Interface chemistry for sodium metal anodes/batteries: a review
Abstract
Sodium metal batteries (SMBs), benefiting from their low cost and high energy densities, have drawn considerable interest as large-scale energy storage devices. However, uncontrollable dendritic formation of sodium metal anodes (SMAs) caused by inhomogeneous deposition of Na+ severely decreases the Coulombic efficiency, leads to short cycling life, and poses potential safety hazards, dragging SMBs out of practical applications. Electrolytes are attracting massive attention for not only providing ion transport channels but also exhibiting vital effects on interfacial compatibility and dendrite growth. In fact, the as-formed solid electrolyte interphase (SEI) has a great influence on the deposition and stripping process of SMAs. Moreover, Na plating process is accompanied by the generation of SEI, in which the electrolyte plays a vital role. Nevertheless, until now, the interaction among electrolyte-SEI-sodium dendrite has rarely been summarized. Herein, a fundamental understanding of sodium dendrite is concluded and the influence of the electrolyte and interface on Na+ deposition is emphasized. Furthermore, the outlook for constructing dendrite-inhibited SMAs is suggested.
Keywords
INTRODUCTION
In recent years, due to their low pollution and ability to regenerate, solar, geothermal, biomass, wind, and tidal power have been favored by researchers[1,2]. However, renewable energy has a short retention period, requiring strict energy storage, so the demand for high-efficiency energy storage devices is gradually increasing[3-5]. In contemporary battery technologies, owing to their excellent electrochemical properties and high energy densities, lithium-ion batteries (LIBs) have been widely used in portable electronic devices, electric vehicles, and energy storage power stations[6,7]. Nevertheless, LIBs with graphite as anode have reached their theoretical energy density, which is difficult to apply to large-scale energy storage. At the same time, the metal raw materials required by LIBs, such as lithium, cobalt, and manganese, are rare in the lithosphere, which is not conducive to expanding the production scale and lowering the cost[8]. Therefore, a new battery system is urgently needed to reduce battery cost and meet the expanding energy storage market[9-11].
With Na ranking seven most abundant and widely distributed in the Earth’s crust, sodium-ion batteries (SIBs) have the edge in resource reserve, cost, energy conversion efficiency, cycle life, stability, and maintenance cost[12-16]. Sodium metal has been reckoned as an ideal anode for SIBs among the available anode materials with a high theoretical capacity of 1166 mAh g-1 and a low redox potential of -2.71 V (vs. standard hydrogen electrode)[17-19]. Na metal can be paired with high-capacity sodium-free cathodes in particular, such as sulfur (S)[20], oxygen (O2)[21], carbon dioxide (CO2)[22], and selenium (Se)[23-25], to achieve the high theoretical energy density. Therefore, due to these multiple advantages, sodium metal batteries (SMBs) have attracted a lot of attention from researchers and are expected to be an alternative for the next generation of high-energy batteries[26-29].
Sodium metal is in a thermodynamically unstable state in the electrolyte, and the electrode surface is inclined to form a solid electrolyte interphase (SEI) film with ion conduction and electron insulation[30,31]. The SEI film can isolate sodium metal and electrolyte to a certain extent to prevent the continuous occurrence of side reactions. However, it cannot withstand the severe volume change caused by sodium deposition/stripping during the cycling, which will lead to the formation of tree-like or moss-like dendrites after uneven deposition on the surface of sodium metal[32]. Sodium dendrites can exacerbate side reactions, cause “dead sodium” generation, reduce Coulombic efficiency (CE), and increase battery polarization. In more severe cases, dendrites will pierce the SEI, leading to short circuit[33,34]. To make things worse, the accompanying thermal runaway will cause fires and even explosions, dragging SMBs out of commercial applications. Hence, strategies to inhibit dendrite growth to achieve safe SMBs have been strongly considered during the past few decades[35,36].
By surveying the recent literature, several review papers related to dendrite-inhibited SMBs have been published in recent years[37-40]. As is known, the deposition behavior of Na+ is closely related to the interface properties of sodium metal anodes (SMAs). Electrolytes are attracting massive attention for not only providing ion transport channels but also exhibiting vital effects on the interfacial compatibility of sodium anodes. However, until now, the interaction among electrolyte-SEI-sodium dendrite has rarely been summarized, and reviews about electrolyte regulation strategies in suppressing dendrite growth for SMBs have not been published thus far[41-43]. This review summarizes a fundamental understanding of sodium dendrite and then gathers various electrolytes, including the components, concentrations, and additives of liquid electrolytes and solid-state electrolytes technologies, to inhibit dendrite growth and the related SEI for dendrite-suppressed SMAs [Figure 1][44-52]. Finally, this review briefly presents a general conclusion and outlook for constructing high-performance and dendrite-suppressed SMBs.
UNDERSTANDING INTERFACE CHEMISTRY OF SODIUM ANODES
As is known, the existence of sodium dendrites is one of the toughest challenges for the commercial application of SMBs. It not only induces a low Coulombic efficiency and short cycling lifespan but also causes a poor safety profile for SMBs[53-55]. The generation of sodium dendrites is closely related to the formation of SEI and the resulting interfacial chemistry between SMAs and electrolytes. The nature of the SEI is believed to determine the plating/stripping behavior of sodium metals and the reversibility of batteries. In fact, electrolytes influence the formation and evolution of SEI. Therefore, controlling interfacial chemistry through electrolyte regulation plays an important role in the practical application of SMBs[56]. This section discusses a comprehensive understanding of the dendrite formation mechanisms and the interaction among electrolyte-SEI-sodium dendrite.
Formation mechanisms of sodium dendrite
In general, the deposition of Na+ occurs during the passage of Na+ through the SEI layer and the acquisition of electrons on the current collector, and this process is influenced by the inhomogeneous nucleation behavior[57]. During the deposition course, local electric field will induce an overpotential peak for non-homogeneous nucleation due to the uneven distribution of electric field caused by the impurities and defects in the sodium metal itself, which in turn will overcome the nucleation barrier and start the nucleation and deposition. The SEI is not sufficient to support the pressure brought by the sodium metal deposition and breaks into fragments around the deposition site to continuously expose fresh metal and generate a new SEI. Meanwhile, sodium metal tends to be deposited under the new SEI, gradually consuming sodium metal and generating dendrites. Although both sodium and lithium share many similarities, such as preferring to grow on the nucleation sites created by cracks, sodium dendrites are not just analogs of lithium dendrites[58]. The shapes of sodium dendrites are undefined but are frequently either needle or mossy structures. However, for lithium, these structures are concerned with defect catalyzed base growth conditions at low currents. In addition, they exhibit different hardness. With regard to Na, it is soft, which means it cannot penetrate separators as lithium does. As for Li, due to the greater hardness, lithium dendrite is inclined to penetrate the SEI, thus leading to the short circuit. Furthermore, the ion radius of Na is 55% larger than Li, which will lead to different SEI forming situations, thus working on dendrites[59,60]. Besides that, the lithium dendrite growth pattern can be accounted for by Sand’s time model. Nevertheless, it is difficult to explain sodium dendrite formation by using a formula, due to the lack of experimental data. Based on the current situation of SMB research, it is hard to form a continuous and complete SEI on the surface of sodium metal; sodium dendrites grow following a surface growth mechanism, i.e., they grow as particles over the entire sodium metal surface and even scatter in the pores of the diaphragm, thus causing a short circuit [Figure 2].
During the deposition process, the initial growth direction of sodium dendrites will be along the tip. In addition, dissolution of sodium dendrites is observed on the anode and, due to the electrostatic shielding effect, dissolution does not occur at the tip but preferentially at the bottom of the dendrite, where the disconnected low-density mossy electrodeposits are called “dead Na”. The dead Na will quickly drift to the electrolyte surface, and the detached moss (isolated) sodium fragments will fill the electrolyte and the diaphragm, eventually short-circuiting the cell. Consequently, for sodium, owing to its softness, the short circuit can be explained by the accumulation of dead Na.
Thus far, lithium dendrites have been extensively studied, and models such as tip growth, root growth, and surface growth have been proposed. However, as there is no existing appropriate model for sodium dendrite, we can turn to the lithium model for insight into the influencing factors, as lithium is similar to sodium to some extent. Akolkar put forward Equation (1) (where it represents tip current density and if represents flat surface current density), which shows that the higher the it/if ratio, the higher the dendrite propagation[61]. In addition, the cathodic transfer coefficient (αc), the bulk concentration of Li (C0), and b parameter all function on dendrite growth. Fast dendritic growth is favored when operating near the limiting current (if/iL is close to unity); however, slow but sustained dendritic growth can take place well below the limiting current density. Dendrite growth is suppressed in systems that exhibit a lower cathodic transfer coefficient[57].
The relationship between electrolyte-SEI-sodium dendrite
Electrolyte plays an important role in ion transport and affects the deposition morphology of metal anodes. Different electrolyte types have a major influence on dendrite appearance[20,47,62-69]. SEI layers will greatly affect dendrite formation. In liquid electrolytes, following the cycling of batteries, SEI layers will be easily formed, and the uneven morphology of sodium surface will contribute to the growth of dendrite[70]. The thicker SEI film exhibits better mechanical strength, conducive to inhibiting dendrite formation. Interestingly, CE will decrease because of the electrolyte consumption caused by SEI formation[71,72]. The thinner SEI cannot withstand the dendrite penetration despite showing high CE with little electrolyte consumption[73]. Therefore, the mechanical strength of SEI is important when considering the balance between CE and dendrite growth. Moreover, the component of SEI is also of importance for dendrite generation[74]. As mentioned above, during the Na plating/exfoliation process, an SEI film is produced. However, long-term, highly reversible, and non-dendritic electroplating stripping of Na anodes using diethylene glycol dimethyl ether with NaPF6 electrolyte led to SEI fracture due to its poor mechanical strength. Further characterization showed that a homogeneous and dense SEI consisting of Na2O and NaF was formed on the sodium metal surface, which was highly permeable to the solvent[17]. Homogeneity of the SEI promotes uniform Na+ flux and non-dendritic sodium, thus keeping the SEI intact and stable during cycling. Besides that, the concentration of electrolyte is worth considering. For instance, excessive NaF tends to form a more brittle SEI, which leads to CE fluctuations and dendrite growth in ether-based electrolytes. This factor is discussed in the subsequent section on high-concentration electrolytes[75]. Basically, in addition to the essential factors brought by the characteristics of SMBs, the influence of external conditions also significantly affects its growth[76]. Current density and charging capacity are the key parameters affecting dendrite morphology by influencing the formation of SEI[41,42]. Wenzel et al. showed that dendrites exhibit shrub-like growth with a current density of 120 µA cm-2, while, when increased to 240 µA cm-2, the dendrites demonstrate apparent dendritic growth[77]. It is well known that high current densities accelerate dendrite growth, but they also cause a shift from needle-like to moss-like sodium dendrites. One-dimensional needle-like dendrites are a safety hazard due to their growing length. Although three-dimensional mossy dendrites tend to grow in all directions, the lateral growth is dominant and usually results in more dead Na because the roots of sodium dendrites prefer to dissolve compared to the tips, which possess a strong electrostatic shielding effect[78]. Whichever dendrites are formed, the performance of the battery is severely affected. A further increase in current density, however, induces a further increase in the deposition overpotential, which results in a more nonuniform deposition of sodium metal. In addition to current density and capacity, temperature and internal battery pressure, referred to as the external causes, affect ion diffusion and then SEI formation, thus working on dendrite growth, which is not discussed further here because of the lack of research in SMBs.
