Customization of functional MOFs by a modular design strategy for target applications
Abstract
Herein, we propose a versatile “functional modular assembly” strategy for customizing MOFs that allows installing the desired functional unit into a host material. The functional unit could be switched according to different applications. MOF-808, a highly stable Zr-MOF containing dangling formate groups, was selected as a host material for demonstration. Functional molecules with carboxyl connectors can be directly inserted into MOF-808 to form functional modular MOFs (FM-MOFs) through single substitution, while for those without carboxyl connectors, a pre-designed convertor was grafted firstly followed by the functional molecules in a stepwise manner. A series of tailor-made FM-MOFs were generated and show excellent performance toward different applications, such as adsorption, catalysis, fluorescent sensing, electrochemistry, and the control of surface wettability. On the other hand, the functional units on the FM-MOFs can switch freely and completely via full interconversion, as well as partly to construct multivariate MOFs (MTV-MOFs). Therefore, this strategy provides a benchmark for rapid customization of functional MOFs for diverse applications that can realize the rapid modular design of materials.
Keywords
INTRODUCTION
Porous materials have been attracting intense attention owing to their wide range of potential applications[1,2]. Rational design and functional targeting synthesis of porous materials on demand by the introduction of functional groups or molecules are essential for practical application[3,4]. It is relatively difficult to insert and modify functional groups in the structures of conventional porous solids such as zeolites and activated carbons, especially at the molecular level, whereas metal-organic frameworks (MOFs), newly developed porous crystalline materials constructed by metal clusters and organic linkers, are acknowledged to be relatively easy in this regard[5-9]. Because of their highly diversified structure, tailorable porosity, and tunable functionality, MOFs show great potential in various areas including adsorption[10-12], catalysis[13,14], chemical sensing[15,16], and electrochemistry[17,18]. Endowed with these fantastic features, MOFs are an ideal platform to customize functional materials on demand. Currently, various functional methods have been used in different kinds of MOF materials for a given system[19-21]. However, these functional approaches sometimes have poor versatility and are difficult to extend to other host MOFs or functional molecules[22]. In some cases, time- and resource-intensive processes are needed to find and design requisite host materials, functional parts, and their appropriate connection modes in each unit operation. Besides, it is also relatively difficult to impart some complex but useful functional molecules into MOF structures through direct postsynthetic substitution or sophisticated organic synthesis. Considering the variety and complexity of practical demands, a general strategy that allows the versatile and facile customization of MOFs according to a wide variety of applications is highly desirable yet remains a challenge to date.
To tackle the above-mentioned question, we here propose a “functional modular assembly” (FMA) strategy for customizing MOFs - a versatile conceptual approach that allows installing desired functional units into a host MOF material, opening up the possibility of assembling modular devices according to practical requirements. Such a novel strategy should possess the following features: (i) the functional module on the host MOF can be installed and switched freely, acting as a “plug and socket”, and thus a proper functional unit can be inserted for a targeted application; (ii) to enrich modular diversity, the accessible functional molecules should be modularized as much as possible; (iii) the assembly step of installing functional modules onto host MOF should be accomplished in a simple manner, thereby facilitating target customization of MOFs in a facile and efficient way; and (iv) the host MOF material should be stable enough to be used in different conditions. In addition, as most MOFs are moisture sensitive and unstable in aqueous media, developing stable hydrophobic MOFs is necessary for practical applications[23,24].
