2021;60:13452-62.
16. Shah AH, Zhang Z, Huang Z, et al. The role of alkali metal cations and platinum-surface hydroxyl in the alkaline hydrogen evolution reaction. Nat Catal 2022;5:923-33.
17. Li P, Jiang Y, Hu Y, et al. Hydrogen bond network connectivity in the electric double layer dominates the kinetic pH effect in hydrogen electrocatalysis on Pt. Nat Catal 2022;5:900-11.
18. Lucas CA, Thompson P, Gründer Y, Markovic NM. The structure of the electrochemical double layer: Ag(111) in alkaline electrolyte. Electrochem Commun 2011;13:1205-8.
19. Sun Z, Yan W, Yao T, Liu Q, Xie Y, Wei S. XAFS in dilute magnetic semiconductors. Dalton Trans 2013;42:13779-801.
20. Huang J, Sementa L, Liu Z, et al. Experimental Sabatier plot for predictive design of active and stable Pt-alloy oxygen reduction reaction catalysts. Nat Catal 2022;5:513-23.
21. Kang J, Qiu X, Hu Q, et al. Valence oscillation and dynamic active sites in monolayer NiCo hydroxides for water oxidation. Nat Catal 2021;4:1050-8.
22. Bergmann A, Jones TE, Martinez Moreno E, et al. Unified structural motifs of the catalytically active state of Co(oxyhydr)oxides during the electrochemical oxygen evolution reaction. Nat Catal 2018;1:711-9.
23. Jia Q, Ramaswamy N, Hafiz H, et al. Experimental observation of redox-induced Fe-N switching behavior as a determinant role for oxygen reduction activity. ACS Nano 2015;9:12496-505.
24. Velasco-Vélez JJ, Falling LJ, Bernsmeier D, et al. A comparative study of electrochemical cells for in situ x-ray spectroscopies in the soft and tender x-ray range. J Phys D Appl Phys 2021;54:124003.
25. Diercks JS, Herranz J, Ebner K, et al. Spectroscopy vs. electrochemistry: catalyst layer thickness effects on operando/in situ measurements. Angew Chem Int Ed Engl 2023;62:e202216633.
26. Zitolo A, Ranjbar-Sahraie N, Mineva T, et al. Identification of catalytic sites in cobalt-nitrogen-carbon materials for the oxygen reduction reaction. Nat Commun 2017;8:957.
27. Hu J, Shang W, Xin C, et al. Uncovering dynamic edge-sites in atomic Co-N-C electrocatalyst for selective hydrogen peroxide production. Angew Chem Int Ed Engl 2023;62:e202304754.
28. Zhang M, de Respinis M, Frei H. Time-resolved observations of water oxidation intermediates on a cobalt oxide nanoparticle catalyst. Nat Chem 2014;6:362-7.
29. Dong JC, Zhang XG, Briega-Martos V, et al. In situ Raman spectroscopic evidence for oxygen reduction reaction intermediates at platinum single-crystal surfaces. Nat Energy 2019;4:60-7.
30. Mefford JT, Akbashev AR, Kang M, et al. Correlative operando microscopy of oxygen evolution electrocatalysts. Nature 2021;593:67-73.
31. Saveleva VA, Ebner K, Ni L, et al. Potential-induced spin changes in Fe/N/C electrocatalysts assessed by in situ X-ray emission spectroscopy. Angew Chem Int Ed Engl 2021;60:11707-12.
33. Cutsail GE III, DeBeer S. Challenges and opportunities for applications of advanced X-ray spectroscopy in catalysis research. ACS Catal 2022;12:5864-86.
34. Chen J, Finfrock YZ, Wang Z, Sham TK. Strain and ligand effects in Pt-Ni alloys studied by valence-to-core X-ray emission spectroscopy. Sci Rep 2021;11:13698.
35. Lancaster KM, Roemelt M, Ettenhuber P, et al. X-ray emission spectroscopy evidences a central carbon in the nitrogenase iron-molybdenum cofactor. Science 2011;334:974-7.
Cite This Article
How to Cite
Download Citation
Export Citation File:
Type of Import
Tips on Downloading Citation
Citation Manager File Format
Type of Import
Direct Import: When the Direct Import option is selected (the default state), a dialogue box will give you the option to Save or Open the downloaded citation data. Choosing Open will either launch your citation manager or give you a choice of applications with which to use the metadata. The Save option saves the file locally for later use.
Indirect Import: When the Indirect Import option is selected, the metadata is displayed and may be copied and pasted as needed.
About This Article
Special Issue
Copyright
Data & Comments
Data
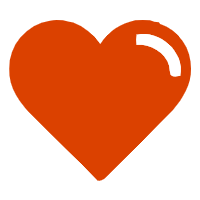
Comments
Comments must be written in English. Spam, offensive content, impersonation, and private information will not be permitted. If any comment is reported and identified as inappropriate content by OAE staff, the comment will be removed without notice. If you have any queries or need any help, please contact us at [email protected].