LIQUIDE ELECTROLYTES
Liquid electrolyte is currently the most mature electrolyte system. Because of the loose and fragile SEI, with weak mechanical strength and dissolving in liquid electrolytes, unfavorable dendritic growth will emerge, leading to serious security trouble. Hence, a homogeneous, strong, and dense SEI is vital in protecting SMBs. Electrolyte additives, (local) concentrated electrolytes, and emerging novel electrolytes are regarded as some of the effective solutions in liquid electrolytes[79-81].
Electrolyte additives
Electrolyte additives are the most convenient solution to sodium dendrites. NaF, with high surface energy, possesses the strong ability to adjust surface tension and suppress dendrite growth. NaF is also intrinsically characterized by negligible solubility in most electrolytes and wide electrochemical stability windows[54]. Consequently, generated by F-, it can enhance the mechanical strength of SEI and promote uniform distribution of Na+. Currently, as the most extensively used additive, fluoroethylene carbonate (FEC) has been proven to be capable of promoting the formation of NaF-rich SEI and obtaining a compact deposition. However, FEC also tends to overreact with sodium, which constantly destroys and generates the SEI layer, consuming sodium and thus reducing CE. As a result, a new additive was added to the electrolyte to inhibit FEC from reacting with sodium by Zhu et al.[46] 1,3,2-Dioxathiolane 2,2-dioxide (DTD) and FEC were added as additives to the non-combustible electrolyte, and a stable fluoride SEI was generated in situ on the surface of the SMAs, effectively inhibiting dendrite growth and alleviating the occurrence of side reactions [Figure 3A]. DTD additive is conducive to forming a constitutionally stable SEI layer, which enables dendrite-inhibited Na anode.
Figure 3. Electrolyte additives regulate SEI for dendrite-inhibited SMAs. (A) Diagram of SEI layer formed in TMP/FEC-E with or without DTD. Reproduced with permission from[46]. Copyright 2021, Elsevier. (B) Diagram of C60(NO2)6-affected Na anode. Reproduced with permission from[82]. Copyright 2021, Elsevier. (C) Diagram of Na2S6 additive- and Na2S6-NaNO3 co-additive-affected Na anode. Reproduced with permission from[55]. Copyright 2018, Wiley-VCH. (D) Simulation of Na+ concentrations and deposition structures of Na metal anodes in the SEI layer. Reproduced with permission from[83]. Copyright 2020, The Royal Society of Chemistry. (E) Interfacial environment in the electrolyte with cation additives. (F) In situ optical microscopic observations of Na deposition on copper wire in 1.00 M NaPF6 DME and 1.00 M NaPF6 + 0.50 M LiPF6 DME. Reproduced with permission from[84]. Copyright 2018, Cell Press. SEI: Solid electrolyte interphase; SMAs: sodium metal anodes.
In addition to NaF additive, Li et al. showed that nitrofullerene [C60(NO2)6] additive, exhibiting good compatibility with different electrolytes, enabled highly reversible and dendrite-free SMAs [Figure 3B][82]. Consequently, they could promote the formation of stable protective layers on SMAs and facilitate uniform Na deposition, thus effectively suppressing Na dendrite growth. Moreover, Wang et al. used sodium polysulfide (Na2S6) alone as a prepassivation agent to improve the long-term reversibility of Na anode; the concomitant stable interface could inhibit the dendrite formation[55]. However, Na2S6-NaNO3 as co-additive had the opposite result, and the as-formed SEI with a component of sodium alkoxide (RCH2ONa) and Na2S gave rise to dendritic/mossy Na growth [Figure 3C]. Chen et al. constructed fast Na+ diffusion SEI with different interface compositions through the interaction of sodium and tin chloride, and the results show that the interface of symbolic inorganic components had better structural stability and cycling performance [Figure 3D][83]. This provides guidance for the selection of additives for SMBs.
In addition to the above additive strategies for regulating SEI, a reasonable and feasible cationic additive strategy for stabilizing SMAs was proposed [Figure 3E][84]. Due to the electrostatic shielding effect, the electrolyte containing cationic additives gas evolution was prevented to a certain extent, and the anode appeared smooth [Figure 3F]. Through first-principles calculation and molecular dynamics simulation, three principles were proved, including electrode potential, LUMO level reduction, and binding energy with solvent. Mature cationic additive strategies provide new opportunities for the rational design of electrolytes for stable and safe SMBs.
(Local) concentration electrolytes
Salt concentration in the electrolyte has an enormous influence on improving electrochemical performance and suppressing dendrite growth. Increasing the electrolyte concentration can form a unique solvated structure and reduce the reaction between sodium and electrolyte, thereby reducing the formation of dendrites. Regarding the illustration of concentration effect, solvation structure and interfacial structure are deemed to influence the electrolyte properties as well as electrode-electrolyte interfaces that together can affect the battery performance. Figure 4A depicts two models, the superhigh concentration model and the ultralow one, which show that the former will get an anion-derived SEI and the latter will achieve a solvent-derived SEI[85]. From that perspective, Ma et al. used different concentrations of NaCF3SO3 dissolved in TEGDME solvent as electrolytes for Na-O2 batteries and tested their electrochemical properties for comparison[86]. The results show that the stability of the electrolyte lowered with decreasing concentration. Thus, the stability of the Na cell was substantially improved when a saturated electrolyte was used. This is mainly due to the enhanced corrosion resistance of the sodium anode, reduced oxygen solubility, and effective inhibition of Na dendrite growth at the mercy of the high-concentration electrolyte [Figure 4B]. This work not only brings new insights to advance the development of long-life sodium-oxygen batteries but also stimulates additional studies to gain fundamental insights into the high concentration electrolytes for metal-oxygen batteries.
Figure 4. Local concentration electrolytes regulate SEI for dendrite-inhibited SMAs. (A) Solvation and interfacial structures of superhigh and ultralow concentrated electrolytes. Reproduced with permission from[85]. Copyright 2020, American Chemical Society. (B) Voltage profiles for Na/Na symmetric cells running in O2 with NaCF3SO3/TEGDME electrolytes in various concentrations. Reproduced with permission from[86]. Copyright 2021, Elsevier. (C) Diagram of dilution from a HCE to a LHCE. (D) Atomic ratios of SEI layer after the 10th stripping of Na//Cu cells using dilute, HCE, and LHCE. Reproduced with permission from[45]. Copyright 2018, American Chemical Society. (E) Diagram of interface behavior Na+ deposition. (F-H) Deposition morphology of sodium anode with different concentrations of electrolyte. (E-H) Reproduced with permission from[88]. Copyright 2021, Elsevier. SEI: Solid electrolyte interphase; SMAs: sodium metal anodes; LHCE: local high-concentration electrolyte.
High-concentration electrolyte can solve the problem of dendrite growth caused by the strong reaction nature of sodium and electrolyte, but the high viscosity, excessive cost, and poor wetting of HCE also limit its application[87]. As shown in Figure 4C, by adding inert solvents such as double (2,2,2-trifluoroethyl) ether (BTFE) to the HCE, BTFE does not react with sodium metal and can form a local high-concentration electrolyte (LHCE) without changing the solvation structure[45]. LHCE not only provides high thick electrolyte contact with metal sodium to reduce corrosion but also forms effective wetting between sodium and cathode material, providing a good interface reaction. In this study, LHCE [2.1 M NaFSI/DME-BTFE (1:2)] could achieve a high CE of > 99%, compared with other electrolytes in different concentrations. XPS analysis disclosed that more FSI anions participated in the formation of a more robust SEI layer when Na metal was cycled in either HCE or LHCE, thereby mitigating the consumption of solvent molecules during repeated Na deposition stripping processes [Figure 4D].
In addition, LHCE with a molar ratio of NaFSI:DME:OTE (1H,1H, 5H- octafluoropentyl -1,1,2, 2-tetrafluoroethyl ether) = 1:1.5:3 was used as the model electrolyte to in situ construct inorganic SEI films with gradient distribution on the surface of sodium anode [Figure 4E][88]. In the presence of OTE, the solvation configuration of the electrolyte changed from 3D network aggregation in HCE to cationic and anion solvent clusters in LHCE, which promoted more anions to enter the first solvated sheath. The overall migration mechanism of cation and anion solvent clusters in the electrolyte resulted in the enrichment of a large number of anions on the surface of Na, which promoted the gradual decomposition of FSI- anions, thus forming the inorganic rich and gradient distribution of ultra-thin SEI, such as NaF, NaSON, Na2S, etc., which further reduced the activation energy of Na+ transport in the SEI film. These advantages made Na||Na3V2(PO4)3 battery exhibit different morphology [Figure 4F-H]. This study provided new insights into developing high energy density SMBs by regulating the electrode-electrolyte interface membrane.
Novel electrolytes
In the above discussion, we summarize the effects of additives and concentrations on the interface in the regulation of liquid electrolytes. Most of the currently available organic electrolytes form SEI with continuity and inhomogeneity, as well as more severe dendrite growth now. Hence, it is very important to regulate the interface from the composition of electrolytes (salts and solvents)[89]. As shown in Figure 5A, Sun et al. designed the first chloroaluminate ionic liquid electrolyte for rechargeable SMBs by adding two important additives (ethylaluminum dichloride and 1-ethyl-3-methylimidazolium bis(fluorosulfonyl)imide) to an ionic liquid consisting of AlCl3/1-methyl-3-ethylimidazolium chloride/NaCl[90]. The system exhibited good inflammability and the absence of obvious dendrites. Meanwhile, they found that the formation of stable SEI is related to the existence of FSI anions, but the single presence of FSI anions is not sufficient to support metal Na anode for long-term charging and discharging cycles. Furthermore, a novel, dual electrolyte consisting of β”-alumina solid electrolyte (BASE) and an inorganic IL, Na[OTF]-Cs[TFSA], was integrated by Wang et al. [Figure 5B][91]. Investigations revealed IL exhibited high ionic conductivity and excellent thermal and chemical stability, making it propitious for intermediate-temperature operations. After 400 cycles, no significant change was observed in the interfacial resistance, which meant no dendrite formation. Ruiz-martínez et al. designed a liquid-amino electrolyte with diverse dissolved sodium salts[92]. This electrolyte had excellent properties of low flammability and high electrical conductivity. Most notably, using this electrolyte can effectively inhibit dendrite growth on the surface of SMAs, enabling highly reversible plating/stripping. In addition, the same high CE and cycling stability could be achieved by sodium deposition on copper foil [Figure 5C]. Zhou et al. designed a new type of sodium liquid metal battery, which used low melting point ternary mixed cationic chloride molten salt LICL-NACL-KCl (59:5:36 mol%) as the electrolyte, greatly reduced the operating temperature and sodium halide content of the battery, and effectively inhibited the dissolution of SMAs in the electrolyte[93]. At the same time, the utilization rate of electrode materials was effectively improved through the construction of Bi-Sb binary alloy electrode, and the 10 Ah battery maintained a Coulomb efficiency of about 97% and ran stably for more than 700 cycles [Figure 5D]. The exploration and research of new electrolytes provide infinite possibilities for the realization of high-performance SMBs. The development of new electrolytes starts from scratch, so their development is relatively slow. In the future, new salts and solvents should be designed to regulate the interface chemistry of Na anode and thus achieve high-performance SMAs.