To fulfill this goal, the proper connection mode between host material and modules is firstly needed. Considering the reversibility of coordination bonds in MOFs, postsynthetic substitution appears to be a reliable and simple approach to implementing installing and switching functional modules in MOFs. Moreover, combining different functional modules into one host MOF through multi-substitution may also open up the possibility of constructing multivariate MOFs (MTV-MOFs) for a target application. In this work, as a proof-of-concept experiment, we demonstrate that such a “functional modular assembly” strategy can be achieved on the highly robust MOF-808. This Zr-MOF was chosen as a host material for the following two reasons: (i) exceptional water/chemical stability; and (ii) the dangling formate groups on Zr6 clusters are prone to be substituted by other ligands with carboxyl groups in a simple way, making it easy to graft functional molecules/active fragments onto the host material. These interesting features of MOF-808 stimulate us to try to use the carboxyl group as a connector to assemble functional modular MOFs (FM-MOFs). Functional molecules with a carboxyl connector can be directly inserted into MOF-808 through single substitution. For functional molecules without a connector, we insert a pre-designed convertor and modules in a stepwise manner by tandem postsynthetic modification. That is, the convertor has two different terminals that can combine modules and MOF-808, respectively. Theoretically, a wide range of accessible functional molecules could be used as modules in the assembly process, thus generating practically numerous distinct new MOFs for functional targeting customization. To prove the feasibility of our strategy, several applications from completely different fields including adsorption, catalysis, fluorescent sensing, electrochemistry, and the control of surface wettability were selected as examples. Such tailor-made FM-MOFs exhibit excellent performance toward different target applications. Moreover, the functional units on our FM-MOFs can fully interconvert via a simple solvothermal method, readily implementing the installing and switching of modules in MOFs freely. Interestingly, we also found that two functional modules can be assembled into MOF-808 through partial conversion to construct multivariate FM-MOFs. Overall, this functional modular assembly strategy enables MOFs to achieve the goals of multi-modules, multi-objectives, switchable modules, and interfunctional coordination, providing a general and simple route for the rapid targeting customization of functional MOFs on demands.
RESULTS AND DISCUSSION
Customization of FM-MOFs by single substitution
A schematic illustration of the construction of FM-MOFs is shown in Scheme 1. Theoretically, in the assembly process, various desired functional modules could be imparted into the host framework to customize FM-MOFs according to arbitrary target applications. Thus, it is imperative to ensure that the various functional molecules can be modularized as much as possible. Considering that labile formate groups on MOF-808 are easy to be substituted, a carboxyl group can serve as a connector to impart functional molecules into MOF-808. To specify this concept, several applications including Hg2+ capture, Ag+ recovery, proton conductivity, detection of nitro explosives, and the control of surface wettability were taken as examples. We subsequently used thioglycollic acid (M1, M refers to module), propiolic acid (M2), oxalic acid (M3), pyrenecarboxylic acid (M4), and perfluorooctanoic acid (M5) to assemble series of FM-
Figure 1. Customization of FM-MOFs through single substitution. (A) Schematic illustration of constructing FM-MOFs by functional modules with carboxyl connector. (B) Hg2+ adsorption kinetics of FM-MOF-1 at the initial concentration of 10 ppm. (C) Ag+ adsorption isotherm for FM-MOF-2. The inset shows the linear regression by fitting the experimental data with the Langmuir model. (D) Humidity dependence of the proton conductivities in FM-MOF-3 at room temperature. (E) The effect on the emission spectra of FM-MOF-4 dispersed in DMF upon incremental addition of a TNP solution (1 mM). The inset shows the original fluorescence and the decreased fluorescence upon the addition of TNP solution (1 mM). (F) Contact angle images of FM-MOF-5 and MOF-808.