Figure 5. Novel electrolytes regulate SEI for dendrite-inhibited SMAs. (A) Diagram of the battery configuration and IL electrolyte. Reproduced with permission from[89]. Copyright 2019, Nature Publishing Group. (B) Operation mechanisms of Na-S battery using dual BASE/IL electrolyte. Operation temperature, 150 °C; IL, Na[OTF]-Cs[TFSA]. Reproduced with permission from[91]. Copyright 2021, Wiley-VCH. (C) CV for Na deposition/stripping over Na (supported on Cu) in a three-electrode cell. Reproduced with permission from[92]. Copyright 2017, The Royal Society of Chemistry. (D) Structure diagram of Na-LMBs; charge capacity, discharge capacity, Coulombic efficiency, and energy efficiency as a function of cycle number; and the theoretical capacity is 10 Ah. Reproduced with permission from[93]. Copyright 2022, Elsevier. SEI: Solid electrolyte interphase; SMAs: sodium metal anodes.
Table 1 shows the performance of representative batteries assembled with liquid electrolytes in the recent literature. Liquid electrolyte is the most used battery system. It has high current density and cycle life. However, its security severely limits the stable cycle for a long time. As a result, the solid-state battery is the future development trend to improve the safety performance of batteries.
Performance of representative batteries assembled with liquid electrolytes
Electrolyte | Current density (mA cm-2) | Stripping capacity (mAh cm-2) | Cycles hours (h) | CE (%) | Refs. |
1.0 M NaPF6 FEC/PC + HFE | 0.5 1.0 5.0 1.0 | 0.5 1.0 1.0 5.0 | 1100 570 200 800 | 94.2 | [44] |
2.1 M NaFSI DME/BTFE | 1.0 2.0 | 1 | 950 950 | 98.9 | [45] |
0.8 NaPF6 FEC + DTD | 0.5 1 | 1 5 | 1350 720 | 93.4 | [46] |
1 M NaPF6/DGM + 0.033 M Na2S6 | 2 5 | 1 1 | 400 60 | 99 | [55] |
1.0 M NaDFOB EC/DMC | 1.5 | 0.1 | 34 | - | [72] |
4 M NaFSI/DME + 1% SbF3 | 0.5 | - | 1000 | - | [74] |
1 M NaPF6 DME + 0.5M C60(NO2)6 | 0.5 0.5 2.0 | 0.5 1.0 2.0 | 500 400 1200 | 99.4 | [82] |
0.8 M LiPF6 DME + 1.0 M NaPF6 | 0.5 2.0 | 0.5 2.0 | 300 300 | 99.2 | [84] |
1.6 M NaCF3SO3 TEGDME | 0.5 | 2.0 | 800 | - | [86] |
1 M NaPF6 FEC/DMC + DMTP | 1 5 | 1 1 | 900 250 | 96.6 | [94] |
0.3 M NaPF6/EC/PC + 2 wt % BSTFA | 0.5 | 0.5 | 350 | - | [95] |
5 M NaFSI DME | 0.0028 | 0.0014 | 600 | 99.3 | [96] |
1 M NaPF6 DGM | 0.5 | 1 | 300 | 99.9 | [97] |
0.64 M NaBF4/G2 | 0.5 1 | - | 3000 2000 | 99.9 | [98] |
0.8 M NaPF6 DME + 0.4 M LiPF6 | - | - | - | 97.0 | [99] |
DME:NaFSI:TTE = 1:1.2:1 | 1 | 1 | 590 | - | [100] |
SOLID-STATE ELECTROLYTES
Many safety problems emerge in liquid electrolytes, such as electrolyte volatilization and overreaction with sodium anode[101-105]. While these problems do not exist in solid-state batteries, the growth of sodium dendrite, serious interface problems between the surface of sodium and solid electrolyte, and the ion conductivity at low room temperature are all urgent problems for solid-state electrolytes. Commonly used solid-state electrolytes are mainly divided into inorganic electrolytes and polymer electrolytes[106]. Inorganic electrolytes represented by inorganic ceramics have high ionic conductivity and firm thermal stability but poor mechanical properties, and defects in inorganic materials themselves may aggravate sodium dendrite growth[107,108]. Polymer electrolytes provide an acceptable interface with their excellent flexibility, whereas low ionic conductivity and parasitic reactions with sodium make them unsuitable to SMBs[109]. Hence, conventional inorganic electrolytes can be combined with polymer electrolytes to form hybrid inorganic-polymer electrolytes, which are capable of overcoming the disadvantages of polymer and inorganic electrolytes, meaning high ionic conductivity and favorable electrode-electrolyte interface. Moreover, the improved mechanical strength of hybrid inorganic-polymer electrolytes, due to inorganic binding to polymer electrolytes, can also inhibit the formation of sodium dendrite[110-112].
Polymer electrolytes
The limitations of liquid electrolytes, such as sodium dendrite growth, serious side reactions, and liquid leakage, hinder the development of SMBs. However, these safety issues will not readily happen in organic polymer electrolytes. However, low ionic conductivity is an issue for conventional polymers. Polyether, especially polyethylene oxide, has attracted extensive attention in polymer electrolytes since 1973 due to its superior coordination ability with alkali metal ions. Chen et al. optimized the ratio of 3-{2-[2-(2-methoxyethoxy)ethoxy]-ethoxy} methyl-3-methyloxetane (MEMO) to 3-hydroxymethyl-3-methyloxetane (HMO) monomers [Figure 6A], in which the former provides conductive suspension and the latter supplies cross-linked branched chain sites, thus forming a polyether-based hyperbranched copolymer electrolyte with both high ionic conductivity and favorable mechanical strength[113]. Furthermore, the concentration of NaTFSI plays a great role in ionic conductivity of the electrolyte, making up for its shortcomings. Similarly, as observed in Figure 6B, Yao et al. designed an advanced all-solid-state sodium battery (NVP@C|PEGDMA-NaFSI-SPE|Na) to achieve high ionic conductivity[49]. UV-cured novel solid polymer electrolyte displayed enhanced ionic conductivity (≈ 10-4 S cm-1 at RT) and interfacial contact. The dendrite growth on the surface of sodium electrode was inhibited significantly. In addition, a solid-state polymer electrolyte (PFSA-Na) was fabricated by a facile ionic exchange from a raw commercial material
Figure 6. Polymer electrolytes regulate SEI for dendrite-inhibited SMAs. (A) Schematic conformation of PEO and PMH. Reproduced with permission from[113]. Copyright 2020, Elsevier. (B) The construction architecture of the NVP@C|PEGDMA-NaFSI-SPE|Na. Reproduced with permission from[49]. Copyright 2020, Wiley-VCH. (C) Diagram of the ionic-exchange process. (D) Surface morphology of sodium metal plates after 20 cycles in SSIB and LSIB. (C and D) Reproduced with permission from[114]. Copyright 2020, Wiley-VCH. (E) Schematic of in situ preparation process for PBA-based GPE in NVP||GPE||Na SMBs. Reproduced with permission from[120]. Copyright 2022, Wiley-VCH. SEI: Solid electrolyte interphase; SMAs: sodium metal anodes.
For challenges related to the contact between anode and polymer electrolytes, Zhao et al. showed that an improved interface was established between a composite Na/C metal anode and the solid PEO electrolyte, which led to more homogeneous plating and stripping, reducing/suppressing dendrite formation[115]. A series of star-like hyperbranched polymers composed of a β-CD core and multiple PMMA-b-PEGMA arms were synthesized through the ATRP method, and free-standing and flexible SPE films were prepared by complexing the resultant polymers with NaSO3CF3 salt in a THF solvent and casting the resulting solution[116]. The polymer exhibited excellent ionic conductance, thermal stability, and a wide electrochemical window. This provides a new direction for the development of new and safe sodium batteries. Polymer electrolytes show excellent performance and can inhibit dendrite formation to a certain extent. This also indicates the future market prospects for polymer electrolytes.
Gel polymer electrolytes (GPEs) composed of polymer matrix and liquid electrolytes have attracted extensive attention in SMBs. The liquid parts provide high ionic conductivity and low interfacial impedance, while the polymer matrix presents excellent mechanical properties and flexibility. Unfortunately, GPEs still face the problems of unstable electrolyte interface and dendrite growth. Therefore, the design and fabrication of advanced gel electrolytes are of the utmost importance for the realization of safe SMBs with excellent performance[117,118]. Wen et al. designed a new photopolymerized gel electrolyte of ETPTA-NaClO4-QSSE, which exhibited unprecedented room-temperature ionic conductivity of
Considering liquid electrolytes employed in batteries give rise to safety hazards such as fire and explosions in an abnormal state, Park et al. devised noncombustible GPEs formed by in situ cross-combination of polypropanolactone triacrylate gel precursors[48]. Although GPEs exhibited a 3D network structure, their ionic conductivity can reach 6.3 mS cm-1 due to the interaction between Na+ and carbonyl and high segmental motion of the polycaprolactone chain. The polymer network with high ionic conductivity could significantly inhibit Na dendrites by bringing in uniform Na deposition, thus improving the interface properties of Na electrode. In addition, Zheng et al. prepared three novel cross-linked gel tricopolymer electrolytes with different copolymers by in situ radical polymerization[121]. The GF separators with GPEs were uniform and showed outstanding integrity, whereas the one with LE manifested that a lot of small particles adhered to the fiber surface. These granules were speculated as dead Na, causing the capacity of the liquid battery to degrade or even short circuit after long cycles.
Inorganic electrolytes
The currently used inorganic SSEs are mainly divided into oxide and sulfide inorganic SSEs. For the former, the traditional oxide electrolyte exhibits high mechanical strength and good dendrite inhibition performance, but the ionic conductivity is low, and the interface contact is unstable. The latter, sulfide electrolytes, have attracted great attention these years, but the shuttling effect and instability are serious problems.
Oxide-type solid-state electrolytes
Poor interface contact is an urgent problem for inorganic solid electrolytes. Lai et al. demonstrated that a β”-Al2O3 electrolyte with a vertically porous bilayer structure [Figure 7A] could solve this issue[50]. The carbon-coated vertically porous β”-Al2O3 layer provided fast electronically and ionically conductive pathways, preventing sodium dendrite from penetration. In addition, β”-Al2O3 showed very good thermal stability, and its excellent sodium ion conductivity at high temperatures made it commonly used in high-temperature batteries. However, at room temperature, the sodium ion conductivity of the β”-Al2O3 is at the mercy of the content of Na2O in materials. To make β”-Al2O3 exhibit acceptable sodium ion conductivity, Bay et al. showed that, by applying a heat treatment in an argon atmosphere to a finely polished Na-β”-alumina surface, a low interfacial resistance to sodium metal of < 10 Ω cm2 could be achieved [Figure 7B][122]. There was a significant decrease in the interfacial resistance from 10,000s Ω cm2 for non-heat-treated samples to 8 Ω cm2, achieved by heat treating the Na-β”-alumina pellets at 900 °C. Therefore, the high ionic conductivity of the Na-β”-alumina ceramic at room temperature is available (1.6 mS cm-1). Nevertheless, at room temperature, more issues remain to be solved, as β”-Al2O3 shows lower mechanical properties and more defects (crystal boundaries, pores, etc.) which cannot inhibit the growth of sodium dendrite. In addition, its low sodium affinity is not suitable for normal temperature SMBs.