Customization of FM-MOFs through tandem postsynthetic modification
The above proof-of-concept experiments revealed that functional molecules with carboxyl groups can be used as modules to insert into MOF-808 directly according to numerous target applications. However, to expand the scope of our strategy, some useful functional molecules without carboxyl groups should also be modularized. To solve this problem, we installed a pre-designed convertor into MOF-808 before functional modules insertion through a tandem postsynthetic modification [Figure 2]. Such convertors should have two terminals that can combine modules and the host framework, respectively. Here, the aforementioned Hg2+ capture and CO2 conversion were taken as target applications to fully illustrate this. Firstly, we tried to use thioglycollic acid (M1) to construct FM-MOF-1 for mercury adsorption. Considering a functional unit with more chelating sites is needed to achieve higher Hg2+ uptake capacity, 1,2-ethanedithiol (M6) containing higher sulfur content yet without carboxyl group compared with M1 was adopted. To impart M6 on MOF-808, we chose propenoic acid (M7) as a convertor and inserted it into the host framework firstly to construct FM-MOF-7, where the accessible vinyl groups could allow for further chemical modifications. As shown in Supplementary Figures 24-28, FM-MOF-7 was characterized by PXRD, N2 adsorption, SEM, FT-IR, and 1H NMR. FT-IR spectra reveal the appearance of a new characteristic peak at 1639 cm-1 compared with MOF-808, which can be attributed to the C=C stretching band of vinyl groups
Figure 2. Customization of FM-MOFs through tandem postsynthetic modification. (A and B) Scheme illustration of FM-MOFs and their pore structures. (C) Hg2+ adsorption isotherm of FM-MOF-6. The inset shows the linear regression by fitting the experimental data with the Langmuir model. (D) Yields of various cyclic carbonates prepared from the cycloaddition of CO2 with related epoxides catalyzed by FM-MOF-8 (red) and FM-MOF-9 (blue).
On the other hand, the cycloaddition of CO2 with epoxides into cyclic carbonates is an attractive strategy for addressing anthropogenic CO2 emission issues, and imidazole-based functional molecules are regarded as efficient catalysts for this purpose. Considering the issues of homogeneous catalysts in product purification and catalyst recycling, it is advisable to graft imidazole-based functional molecules onto host materials for use as heterogeneous catalysts. Here, we chose 1-allylimidazole (M8) as a functional module to construct FM-MOF-8. Because of the absence of carboxylic connector in M8, a suitable convertor was needed. Therefore, bromoacetic acid (M9), which contains both a carboxylic connector and an accessible bromomethyl group for further chemical modification, was selected to assemble FM-MOF-9 first. The detailed characterizations are provided in Supplementary Figures 38-42. FM-MOF-9 was then treated with 1-allylimidazole (M8) through a tandem postsynthetic modification to obtain FM-MOF-8, as characterized by PXRD, N2 adsorption, SEM, FT-IR, and NMR [Supplementary Figures 43-47]. Interestingly, the two incorporated modules of M8 and M9 in FM-MOF-8 combined together to form an imidazolium-based ionic liquid (IL) unit. Because of the proper distribution of formate groups in MOF-808, theoretically, such IL unit in FM-MOF-8 would also disperse uniformly within the pore, thus avoiding agglomeration and providing more accessible catalytic sites compared to the conventional dipping method. To evaluate the effectiveness of FM-MOF-9 as a catalyst, experiments were carried out. As shown in Figure 2D, the reaction yields catalyzed by FM-MOF-8 from related epoxides were determined to be 97.1% for epichlorohydrin, 95.2% for epibromohydrin, 86.2% for 1,2-epoxybutane, and 84.1% for allyl glycidyl ether, indicating that FM-MOF-8 exhibited a highly efficient catalytic performance for a variety of epoxides. It should be noted that the good performance of FM-MOF-8 was achieved without using any co-catalysts, whereas most reported MOF catalysts employ other substances, such as TBAB, to improve activity, which is considered uneconomical and complicated[49]. In contrast, FM-MOF-9 showed an extremely low activity, with a yield of < 10% under similar conditions, manifesting that the grafted M8 played a key role during the catalytic process. The above two proof-of-concept experiments fully demonstrated the reliability of this tandem postsynthetic modification for inserting functional modules without a carboxyl connector into MOF-808, which greatly expands the scope of FM-MOFs for functional targeting customization.
Switching modules on FM-MOFs
Another important aspect to consider is whether the functional modules on FM-MOFs could be freely switched in a simple manner, which allows for changing user-defined units in accordance with different needs, thereby affording matrix recycling and cost saving. The mutual transformation among FM-MOF-2, FM-MOF-7, and FM-MOF-9 was taken as a representative sample to confirm this [Figure 3]. These FM-MOFs were added into the solution containing the corresponding functional module, and the detailed experiments can be found in the Supplementary Materials. This conversion process was repeated several times, and the as-transformed FM-MOFs were determined by 1H NMR analysis. As shown in Supplementary Figures 48-53, for all three FM-MOFs, the signals of the original functional module disappeared, whereas the peaks attributed to the new unit emerged, demonstrating full switching of functional modules on MOF-808. Besides, PXRD measurements indicate no apparent loss of crystallinity during the conversion process [Supplementary Figures 54-59]. To further illustrate this, we also symmetrically investigated the conversion process from FM-MOF-9 to FM-MOF-7 with the increase of time. As shown in Figure 3C and D, the conservation rate reached 65% in the initial 1 h and steadily increased over time. After 24 h of reaction, the 1H NMR spectrum reveals that δ at 3.8 ppm corresponding to the hydrogen signals of -CH2- in M9 significantly decreased, whereas several new peaks around 5-7 ppm attributed to the vinyl group in M7 enhanced dramatically, achieving a high conservation of 95%. The peak of M9 further reduced when we repeated this conservation process several times, demonstrating essentially full conversion from FM-MOF-9 to FM-MOF-7.
Figure 3. Full conversion process among FM-MOFs: (A and B) scheme illustration of mutual transformation among FM-MOFs and the associated pore structures of FM-MOF-2, FM-MOF-7, and FM-MOF-9; (C) the conversion process characterized by 1H NMR spectra; and (D) the conversion rate from FM-MOF-9 to FM-MOF-7 with the increase of time.
Interestingly, we found that two functional modules could coexist in one host matrix during the switching process, which would form multivariate MOFs (MTV-MOFs) via partial conversion [Figure 4]. For full illustration, Hg2+ detection was selected as target application. We combined a mercury capture module and fluorophore moiety into MOF-808 through partial conservation and supposed that the resulting MTV-MOF would show improved performance in mercury detection. As such, the aforementioned FM-MOF-1 was immersed in DMF solution containing M4 for a period of time to obtain FM-MOF-1-4 (see Supplementary Figures 60-62). Compared to FM-MOF-1, the 1H NMR spectra of this MTV-MOF reveal that δ at 2.8 ppm corresponding to M1 decreased and new peaks assigned to the hydrogen signals of pyrene in M4 emerged [Figure 4C]. The relevant signals of M1 in FM-MOF-1 and FM-MOF-1-4 were also integrated against that of the BTC ligand, resulting in peak ratios of 6:7 and 6:2, respectively, demonstrating that 71% of M1 was substituted by M4 during module switching process [Supplementary Figures 63 and 64]. FM-MOF-1-4 was then used to sense a trace quantity of Hg2+ in aqueous solutions. As shown in Figure 4D, obvious fluorescence quenching was observed as the concentration of Hg2+ increased from 10 to 100 µM, specially, the quenching effect of Hg2+ was quantified by the Stern-Volmer equation (I0/I = 1 + Ksv[M]), where I0 and I are emission intensity of MOF material before and after reaction with Hg2+, Ksv is the Stern-Volmer quenching effect constant of Hg2+, and [M] is the concentration of Hg2+. As shown in Figure 4E, FM-MOF-1-4 showed a distinguishable Ksv value for Hg2+ at 6260 M−1, which is 2.6 times higher than that of
Figure 4. Partial conversion process among FM-MOFs: (A) scheme illustration of constructing MTV-MOF through a partial conversion process; (B) constructing FM-MOF-1-4 from FM-MOF-1; (C) 1H NMR spectra of (A) FM-MOF-1-4, (B) FM-MOF-1, and (C) FM-MOF-4 in D2O/KOH solution; (D) effect on the emission spectra of FM-MOF-1-4 with different concentration of Hg2+ in aqueous solution; and (E) Stern-Volmer plots of Hg2+ in aqueous solution for FM-MOF-1-4 (red) and FM-MOF-4 (blue).