Figure 7. Inorganic electrolytes regulate SEI for dendrite-inhibited SMAs. (A) Schematic of the bilayer β”-Al2O3 electrolyte structure. Reproduced with permission from[50]. Copyright 2021, Elsevier. (B) Impact of heat-treatment temperature on interfacial resistance at room temperature. (left) Schematic of Na/Na-β”-alumina/Na cell and equivalent circuit for fitting the impedance spectra. (middle) Representative Nyquist plots for the different heat-treatment temperatures. Markers represent experimental data and lines represent the fitted data. (right) Interfacial resistance according to heat-treatment temperature. Reproduced with permission from[122]. Copyright 2020, Wiley-VCH. (C) Crystal structure view of Na3.4Mg0.1Zr1.9Si2.2P0.8O12. Reproduced with permission from[120]. Copyright 2021, Elsevier. (D) Diagram of the ultrasound solid welding method. Reproduced with permission from[126]. Copyright 2021, Nature Publishing Group. (E) (left) SEM-EDS mapping images of NZSP-0.2Mg against sodium metal after cycling. (right) Confined Na plating at the interface of Na/NZSP-0.2Mg. Reproduced with permission from[127]. Copyright 2021, Wiley-VCH. (F) (top) Diagram of the reactive Na/sulfide-based SSEs interface and stable Na@alucone/sulfide-based SSEs interface. Top-side SEM of the cycled Na|Na3SbS4|Na using (bottom left) bare Na and (bottom right) Na@mld 150 C electrodes. Reproduced with permission from[129]. Copyright 2021, Wiley-VCH. SEI: Solid electrolyte interphase; SMAs: sodium metal anodes.
NASICON is the first reported inorganic solid-state oxide electrolyte, in which Na3Zr2PSi2O12 (NZSP) is the most typical sodium superionic conductor, providing an ionic conductivity of about 10-4 S/cm at room temperature. Moreover, NASICON is also capable of providing high chemical and electrochemical stability, with a wide voltage window (> 5 V) for SMBs. However, the large crystal boundary impedance of the material itself makes the sodium easily grow along the crystal boundary, and the crystal boundary impedance also makes NASICON mostly exhibit poor ionic conductivity[123]. By making the graphene interface layer on the NZSP surface with CVD method, many distributed defect networks were constructed, so that the uniform deposition of metal sodium at the electrolyte and electrode interface could inhibit the growth of Na dendrite and reduce the interface impedance[124]. An integrated structure composed of dense electrolyte layer and porous sodium layer can also effectively reduce the contact resistance between electrolyte and sodium anode and effectively suppress the growth of sodium dendrite.
The dendrite suppressing ability for primitive NZSP is poor. Therefore, Weng et al. showed that the optimized Na3.4Mg0.1Zr1.9Si2.2P0.8O12 electrolyte was obtained by simultaneously substituting the Zr4+ with Mg2+ and P5+ with Si4+ through solid-state reaction[125]. The improved dendrite regulating performance can be explained by the monoclinic structure [Figure 7C], which forms an effective three-dimensional Na+ transport network[125]. Furthermore, the problem of contact between Na and NZSP can be tackled by a room temperature ultrasound solid welding strategy [Figure 7D][126]. With the aid of ultrasound, an intimate bonding interface with a stable interfacial layer was constructed, effectively alleviating dendrite growth. Conversely, the grain boundary phase of a Mg2+-doped NZSP conductor (denoted as NZSP-xMg) was manipulated to introduce favorable Na3-2δMgδPO4-dominant interphase, which facilitated its intimate contact with Na metal and worked as an electron barrier to suppress Na metal dendrite penetration into the electrolyte bulk. As shown in Figure 7E, sheet-like sodium metal was observed to adhere to the surface of NZSP-0.2Mg, and no sodium dendrites appeared[127]. The Na plating mechanism showed that, in NZSP-0.2Mg, the electron-insulted Na3-2δMgδPO4 was introduced to suppress electron transport through the grain boundary and helped to form desirable interphase, which ensured robust Na plating/stripping cycles constrained at the interface. In conclusion, the oxide solid-state electrolyte crystal boundary impedance is so enormous that it is necessary to improve the material density, reduce the crystal boundary impedance, usually through high-temperature annealing, and improve the solid-solid interface between the electrode and electrolyte.
Sulfide solid-state electrolytes
Sulfide plays an important role in inorganic solid electrolytes, which is generally soft in texture and obtained with high energy density and low interface resistance without high-temperature annealing. As the electrostatic effect between sulfur and sodium ions is weak, it can provide higher ion conductivity than the oxygen group. The sulfur group and the modified selenium-based solid electrolyte generally provide an ionic conductivity of 10-3 S cm-1, which is much higher than the oxygen-based solid-state electrolyte. The reported Na2.9Sb0.9W0.1S4 showed the highest ionic conductivity and provided 4.1 ± 0.8 × 10-2 S cm-1 at room temperature. Sulfur-based solid-state electrolyte is therefore considered one of the SSEs with commercial promise. However, the high reactivity and weak electrochemical stability between sulfur solid electrolyte and sodium will lead to the decomposition of sulfur solid electrolyte, which in turn causes uneven deposition of sodium and produces sodium dendrite. Therefore, a stable interface layer is usually added to the negative pole side[128].
A molecular layer deposition (MLD) alucone film was employed on the surface of sodium, which passivated the surface of anode, effectively alleviating the growth of sodium dendrite while preventing the breakdown of Na3SbS4, a solid electrolyte. However, for sulfide-based electrolytes, an inherent problem is that metallic sodium reacts thermodynamically with most thiophosphates to form poor ionic conducting products. Although substitution of P can improve electrochemical performance, those elements that contribute to improved ionic conductivity and chemical stability (As, Sb, etc.) have redox activity, which directly leads to the decomposition of electrolytes in SMB. Therefore, Zhang et al. used alucone films deposited on Na foil to separate Na metal and sulfide electrolytes (as shown in Figure 7F)[129]. In the absence of MLD coating, continuous protruding particles appeared on the surface of the exposed Na metal electrode, indicating the growth of Na dendrites. In contrast, Na@mld 150 C had a flat and smooth surface, showed evidence of limiting Na dendrites and was in close contact with the Na3SbS4 electrolyte. Inserting CPEO, an electrolyte membrane between sodium and Na3SbS4, prevents electron transport from reducing solid-state electrolyte surface defects, thus forming a uniform surface on sodium metal surface and inhibiting dendrite growth.
Hybrid inorganic-polymer electrolytes
Solid electrolytes, considered as promising electrolytes which exhibit much less danger than liquid electrolytes in SMBs, cannot contact thoroughly with the anode/cathode. Modification of inorganic electrolytes can enhance the ion conductivity at room temperature and suppress dendrite growth through coating. Nonetheless, inorganic electrolytes are costly and usually not stable enough in air, thus are not conducive to the development of commercialization. Correspondingly, polymer electrolytes generally exhibit strong flexibility, are inexpensive, easy to prepare, and are more likely to form a tight electrode-electrolyte interface with them.
Inorganic fillers are introduced into polymer electrolytes to form hybrid inorganic-polymer electrolytes. The hydroxyl and functional groups with other characteristics are brought into hybrid electrolytes, such as oxygen vacancy available with Lewis acids and Lewis bases on the polymer chain or sodium salt formation interaction, which transforms the crystallinity of polymers, increases freed Na+ concentration, improves stability and mechanical modulus of polymer electrolytes, etc. It is expected to solve the above key problems of low ionic conductivity and mechanical property.
Different materials have been used in the hybrid electrolyte system, including 0D nanoparticles, 1D nanowires, 2D layered materials, and 3D continuous packing structures[130-135]. Nano-sized NZSP powders were first prepared by Niu et al. with a sol-gel method[136]. As shown in Figure 8A, there are many pores in the cathode without succinonitrile (SN), and the interface contact between the cathode and solid electrolyte is poor, which hinders the transport of Na+ at the cathode and electrolyte-cathode interface. The addition of SN is conducive to the formation of a compact and stable contact interface between nanocomposite cathode and solid electrolyte, improves ion transport, and increases battery cycle life. As shown in Figure 8B, a composite electrolyte with sandwich heterostructure was designed, and the electrochemical window was widened to 5 V[51]. A continuous interface can be formed between the 3D interconnected NZSP and the polymer matrix, facilitating rapid diffusion of sodium ions. The integrated 3D structure is permeated by PAN or PEO. The porous side filled with PAN can withstand oxidation at high voltage, while the porous side filled with PEO can withstand Na metal reduction at low voltage. The battery composed of
Figure 8. Hybrid inorganic-polymer electrolytes regulate SEI for dendrite-inhibited SMAs. (A) Proposed Na+ transport model schematic in the composite electrolytes and illustration of the cathodes. Reproduced with permission from[136]. Copyright 2020, Elsevier. (B) Schematic representation of composite electrolyte. Reproduced with permission from[51]. Copyright 2021, Elsevier. (C) Schematic of ion pathway in NZSP-PEO CE. Reproduced with permission from[137]. Copyright 2021, American Chemical Society. (D) Structure of ANS-GPE composite electrolyte and mechanism of sodium ion transport. (E) Schematic of NVP/Na batteries using ANs-GPE and GFs-GPE electrolytes. (D and E) Reproduced with permission from[47]. Copyright 2016, Nature Publishing Group. (F) Optical microscope images of pristine Na and its morphologies after 100 h plating/deposition when coupled with 2-GPH GPE and LE. Reproduced with permission from[142]. Copyright 2020, Wiley-VCH. SEI: Solid electrolyte interphase; SMAs: sodium metal anodes.
A low-cost and cross-linked gel-polymer electrolyte of poly(methyl methacrylate) (PMMA) was compounded, enabling dendrite suppression and excellent cycling stability[117]. In addition to organic cross-linked polymers, Lei et al. developed a blended inorganic ionic conductor/GPE composite electrolyte, where a β/β”-Al2O3 nanowire (AN) membrane possessing 78.1% β”-phase was tightly wrapped by PVDF-HFP-based GPE, effectively inhibiting the growth of Na dendrite and successive side reactions of the Na metal anode [Figure 8D][47]. This study for the first time proved the efficient inorganic solid electrolyte ion conductor/polymer composite gel transmission behavior of sodium ions with inorganic ion conductor in its solid-liquid mixture of sodium ion transport mechanism, and illustrated the high efficient inorganic ion conductor matrix composite gel electrolyte for building an important highly stable solid SMB system. It provides a universal strategy for achieving high energy density, long life, and high safety of SMBs [Figure 8E].