CONCLUSION
We propose a versatile “functional modular assembly” strategy for customizing MOFs, which allows installing the desired functional unit into a host material, and the unit can be switched flexibly according to different requirements. Functional molecules with connectors could be used as modules to insert into MOF structures through single substitution directly. To enrich modular diversity, molecules without connectors could also be modularized by inserting pre-designed convertors into MOFs ahead through tandem postsynthetic modifications. Therefore, a wide range of available functional molecules, both with and without connectors, could be modularized during such an assembly process. Thus, a series of tailor-made FM-MOFs were generated and showed excellent performance toward entirely different fields, covering adsorption, catalysis, fluorescent sensing, electrochemistry, and the control of surface wettability. Moreover, the functional units on our FM-MOFs can be freely switched via full interconversion. Interestingly, we also found that two functional modules could coexist in one host matrix through partial conversion, opening up the possibility to construct multivariate MOFs (MTV-MOFs). Our strategy, therefore, provides a versatile and simple route for the rapid targeting customization of functional MOFs according to diverse applications, achieving the goals of multi-modules, multi-objectives, switchable units, and interfunctional cooperation for FM-MOFs.
DECLARATIONS
Authors’ contributions
Planned the study, analyzed the data and prepare the manuscript: Peng Y, Tan Q, Huang H
Performed manuscript correcting: Kang X, Zhu Q, Zhong C, Han B
Availability of data and materials
The experimental data and details are available from the Supplementary Materials in the journal.
Financial support and sponsorship
This work is supported by Beijing Natural Science Foundation (2222043) and National Natural Science Foundation of China (21978212).
Conflict of interest
All authors declared that there are no conflicts of interest.
Ethical approval and consent to participate
Not applicable.
Consent for publication
Not applicable.
Copyright
© The Author(s) 2022.
Supplementary Materials
REFERENCES
1. Slater AG, Cooper AI. Porous materials. Function-led design of new porous materials. Science 2015;348:aaa8075.
3. Wu J, Xu F, Li S, et al. Porous polymers as multifunctional material platforms toward task-specific applications. Adv Mater 2019;31:e1802922.
4. Siegelman RL, Kim EJ, Long JR. Porous materials for carbon dioxide separations. Nat Mater 2021;20:1060-72.
5. Kirchon A, Feng L, Drake HF, Joseph EA, Zhou HC. From fundamentals to applications: a toolbox for robust and multifunctional MOF materials. Chem Soc Rev 2018;47:8611-38.
6. Cui Y, Li B, He H, Zhou W, Chen B, Qian G. Metal-organic frameworks as platforms for functional materials. Acc Chem Res 2016;49:483-93.
7. Li B, Chrzanowski M, Zhang Y, Ma S. Applications of metal-organic frameworks featuring multi-functional sites. Coordin Chem Rev 2016;307:106-29.
8. Deng H, Doonan CJ, Furukawa H, et al. Multiple functional groups of varying ratios in metal-organic frameworks. Science 2010;327:846-50.
9. Han Y, Li JR, Xie Y, Guo G. Substitution reactions in metal-organic frameworks and metal-organic polyhedra. Chem Soc Rev 2014;43:5952-81.
10. Li J, Wang X, Zhao G, et al. Metal-organic framework-based materials: superior adsorbents for the capture of toxic and radioactive metal ions. Chem Soc Rev 2018;47:2322-56.
11. Peng Y, Huang H, Zhang Y, et al. A versatile MOF-based trap for heavy metal ion capture and dispersion. Nat Commun 2018;9:187.
12. Peng Y, Zhang Y, Tan Q, Huang H. Bioinspired construction of uranium ion trap with abundant phosphate functional groups. ACS Appl Mater Interfaces 2021;13:27049-56.