Different materials can be mixed into gels to enhance mechanical strength and form an excellent interfacial matrix on the anode. As observed in Figure 8F, doping graphene oxide (GO) into PVDF-HFP generates many amorphous regions during the gel electrolyte generation to enhance sodium ion conductivity and form a uniform and smooth surface[140]. Meanwhile, the large number of oxygen-containing groups provided by GO can effectively anchor the sodium ions in the electrolyte and promote rapid and uniform sodium ion transport. Moreover, the strong H-F hydrogen bond between GO and PH can significantly increase Young’s modulus of the gel electrolyte and effectively inhibit the growth of sodium dendrites. As a result, uniform sodium deposition and ultra-long reversible sodium plating/exfoliation at high current density (5 mA cm-2) are achieved. Furthermore, the photoinitiator benzophenone (BP) and nanometer filler HKUST-1 were imported into the PEO matrix, thus acquiring a composite electrolyte by UV curing[141]. For PHGE (PEO + 5.0 wt.% HKUST-1 gel electrolyte), comparatively coarse surfaces could be noticed even after many cycles. The addition of HKUST-1 convincingly fixed the anions in the battery, stabilized the interface, facilitated the even deposition of Li/Na, and significantly improved the cyclic stability of symmetrical batteries, leading to uniform Na deposition and high sodium transport.
With the progress of gel polymer, novel electrolytes are being developed all the time. Wang et al. showed that, due to the existence of loose and multilayered structure of the Ti3C2Tx MXene filler, the electrochemical properties of the battery were greatly enhanced, as the filler could expand the porosity of the polymer films and augment the contact sites of sodium ions[65]. After 300 cycles, lichen-like sodium dendrites were viewed on the Na anode with GPE-0 (no Ti3C2Tx). In contrast, no pronounced dendrites were noticed on the one with GPE-8, while the sodium ions were evenly deposited on its anode. Although gel electrolyte can inhibit the growth of dendrites to a certain extent, the liquid electrolyte it contains may also pose a safety hazard. Moreover, gel electrolytes generally need to be operated at higher temperatures, so cells that can cycle stably at RT are yet to be developed. In addition to GO and MXene, some 2D materials (BN, clay, etc.) have also been used as electrolytes in battery systems to improve ionic conductivity and inhibit dendrite growth[141,142]. Hamisu et al. fabricated an hBN-SPSU(Na)/PPEGMA hybrid electrolyte based on polysulfone-sodium sulfonate [SPSU(Na)] blended with poly(polyethylene glycol methacrylate) (PPEGMA) using nano-sized hexagonal boron nitride (nano-hBN) as filler[142]. Homogeneous dispersion of hBN not only improves the mechanical properties of polymer electrolytes but also enhances ionic conductivity. The addition of new 2D materials provides a new idea for the preparation of high-performance electrolytes. Through the hybrid effect, solid electrolytes present high ionic conductivity, wide stable potential windows, excellent mechanical properties, and good interface binding ability, thus meeting the requirements of practical application of solid batteries.
Table 2 presents the performance of representative batteries assembled with solid electrolytes in the recent literature. Solid electrolytes have high mechanical properties, wide electrochemical windows, and excellent thermal stability. They can also inhibit dendrite growth and promote the development of metal battery commercialization. However, due to their poor interfacial contact and low working current density, it is still necessary to spend a lot of energy to develop high-performance solid electrolytes systems.
Performance of representative batteries assembled with solid electrolytes
Material | Ionic conductivity (mS cm-1) | Na+ transference number | Electrochemical window (V) | Current density (mA cm-2) | Cycles hours (h) | Refs. |
β/β”-Al2O3 ANs + PVdF-HFP | 1.07 | 0.37 | - | 0.5 | 300 | [47] |
8 wt % PCL-TA + 15 vol % TMP | 6.3 | - | 4.5 | 0.5 | 400 | [48] |
PSTB + PVCA | 0.1 | 0.88 | 4.5 | 0.1、0.25、0.5、1 | 620 | [62] |
PVDF-HFP/PMMA + 8wt %Ti3C2Tx MXene | 3.28 | 0.558 | 5.25 | 0.5 | 300 | [65] |
PFSA-Na | 0.159 | - | 4.7 | 0.5 | 300 | [114] |
ETPTA-QSSE | 1.2 | 0.62 | 4.7 | 0.1 | 1000 | [119] |
PBA | 1.6 | 0.39 | 4 | 0.2 | 900 | [120] |
MADEMP-AEDEP-EA | 3.37 | 0. 52 | 4.9 | 0.2 | 700 | [121] |
HKUST-1/PEO GO/NASICON | 0.6 0.6 | 3.48 - | 5.17 - | 0.2 0.5 | 1000 1000 | [122] [124] |
NZSP-xMg | 0.15 | 0.95 | - | 0.3 | 7000 | [127] |
NZSP/PEO | 0.04 | - | 4.7 | 0.2 | 300 | [136] |
PEO-PN | 0.12 | 0.61 | 5.2 | 0.1 | 1000 | [138] |
GO + PVDF-HFP | 2.3 | 0.82 | 4.7 | 5 | 400 | [140] |
AlF3/NASICON | 0.024 | - | - | 0.15 0.25 | 150 150 | [143] |
BTO/NZSP | 0.96 | - | 4.4 | 0.1 0.2 0.3 | 1000 1000 1000 | [144] |
NBO/NASICON | 1 | - | 6 | 0.3 | 600 | [145] |
Na3Zr2Si2PO12 | 0.85 | - | 4.3 | 0.1 | 1000 | [146] |
SnOx/Sn + NASICON | 0.59 | - | 3 | 0.1 | 1500 | [147] |
NaPTAB + PVDF-HFP | 0.94 | 0.91 | 5.2 | 0.05 | 100 | [148] |
NaTFSI/PEO(80 oC) | 0.41 | 0.16 | 4.66 | 0.1 | 1000 | [149] |
octa-POSS/PEG/NaClO4 | 0.45 | 0.23 | 4.5 | 0.1 0.5 | 5100 3550 | [150] |
NZSP/PVDF-HFP | 0.1 | 0.63 | 4.4 | 0.2 | 400 | [151] |
NaClO4/PEGDA/PEG | 0.08 | 0.83 | 4.8 | - | - | [152] |
EPTA | 5.33 | 0.42 | 5.5 | 1 | 800 | [153] |
PEO/PMMA/PVDF-HFP | 2.02 | - | 4.9 | 0.25 | 600 | [154] |
In conclusion, we have discussed each of types of electrolytes above. The ideal electrolyte should have high ionic conductance, high interfacial stability, wide electrochemical window, thermal stability, low cost, and long life. However, different electrolytes show diverse properties. Liquid electrolyte additives can directly or indirectly participate in the construction of SEI layer to effectively inhibit the growth of sodium dendrite, but different kinds of additives may cause the battery internal reaction to be too complex, which is not conducive to the study of the electrode surface reaction; High-concentration electrolyte is an improvement of liquid electrolyte, which can solve the problem of sodium dendrite and prevent the excessive reaction of metal sodium and electrolyte, but the ion transmission speed and cost caused by high-concentration electrolyte density are important reasons for the difficulty of its commercialization; Novel electrolytes provide infinite possibilities for the development of high-performance batteries. The solid-state electrolyte is the hotspot and focus of research; Polymer electrolytes can provide excellent flexibility thus forming an acceptable contact with the anode; Inorganic solid-state electrolytes can confine the growth of dendrite by strategies such as doping; Hybrid solidstate electrolytes can effectively use the properties of the first two. Nevertheless, whatever the type of the electrolyte is, interfacial chemistry between SMAs and electrolytes plays a vital role in regulating the Na plating/stripping behavior and improving the cycling performance of SMBs. This shows that there is still a lot of space for the progress of SMBs.
CONCLUSIONS AND OUTLOOK
Sodium batteries, as an important supplement to LIBs, can effectively alleviate the dilemma of energy storage, and SMBs receive more attention because of their high energy density. Nevertheless, the safety problems of dendrite growth seriously hinder the development of sodium batteries. We provide a fundamental discussion on sodium dendrite and then summarize the study advances on suppressed dendrite from the perspective of electrolytes and related SEI, including the component and the concentration of liquid electrolytes, solid-state electrolytes, and gel electrolytes. The related issues of SMAs and the strategies regulated by electrolytes and SEI are summarized in Figure 9. This provides a direction for us to inhibit dendrite growth through electrolytes regulation and SEI strategies in the future.
At present, despite great efforts implemented by researchers to surmount SMA issues, there are still a lot of unknown things about Na anode interface chemistry, including the Na ion nucleation mechanism on electrode surface, growth behavior of Na, the Na stripping mechanism, the dynamic evolution mechanism of SEI, the interaction mechanism between SEI and Na anode, the physical and chemical properties of SEI, and the state of SEI in the liquid environment. Thus, how to thoroughly characterize the existing crucial issues is an urgent challenge to be solved in future research.
Insight into the mechanism of dendrite formation. As a world-class problem in LMBs, dendrite has perplexed many researchers, as there does not exist a perfect description of its growth. Nevertheless, for SMBs, there is not even a proposed model at present. Therefore, the formation model of sodium dendrite is urgent to put forward, which lies in more advanced characterization techniques to reveal the in-depth mechanism.
Exploring advanced electrolyte additives. Electrolyte additives can optimize the physical structure and chemical composition of SEI, facilitate the even deposition of sodium ions, and realize the dendrite-free metallic sodium anode.
Developing novel functional electrolytes. For liquid electrolytes, novel functional salts and solvents need to be explored and designed, with a focus on enhancing CE and settling safety issues. In terms of solid electrolytes, new synthesis methods and preparation technologies need to be explored to realize high ionic conductivity and a broad electrochemical window of electrolytes.
Delving into the influence of SEI on dendrite. Currently, most research on SEI focuses on the battery performance. However, the overall dendrite suppression is inseparable from the construction of SEI. Hence, the study of the inhibitory effect of SEI on dendrite should be given great importance, e.g. the impact of different chemical components in SEI and the influence of the balance between thickness and uniformity on dendrite.
Clarify the relationship among electrolyte, SEI, and dendrite. The structural characteristics of electrolyte, SEI, and dendrite need to be studied by advanced characterization methods, and the relationship among them should be elucidated. This provides a more effective way to achieve a dendrite-free sodium anode through electrolyte regulation and SEI strategies in the future.
Emerging computer-aided methods. The design and development of electrolytes and the influencing factors of SEI components on dendrites are very complex. It is a rather time-consuming and costly process to explore through experiments. As a result, mechanism exploration can be combined with emerging computational science such as machine learning and large data analysis to predict the possible mechanism for sodium dendrite and SEI, thus saving labor cost and contributing to technological development.
Combining advanced characterization with theoretical simulations. Advanced characterization techniques should be combined with theoretical simulation to explore Na metal deposition/stripping behavior and the mechanism of SEI formation.
DECLARATIONS
Authors’ contributionsPrepared and revised the manuscript: Li G, Lou X
Revised the manuscript: Peng C, Liu C
Designed and revised the manuscript: Chen W
All authors contributed to the discussion and preparation of the manuscript.
Availability of data and materialsNot applicable.
Financial support and sponsorshipThis review was supported by the National Natural Science Foundation of China (22279121, U1804129), China Postdoctoral Science Foundation (Grant No. 2022M712863), Basic Discipline Top Student Training Program 2.0 of Ministry of Education, Zhongyuan Youth Talent Support Program of Henan Province, Joint Fund of Scientific and Technological Research and Development Program of Henan Province, and Zhengzhou University.
Conflicts of interestAll authors declared that there are no conflicts of interest.
Ethical approval and consent to participateNot applicable.
Consent for publicationNot applicable.
Copyright© The Author(s) 2022.