13. Shen Y, Pan T, Wang L, Ren Z, Zhang W, Huo F. Programmable logic in metal-organic frameworks for catalysis. Adv Mater 2021;33:e2007442.
14. Newar R, Akhtar N, Antil N, et al. Amino acid-functionalized metal-organic frameworks for asymmetric base-metal catalysis. Angew Chem Int Ed Engl 2021;60:10964-70.
15. Cui Y, Yue Y, Qian G, Chen B. Luminescent functional metal-organic frameworks. Chem Rev 2012;112:1126-62.
16. Wang B, Lv XL, Feng D, et al. Highly stable Zr(IV)-based metal-organic frameworks for the detection and removal of antibiotics and organic explosives in water. J Am Chem Soc 2016;138:6204-16.
17. Li H, Li C, Wang Y, et al. Selenium confined in ZIF-8 derived porous carbon@MWCNTs 3D networks: tailoring reaction kinetics for high performance lithium-selenium batteries. Chem Synth 2022;2:8.
18. Kang X, Wang B, Hu K, et al. Quantitative electro-reduction of CO2 to liquid fuel over electro-synthesized metal-organic frameworks. J Am Chem Soc 2020;142:17384-92.
19. Li N, Chang Z, Zhong M, et al. Functionalizing MOF with redox-active tetrazine moiety for improving the performance as cathode of Li-O2 batteries. CCS Chem 2021;3:1297-305.
20. Cho W, Lee HJ, Choi G, Choi S, Oh M. Dual changes in conformation and optical properties of fluorophores within a metal-organic framework during framework construction and associated sensing event. J Am Chem Soc 2014;136:12201-4.
21. Deria P, Bury W, Hupp JT, Farha OK. Versatile functionalization of the NU-1000 platform by solvent-assisted ligand incorporation. Chem Commun (Camb) 2014;50:1965-8.
22. Zhu W, Xiang G, Shang J, et al. Versatile surface functionalization of metal-organic frameworks through direct metal coordination with a phenolic lipid enables diverse applications. Adv Funct Mater 2018;28:1705274.
23. Jayaramulu K, Geyer F, Schneemann A, et al. Hydrophobic metal-organic frameworks. Adv Mater 2019;31:e1900820.
24. Mukherjee S, Datta K, Fischer RA. Hydrophobicity: a key factor en route to applications of metal-organic frameworks. Trends in Chemistry 2021;3:911-25.
25. Hou H, Yu D, Hu G. Preparation and properties of ion-imprinted hollow particles for the selective adsorption of silver ions. Langmuir 2015;31:1376-84.
26. Yao Y, Gao B, Wu F, Zhang C, Yang L. Engineered biochar from biofuel residue: characterization and its silver removal potential. ACS Appl Mater Interfaces 2015;7:10634-40.
27. Zhang M, Zhang Y, Helleur R. Selective adsorption of Ag+ by ion-imprinted O-carboxymethyl chitosan beads grafted with thiourea-glutaraldehyde. Chemical Engineering Journal 2015;264:56-65.
28. Wang L, Wang K, Huang R, Qin Z, Su Y, Tong S. Hierarchically flower-like WS2 microcrystals for capture and recovery of Au (III), Ag (I) and Pd (II). Chemosphere 2020;252:126578.
29. Pan X, Fu L, Wang H, Xue Y, Zu J. Synthesis of novel sulfydryl-functionalized chelating adsorbent and its application for selective adsorption of Ag(I) under high acid. Separation and Purification Technology 2021;271:118778.
30. Fard Z, Malliakas CD, Mertz JL, Kanatzidis MG. Direct extraction of Ag+ and Hg2+ from cyanide complexes and mode of binding by the layered K2 MgSn2S6 (KMS-2). Chem Mater 2015;27:1925-8.
31. Ma L, Wang Q, Islam SM, Liu Y, Ma S, Kanatzidis MG. Highly Selective and Efficient Removal of Heavy Metals by Layered Double Hydroxide Intercalated with the MoS4(2-) Ion. J Am Chem Soc 2016;138:2858-66.