REFERENCES
1. Ge J, Fan L, Rao AM, Zhou J, Lu B. Surface-substituted Prussian blue analogue cathode for sustainable potassium-ion batteries. Nat Sustain 2022;5:225-34.
2. Song K, Liu C, Mi L, Chou S, Chen W, Shen C. Recent progress on the alloy-based anode for sodium-ion batteries and potassium-ion batteries. Small 2021;17:e1903194.
3. Wan Y, Song K, Chen W, et al. Ultra-high initial coulombic efficiency induced by interface engineering enables rapid, stable sodium storage. Angew Chem Int Ed Engl 2021;60:11481-6.
4. Leng K, Li G, Guo J, et al. A safe polyzwitterionic hydrogel electrolyte for long-life quasi-solid state zinc metal batteries. Adv Funct Mater 2020;30:2001317.
5. Zhang C, Liu S, Li G, Zhang C, Liu X, Luo J. Incorporating ionic paths into 3D conducting scaffolds for high volumetric and areal capacity, high rate lithium-metal anodes. Adv Mater 2018:e1801328.
6. Li G, Guan X, Wang A, Wang C, Luo J. Cations and anions regulation through zwitterionic gel electrolytes for stable lithium metal anodes. Energy Storage Mater 2020;24:574-8.
7. Wang R, Zheng J, Feng X, et al. Highly [010]-oriented, gradient Co-doped LiMnPO4 with enhanced cycling stability as cathode for Li-ion batteries. J Solid State Electrochem 2020;24:511-9.
8. Yang K, Zhang X, Song K, et al. Se-C bond and reversible SEI in facile synthesized SnSe23D carbon induced stable anode for sodium-ion batteries. Electrochimica Acta 2020;337:135783.
9. Hwang JY, Myung ST, Sun YK. Sodium-ion batteries: present and future. Chem Soc Rev 2017;46:3529-614.
10. Åvall G, Mindemark J, Brandell D, Johansson P. Sodium-ion battery electrolytes: modeling and simulations. Adv Energy Mater 2018;8:1703036.
11. Zhang J, Song K, Mi L, et al. Bimetal synergistic effect induced high reversibility of conversion-type Ni@NiCo2S4 as a free-standing anode for sodium ion batteries. J Phys Chem Lett 2020;11:1435-42.
12. Chen L, Song K, Shi J, et al. PAANa-induced ductile SEI of bare micro-sized FeS enables high sodium-ion storage performance. Sci China Mater 2021;64:105-14.
13. Ma B, Bai P. Fast charging limits of ideally stable metal anodes in liquid electrolytes. Adv Eng Mater 2022;12:2102967.
14. Zhao Y, Liu H, Meng X, Liu A, Chen Y, Ma T. A cross-linked tin oxide/polymer composite gel electrolyte with adjustable porosity for enhanced sodium ion batteries. Chem Energ J 2022;431:133922.
15. Zhou S, Lan J, Song K, Zhang Z, Shi J, Chen W. SnS/SnS2/rGO heterostructure with fast kinetics enables compact sodium ion storage. FlatChem 2021;28:100259.
16. Miao RJ, Cao XG, Wang WG, Zhang HY. Influence of Bi2O3 additive on the electrochemical performance of Na3.1Y0.1Zr1.9Si2PO12 inorganic solid electrolyte. Ceram Int 2021;47:17455-62.
17. Das SK, Lau S, Archer LA. Sodium-oxygen batteries: a new class of metal-air batteries. J Mater Chem A 2014;2:12623.
19. Zhang D, Li B, Wang S, Yang S. Simultaneous formation of artificial SEI film and 3D host for stable metallic sodium anodes. ACS Appl Mater Interfaces 2017;9:40265-72.
20. Wu J, Liu J, Lu Z, et al. Non-flammable electrolyte for dendrite-free sodium-sulfur battery. Energy Storage Mater 2019;23:8-16.
21. Zhao S, Li L, Li F, Chou S. Recent progress on understanding and constructing reliable Na anode for aprotic Na-O2 batteries: a mini review. Electrochem commun 2020;118:106797.
22. Tong Z, Wang S, Fang M, et al. Na-CO2 battery with NASICON-structured solid-state electrolyte. Nano Energy 2021;85:105972.
23. Ding J, Zhou H, Zhang H, Tong L, Mitlin D. Selenium impregnated monolithic carbons as free-standing cathodes for high volumetric energy lithium and sodium metal batteries. Adv Energy Mater 2018;8:1701918.
24. Pham VH, Boscoboinik JA, Stacchiola DJ, et al. Selenium-sulfur (SeS) fast charging cathode for sodium and lithium metal batteries. Energy Storage Mater 2019;20:71-9.
25. Wang Y, Wang Y, Wang Y, et al. Developments and perspectives on emerging high-energy-density sodium-metal batteries. Chem 2019;5:2547-70.
26. Wang Y, Jiang R, Liu Y, et al. Enhanced sodium metal/electrolyte interface by a localized high-concentration electrolyte for sodium metal batteries: first-principles calculations and experimental studies. ACS Appl Energy Mater 2021;4:7376-84.
27. Yu Q, Lu Q, Qi X, et al. Liquid electrolyte immobilized in compact polymer matrix for stable sodium metal anodes. Energy Storage Mater 2019;23:610-6.
28. Xu X, Li Y, Cheng J, et al. Composite solid electrolyte of Na3PS4-PEO for all-solid-state SnS2/Na batteries with excellent interfacial compatibility between electrolyte and Na metal. J Energ Chem 2020;41:73-8.
29. Mittal N, Tien S, Lizundia E, Niederberger M. Hierarchical nanocellulose-based gel polymer electrolytes for stable na electrodeposition in sodium ion batteries. Small 2022:e2107183.
30. Bao C, Wang B, Liu P, et al. Solid electrolyte interphases on sodium metal anodes. Adv Funct Mater 2020;30:2004891.
31. Yao G, Zhang X, Yan Y, et al. Facile synthesis of hierarchical Na2Fe(SO4)2@rGO/C as high-voltage cathode for energy density-enhanced sodium-ion batteries. J Energ Chem 2020;50:387-94.
32. Ponrouch A, Monti D, Boschin A, Steen B, Johansson P, Palacín MR. Non-aqueous electrolytes for sodium-ion batteries. J Mater Chem A 2015;3:22-42.
33. Li Y, Arnold W, Halacoglu S, Jasinski JB, Druffel T, Wang H. Phase-transition interlayer enables high-performance solid-state sodium batteries with sulfide solid electrolyte. Adv Funct Mater 2021;31:2101636.
34. Wei S, Choudhury S, Xu J, Nath P, Tu Z, Archer LA. Highly stable sodium batteries enabled by functional ionic polymer membranes. Adv Mater 2017;29:1605512.
35. Manohar C, Raj K A, Kar M, Forsyth M, Macfarlane DR, Mitra S. Stability enhancing ionic liquid hybrid electrolyte for NVP@C cathode based sodium batteries. Sustainable Energy Fuels 2018;2:566-76.
36. Mishra K, Yadav N, Hashmi SA. Recent progress in electrode and electrolyte materials for flexible sodium-ion batteries. J Mater Chem A 2020;8:22507-43.
37. Wang T, Hua Y, Xu Z, Yu JS. Recent advanced development of artificial interphase engineering for stable sodium metal anodes. Small 2022;18:e2102250.
38. Lee J, Kim J, Kim S, Jo C, Lee J. A review on recent approaches for designing the SEI layer on sodium metal anodes. Mater Adv 2020;1:3143-66.
40. Ma C, Xu T, Wang Y. Advanced carbon nanostructures for future high performance sodium metal anodes. Energy Storage Mater 2020;25:811-26.
41. Fan L, Li X. Recent advances in effective protection of sodium metal anode. Nano Energy 2018;53:630-42.
42. Matios E, Wang H, Wang C, Li W. Enabling safe sodium metal batteries by solid electrolyte interphase engineering: a review. Ind Eng Chem Res 2019;58:9758-80.
44. Zheng X, Gu Z, Liu X, et al. Bridging the immiscibility of an all-fluoride fire extinguishant with highly-fluorinated electrolytes toward safe sodium metal batteries. Energy Environ Sci 2020;13:1788-98.
45. Zheng J, Chen S, Zhao W, Song J, Engelhard MH, Zhang J. Extremely stable sodium metal batteries enabled by localized high-concentration electrolytes. ACS Energy Lett 2018;3:315-21.
46. Zhu M, Li L, Zhang Y, et al. An in-situ formed stable interface layer for high-performance sodium metal anode in a non-flammable electrolyte. Energy Storage Mater 2021;42:145-53.
47. Lei D, He YB, Huang H, et al. Cross-linked beta alumina nanowires with compact gel polymer electrolyte coating for ultra-stable sodium metal battery. Nat Commun 2019;10:4244.
48. Park T, Park M, Ban A, Lee Y, Kim D. Nonflammable gel polymer electrolyte with ion-conductive polyester networks for sodium metal cells with excellent cycling stability and enhanced safety. ACS Appl Energy Mater 2021;4:10153-62.
49. Yao Y, Wei Z, Wang H, et al. Toward high energy density all solid-state sodium batteries with excellent flexibility. Adv Energy Mater 2020;10:1903698.
50. Lai H, Li Y, Wang J, Li W, Wu X, Wen Z. Design of solid-state sodium-ion batteries with high mass-loading cathode by porous-dense bilayer electrolyte. Journal of Materiomics 2021;7:1352-7.
51. Ran L, Li M, Cooper E, et al. Enhanced safety and performance of high-voltage solid-state sodium battery through trilayer, multifunctional electrolyte design. Energy Storage Mater 2021;41:8-13.
52. Zhang Z, Zhang Q, Ren C, et al. A ceramic/polymer composite solid electrolyte for sodium batteries. J Mater Chem A 2016;4:15823-8.
53. Hong Y, Li N, Chen H, Wang P, Song W, Fang D. In operando observation of chemical and mechanical stability of Li and Na dendrites under quasi-zero electrochemical field. Energy Storage Mater 2018;11:118-26.
54. Han M, Zhu C, Ma T, Pan Z, Tao Z, Chen J. In situ atomic force microscopy study of nano-micro sodium deposition in ester-based electrolytes. Chem Commun (Camb) 2018;54:2381-4.
55. Wang H, Wang C, Matios E, Li W. Facile stabilization of the sodium metal anode with additives: unexpected key role of sodium polysulfide and adverse effect of sodium nitrate. Angew Chem Int Ed Engl 2018;57:7734-7.
56. Hu J, Wang H, Wang S, et al. Electrochemical deposition mechanism of sodium and potassium. Energy Storage Mater 2021;36:91-8.
57. Zheng X, Bommier C, Luo W, Jiang L, Hao Y, Huang Y. Sodium metal anodes for room-temperature sodium-ion batteries: applications, challenges and solutions. Energy Storage Mater 2019;16:6-23.
58. Gaissmaier D, van den Borg M, Fantauzzi D, Jacob T. Microscopic properties of Na and Li-a first principle study of metal battery anode materials. ChemSusChem 2020;13:771-83.
59. Medenbach L, Bender CL, Haas R, et al. Origins of dendrite formation in sodium-oxygen batteries and possible countermeasures. Energy Technol 2017;5:2265-74.
60. Rodriguez R, Loeffler KE, Nathan SS, et al. In situ optical imaging of sodium electrodeposition: effects of fluoroethylene carbonate. ACS Energy Lett 2017;2:2051-7.
61. Akolkar R. Mathematical model of the dendritic growth during lithium electrodeposition. J Power Sources 2013;232:23-8.
62. Park MS, Woo HS, Heo JM, et al. Thermoplastic polyurethane elastomer-based gel polymer electrolytes for sodium-metal cells with enhanced cycling performance. ChemSusChem 2019;12:4645-54.
63. Gu Y, Wang WW, Li YJ, et al. Designable ultra-smooth ultra-thin solid-electrolyte interphases of three alkali metal anodes. Nat Commun 2018;9:1339.
64. Sun B, Xiong P, Maitra U, et al. Design strategies to enable the efficient use of sodium metal anodes in high-energy batteries. Adv Mater 2020;32:e1903891.
65. Wang X, Wang X, Chen J, Zhao Y, Mao Z, Wang D. Durable sodium battery composed of conductive Ti3C2Tx MXene modified gel polymer electrolyte. Solid State Ionics 2021;365:115655.
66. Gao H, Xin S, Xue L, Goodenough JB. Stabilizing a high-energy-density rechargeable sodium battery with a solid electrolyte. Chem 2018;4:833-44.
67. Wang H, Matios E, Luo J, Li W. Combining theories and experiments to understand the sodium nucleation behavior towards safe sodium metal batteries. Chem Soc Rev 2020;49:3783-805.
68. Rees GJ, Spencer Jolly D, Ning Z, Marrow TJ, Pavlovskaya GE, Bruce PG. Imaging sodium dendrite growth in all-solid-state sodium batteries using 23Na T2-weighted magnetic resonance imaging. Angew Chem Int Ed Engl 2021;60:2110-5.
69. Wang X, Chen J, Mao Z, Wang D. In situ construction of a stable interface induced by the SnS2 ultra-thin layer for dendrite restriction in a solid-state sodium metal battery. J Mater Chem A 2021;9:16039-45.
70. Yi Q, Lu Y, Sun X, Zhang H, Yu H, Sun C. Fluorinated ether based electrolyte enabling sodium-metal batteries with exceptional cycling stability. ACS Appl Mater Interfaces 2019;11:46965-72.
71. Wang T, Yang K, Shi J, et al. Simple synthesis of sandwich-like SnSe2/rGO as high initial coulombic efficiency and high stability anode for sodium-ion batteries. J Energ Chem 2020;46:71-7.
72. Gao L, Chen J, Liu Y, Yamauchi Y, Huang Z, Kong X. Revealing the chemistry of an anode-passivating electrolyte salt for high rate and stable sodium metal batteries. J Mater Chem A 2018;6:12012-7.
73. Xu Y, Sun H, Ma C, Gai J, Wan Y, Chen W. Pre-sodiation strategy for superior sodium storage batteries. Chin J Chem Eng 2021;39:261-8.
74. Fang W, Jiang H, Zheng Y, et al. A bilayer interface formed in high concentration electrolyte with SbF3 additive for long-cycle and high-rate sodium metal battery. J Power Sources 2020;455:227956.
75. Lei Y, Du G, Qi Y, Niu Y, Bao S, Xu M. Gelation of organic liquid electrolyte to achieve superior sodium-ion full-cells. J Colloid Interface Sci 2021;599:190-7.
76. Xu M, Li Y, Ihsan-ul-haq M, et al. NaF-rich solid electrolyte interphase for dendrite-free sodium metal batteries. Energy Storage Mater 2022;44:477-86.
77. Wenzel S, Leichtweiss T, Krüger D, Sann J, Janek J. Interphase formation on lithium solid electrolytes - an in situ approach to study interfacial reactions by photoelectron spectroscopy. Solid State Ionics 2015;278:98-105.
78. Binder M, Mandl M, Zaubitzer S, et al. Sodium cyclopentadienide as a new type of electrolyte for sodium batteries. ChemElectroChem 2021;8:365-9.
79. Ge C, Wang L, Xue L, et al. Synthesis of novel organic-ligand-doped sodium bis(oxalate)-borate complexes with tailored thermal stability and enhanced ion conductivity for sodium ion batteries. J Power Sources 2014;248:77-82.
80. Voropaeva D, Novikova S, Kulova T, Yaroslavtsev A. Solvation and sodium conductivity of nonaqueous polymer electrolytes based on Nafion-117 membranes and polar aprotic solvents. Solid State Ionics 2018;324:28-32.
81. Xu X, Zhou D, Qin X, et al. A room-temperature sodium-sulfur battery with high capacity and stable cycling performance. Nat Commun 2018;9:3870.
82. Li P, Jiang Z, Huang X, Lu X, Xie J, Cheng S. Nitrofullerene as an electrolyte-compatible additive for high-performance sodium metal batteries. Nano Energy 2021;89:106396.
83. Chen Q, He H, Hou Z, et al. Building an artificial solid electrolyte interphase with high-uniformity and fast ion diffusion for ultralong-life sodium metal anodes. J Mater Chem A 2020;8:16232-7.
84. Chen X, Shen X, Hou T, Zhang R, Peng H, Zhang Q. Ion-solvent chemistry-inspired cation-additive strategy to stabilize electrolytes for sodium-metal batteries. Chem 2020;6:2242-56.
85. Hu Y, Lu Y. The mystery of electrolyte concentration: from superhigh to ultralow. ACS Energy Lett 2020;5:3633-6.
86. Ma J, Zhang W, Wang X, et al. Revealing the mechanism of saturated ether electrolyte for improving the long-cycling stability of Na-O2 batteries. Nano Energy 2021;84:105927.
87. Wang H, Tong Z, Yang R, et al. Electrochemically stable sodium metal-tellurium/carbon nanorods batteries. Adv Energy Mater 2019;9:1903046.
88. Zhou X, Zhang Q, Zhu Z, Cai Y, Li H, Li F. Anion-reinforced solvation for a gradient inorganic-rich interphase enables high-rate and stable sodium batteries. Angew Chem Int Ed Engl 2022;61:e202205045.
89. Forsyth M, Yoon H, Chen F, et al. Novel Na+ ion diffusion mechanism in mixed organic-inorganic ionic liquid electrolyte leading to high Na+ transference number and stable, high rate electrochemical cycling of sodium cells. J Phys Chem C 2016;120:4276-86.
90. Sun H, Zhu G, Xu X, et al. A safe and non-flammable sodium metal battery based on an ionic liquid electrolyte. Nat Commun 2019;10:3302.
91. Wang D, Hwang J, Chen C, Kubota K, Matsumoto K, Hagiwara R. A β”-Alumina/inorganic ionic liquid dual electrolyte for intermediate-temperature sodium-sulfur batteries. Adv Funct Materials 2021;31:2105524.
92. Ruiz-martínez D, Kovacs A, Gómez R. Development of novel inorganic electrolytes for room temperature rechargeable sodium metal batteries. Energy Environ Sci 2017;10:1936-41.
93. Zhou H, Li H, Gong Q, et al. A sodium liquid metal battery based on the multi-cationic electrolyte for grid energy storage. Energy Storage Mater 2022;50:572-9.
94. Liu X, Zheng X, Deng Y, et al. Implanting a fire-extinguishing alkyl in sodium metal battery electrolytes via a functional molecule. Adv Funct Materials 2022;32:2109378.
95. Jiang R, Hong L, Liu Y, et al. An acetamide additive stabilizing ultra-low concentration electrolyte for long-cycling and high-rate sodium metal battery. Energy Storage Mater 2021;42:370-9.
96. Shi Q, Zhong Y, Wu M, Wang H, Wang H. High-performance sodium metal anodes enabled by a bifunctional potassium salt. Angew Chem Int Ed Engl 2018;57:9069-72.
97. Lee J, Lee Y, Lee J, et al. Ultraconcentrated sodium bis(fluorosulfonyl)imide-based electrolytes for high-performance sodium metal batteries. ACS Appl Mater Interfaces 2017;9:3723-32.
98. Seh ZW, Sun J, Sun Y, Cui Y. A Highly reversible room-temperature sodium metal anode. ACS Cent Sci 2015;1:449-55.
99. Wang S, Chen Y, Jie Y, et al. Stable sodium metal batteries via manipulation of electrolyte solvation structure. Small Methods 2020;4:1900856.
100. Zhang Q, Lu Y, Miao L, et al. An alternative to lithium metal anodes: non-dendritic and highly reversible sodium metal anodes for Li-Na hybrid batteries. Angew Chem Int Ed Engl 2018;57:14796-800.
101. Lee B, Paek E, Mitlin D, Lee SW. Sodium metal anodes: emerging solutions to dendrite growth. Chem Rev 2019;119:5416-60.
102. He J, Bhargav A, Shin W, Manthiram A. Stable dendrite-free sodium-sulfur batteries enabled by a localized high-concentration electrolyte. J Am Chem Soc 2021;143:20241-8.
103. Chen F, Wang X, Armand M, Forsyth M. Cationic polymer-in-salt electrolytes for fast metal ion conduction and solid-state battery applications. Nat Mater 2022; doi: 10.1038/s41563-022-01319-w.
104. Lu Z, Yang H, Guo Y, et al. Electrolyte sieving chemistry in suppressing gas evolution of sodium-metal batteries. Angew Chem Int Ed Engl 2022;61:e202206340.
105. Wang X, Zhang C, Sawczyk M, et al. Ultra-stable all-solid-state sodium metal batteries enabled by perfluoropolyether-based electrolytes. Nat Mater 2022;21:1057-65.
106. Zhu X, Zhao R, Deng W, Ai X, Yang H, Cao Y. An all-solid-state and all-organic sodium-ion battery based on redox-active polymers and plastic crystal electrolyte. Electrochimica Acta 2015;178:55-9.
107. Babu B, Enke M, Prykhodska S, Lex-Balducci A, Schubert US, Balducci A. New diglyme-based gel polymer electrolytes for na-based energy storage devices. ChemSusChem 2021;14:4836-45.
108. Yu X, Xue L, Goodenough JB, Manthiram A. Ambient-temperature all-solid-state sodium batteries with a laminated composite electrolyte. Adv Funct Mater 2021;31:2002144.
109. Zheng J, Yang Y, Li W, Feng X, Chen W, Zhao Y. Novel flame retardant rigid spirocyclic biphosphate based copolymer gel electrolytes for sodium ion batteries with excellent high-temperature performance. J Mater Chem A 2020;8:22962-8.
110. Bitner-Michalska A, Nolis GM, Żukowska G, et al. Fluorine-free electrolytes for all-solid sodium-ion batteries based on percyano-substituted organic salts. Sci Rep 2017;7:40036.
111. Gao H, Xue L, Xin S, Park K, Goodenough JB. A plastic-crystal electrolyte interphase for all-solid-state sodium batteries. Angew Chem Int Ed Engl 2017;56:5541-5.
112. Makhlooghiazad F, Nti F, Sun J, et al. Composite electrolytes based on electrospun PVDF and ionic plastic crystal matrices for Na-metal battery applications. J Phys Mater 2021;4:034003.
113. Chen G, Ye L, Zhang K, et al. Hyperbranched polyether boosting ionic conductivity of polymer electrolytes for all-solid-state sodium ion batteries. Chem Eng J 2020;394:124885.
114. Du G, Tao M, Li J, et al. Low-operating temperature, high-rate and durable solid-state sodium-ion battery based on polymer electrolyte and prussian blue cathode. Adv Energy Mater 2020;10:1903351.
115. Zhao C, Liu L, Lu Y, Wagemaker M, Chen L, Hu YS. Revealing an interconnected interfacial layer in solid-state polymer sodium batteries. Angew Chem Int Ed Engl 2019;58:17026-32.
116. Chen S, Feng F, Yin Y, Che H, Liao X, Ma Z. A solid polymer electrolyte based on star-like hyperbranched β-cyclodextrin for all-solid-state sodium batteries. J Power Sources 2018;399:363-71.
117. Gao H, Zhou W, Park K, Goodenough JB. A sodium-ion battery with a low-cost cross-linked gel-polymer electrolyte. Adv Energy Mater 2016;6:1600467.
118. Xiong W, Tu Z, Yin Z, Zhang X, Hu X, Wu Y. Supported ionic liquid gel membranes enhanced by ionization modification for sodium metal batteries. ACS Sustainable Chem Eng 2021;9:12100-8.
119. Wen P, Lu P, Shi X, et al. Photopolymerized gel electrolyte with unprecedented room-temperature ionic conductivity for high-energy-density solid-state sodium metal batteries. Adv Energy Mater 2021;11:2002930.
120. Zhang W, Zhang J, Liu X, et al. In-situ polymerized gel polymer electrolytes with high room-temperature ionic conductivity and regulated Na+ solvation structure for sodium metal batteries. Adv Funct Materials 2022;32:2201205.
121. Zheng J, Sun Y, Li W, Feng X, Chen W, Zhao Y. Effects of comonomers on the performance of stable phosphonate-based gel terpolymer electrolytes for sodium-ion batteries with ultralong cycling stability. ACS Appl Mater Interfaces 2021;13:25024-35.
122. Bay M, Wang M, Grissa R, Heinz MVF, Sakamoto J, Battaglia C. Sodium plating from Na-β”-alumina ceramics at room temperature, paving the way for fast-charging all-solid-state batteries. Adv Energy Mater 2020;10:1902899.
123. Wu J, Zhang R, Fu Q, et al. Inorganic solid electrolytes for all-solid-state sodium batteries: fundamentals and strategies for battery optimization. Adv Funct Mater 2021;31:2008165.
124. Matios E, Wang H, Wang C, et al. Graphene regulated ceramic electrolyte for solid-state sodium metal battery with superior electrochemical stability. ACS Appl Mater Interfaces 2019;11:5064-72.
125. Weng W, Liu G, Shen L, Yao X. High ionic conductivity and stable phase Na11.5Sn2Sb0.5Ti0.5S12 for all-solid-state sodium batteries. J Power Sources 2021;512:230485.
126. Wang X, Chen J, Wang D, Mao Z. Improving the alkali metal electrode/inorganic solid electrolyte contact via room-temperature ultrasound solid welding. Nat Commun 2021;12:7109.
127. Wang C, Sun Z, Zhao Y, et al. Grain boundary design of solid electrolyte actualizing stable all-solid-state sodium batteries. Small 2021;17:e2103819.
128. Quérel E, Seymour ID, Cavallaro A, Ma Q, Tietz F, Aguadero A. The role of NaSICON surface chemistry in stabilizing fast-charging Na metal solid-state batteries. J Phys Energy 2021;3:044007.
129. Zhang S, Zhao Y, Zhao F, et al. Gradiently sodiated alucone as an interfacial stabilizing strategy for solid-state na metal batteries. Adv Funct Mater 2020;30:2001118.
130. Johari NSM, Jonderian A, Jia S, et al. High-throughput development of Na2ZnSiO4-based hybrid electrolytes for sodium-ion batteries. J Power Sources 2022;541:231706.
131. Nsm J, Sbrs A, Ahmad N. Sodium-ion nanoionic hybrid solid electrolyte: extended study on enhanced electrical and electrochemical properties. Solid State Ionics 2022;377:115882.
132. Zhang T, Li J, Li X, et al. A silica-reinforced composite electrolyte with greatly enhanced interfacial lithium-ion transfer kinetics for high-performance lithium metal batteries. Adv Mater 2022:e2205575.
133. Zhang C, Wang A, Zhang J, Guan X, Tang W, Luo J. 2D materials for lithium/sodium metal anodes. Adv Energy Mater 2018;8:1802833.
134. Tang W, Tang S, Zhang C, et al. Simultaneously enhancing the thermal stability, mechanical modulus, and electrochemical performance of solid polymer electrolytes by incorporating 2D sheets. Adv Energy Mater 2018;8:1800866.
135. He F, Tang W, Zhang X, Deng L, Luo J. High energy density solid state lithium metal batteries enabled by sub-5 µm solid polymer electrolytes. Adv Mater 2021;33:e2105329.
136. Niu W, Chen L, Liu Y, Fan L. All-solid-state sodium batteries enabled by flexible composite electrolytes and plastic-crystal interphase. Chem Eng J 2020;384:123233.
137. Zhou Y, Xiao Z, Han D, et al. Approaching practically accessible and environmentally adaptive sodium metal batteries with high loading cathodes through in situ interlock interface. Adv Funct Materials 2022;32:2111314.
138. Ran L, Tao S, Gentle I, et al. Stable interfaces in a sodium metal-free, solid-state sodium-ion battery with gradient composite electrolyte. ACS Appl Mater Interfaces 2021;13:39355-62.
139. Matios E, Wang H, Luo J, et al. Reactivity-guided formulation of composite solid polymer electrolytes for superior sodium metal batteries. J Mater Chem A 2021;9:18632-43.
140. Luo C, Shen T, Ji H, et al. Mechanically robust gel polymer electrolyte for an ultrastable sodium metal battery. Small 2020;16:e1906208.
141. Zhang Z, Huang Y, Li C, Li X. Metal-organic framework-supported poly(ethylene oxide) composite gel polymer electrolytes for high-performance lithium/sodium metal batteries. ACS Appl Mater Interfaces 2021;13:37262-72.
142. Hamisu A, Çelik SÜ. Polymer composite electrolyte of SPSU(Na)/PPEGMA/hBN for sodium-ion batteries. Polymers and Polymer Composites 2019;27:419-28.
143. Miao X, Di H, Ge X, et al. AlF3-modified anode-electrolyte interface for effective Na dendrites restriction in NASICON-based solid-state electrolyte. Energy Storage Mater 2020;30:170-8.
144. Sun Z, Zhao Y, Ni Q, et al. Solid-state Na metal batteries with superior cycling stability enabled by ferroelectric enhanced Na/Na3Zr2Si2PO12 interface. Small 2022;18:e2200716.
145. Zhao Y, Wang C, Dai Y, Jin H. Homogeneous Na+ transfer dynamic at Na/Na3Zr2Si2PO12 interface for all solid-state sodium metal batteries. Nano Energy 2021;88:106293.
146. Wang C, Jin H, Zhao Y. Surface potential regulation realizing stable sodium/Na3Zr2Si2PO12 interface for room-temperature sodium metal batteries. Small 2021;17:e2100974.
147. Yang J, Xu H, Wu J, et al. Improving Na/Na3Zr2Si2PO12 interface via SnOx/Sn film for high-performance solid-state sodium metal batteries. Small Methods 2021;5:e2100339.
148. Yang L, Jiang Y, Liang X, et al. Novel sodium-poly(tartaric acid)borate-based single-ion conducting polymer electrolyte for sodium-metal batteries. ACS Appl Energy Mater 2020;3:10053-60.
149. Qi X, Ma Q, Liu L, et al. Sodium bis(fluorosulfonyl)imide/poly(ethylene oxide) polymer electrolytes for sodium-ion batteries. ChemElectroChem 2016;3:1741-5.
150. Zheng Y, Pan Q, Clites M, Byles BW, Pomerantseva E, Li CY. High-capacity all-solid-state sodium metal battery with hybrid polymer electrolytes. Adv Energy Mater 2018;8:1801885.
151. Ling W, Fu N, Yue J, et al. A flexible solid electrolyte with multilayer structure for sodium metal batteries. Adv Energy Mater 2020;10:1903966.
152. Luo C, Li Q, Shen D, Zheng R, Huang D, Chen Y. Enhanced interfacial kinetics and fast Na+ conduction of hybrid solid polymer electrolytes for all-solid-state batteries. Energy Storage Mater 2021;43:463-70.
153. Xu X, Lin K, Zhou D, et al. Quasi-solid-state dual-ion sodium metal batteries for low-cost energy storage. Chem 2020;6:902-18.
Cite This Article
How to Cite
Li, G.; Lou X.; Peng C.; Liu C.; Chen W. Interface chemistry for sodium metal anodes/batteries: a review. Chem. Synth. 2022, 2, 16. http://dx.doi.org/10.20517/cs.2022.19
Download Citation
Export Citation File:
Type of Import
Tips on Downloading Citation
Citation Manager File Format
Type of Import
Direct Import: When the Direct Import option is selected (the default state), a dialogue box will give you the option to Save or Open the downloaded citation data. Choosing Open will either launch your citation manager or give you a choice of applications with which to use the metadata. The Save option saves the file locally for later use.
Indirect Import: When the Indirect Import option is selected, the metadata is displayed and may be copied and pasted as needed.
About This Article
Copyright
Author Biographies
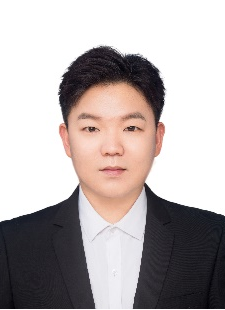
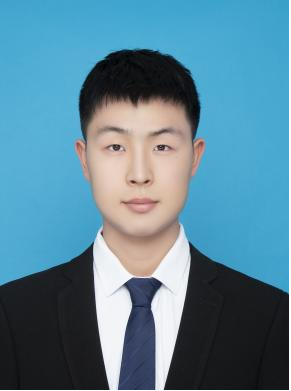
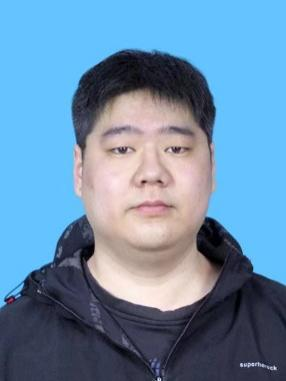
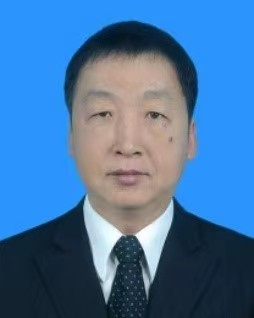
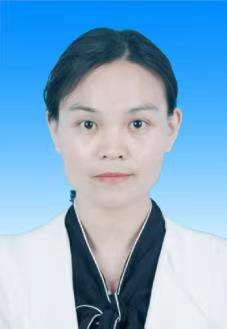
Comments
Comments must be written in English. Spam, offensive content, impersonation, and private information will not be permitted. If any comment is reported and identified as inappropriate content by OAE staff, the comment will be removed without notice. If you have any queries or need any help, please contact us at support@oaepublish.com.