32. Zhou Y, Gao B, Zimmerman AR, Cao X. Biochar-supported zerovalent iron reclaims silver from aqueous solution to form antimicrobial nanocomposite. Chemosphere 2014;117:801-5.
33. Asiabi H, Yamini Y, Shamsayei M, Molaei K, Shamsipur M. Functionalized layered double hydroxide with nitrogen and sulfur co-decorated carbondots for highly selective and efficient removal of soft Hg2+ and Ag+ ions. J Hazard Mater 2018;357:217-25.
34. Das R, Giri S, King Abia AL, Dhonge B, Maity A. Removal of noble metal ions (Ag + ) by mercapto group-containing polypyrrole matrix and reusability of its waste material in environmental applications. ACS Sustainable Chem Eng 2017;5:2711-24.
35. Wu H, Yang F, Lv X, et al. A stable porphyrinic metal-organic framework pore-functionalized by high-density carboxylic groups for proton conduction. J Mater Chem A 2017;5:14525-9.
36. Xue WL, Deng WH, Chen H, et al. MOF-directed synthesis of crystalline ionic liquids with enhanced proton conduction. Angew Chem Int Ed Engl 2021;60:1290-7.
37. Sharma A, Lim J, Jeong S, et al. Superprotonic conductivity of MOF-808 achieved by controlling the binding mode of grafted sulfamate. Angew Chem Int Ed Engl 2021;60:14334-8.
38. Yee KK, Reimer N, Liu J, et al. Effective mercury sorption by thiol-laced metal-organic frameworks: in strong acid and the vapor phase. J Am Chem Soc 2013;135:7795-8.
39. Hou YL, Yee KK, Wong YL, et al. Metalation triggers single crystalline order in a porous solid. J Am Chem Soc 2016;138:14852-5.
40. Luo F, Chen JL, Dang LL, et al. High-performance Hg2+ removal from ultra-low-concentration aqueous solution using both acylamide- and hydroxyl-functionalized metal-organic framework. J Mater Chem A 2015;3:9616-20.
41. Zhao M, Huang Z, Wang S, Zhang L, Zhou Y. Design of l-cysteine functionalized UiO-66 MOFs for selective adsorption of Hg(II) in aqueous medium. ACS Appl Mater Interfaces 2019;11:46973-83.
42. Shi M, Lin D, Huang R, Qi W, Su R, He Z. Construction of a mercapto-functionalized Zr-MOF/melamine sponge composite for the efficient removal of oils and heavy metal ions from water. Ind Eng Chem Res 2020;59:13220-7.
43. Liang L, Chen Q, Jiang F, et al. In situ large-scale construction of sulfur-functionalized metal-organic framework and its efficient removal of Hg(ii) from water. J Mater Chem A 2016;4:15370-4.
44. Liang L, Liu L, Jiang F, et al. Incorporation of In2S3 nanoparticles into a metal-organic framework for ultrafast removal of hg from water. Inorg Chem 2018;57:4891-7.
45. Jiang SY, He WW, Li SL, Su ZM, Lan YQ. Introduction of molecular building blocks to improve the stability of metal-organic frameworks for efficient mercury removal. Inorg Chem 2018;57:6118-23.
46. Li J, Duan Q, Wu Z, et al. Few-layered metal-organic framework nanosheets as a highly selective and efficient scavenger for heavy metal pollution treatment. Chemical Engineering Journal 2020;383:123189.
47. Mon M, Lloret F, Ferrando-soria J, Martí-gastaldo C, Armentano D, Pardo E. Selective and efficient removal of mercury from aqueous media with the highly flexible arms of a BioMOF. Angew Chem 2016;128:11333-8.
48. Fu K, Liu X, Lv C, et al. Superselective Hg(II) removal from water using a thiol-laced MOF-based sponge monolith: performance and mechanism. Environ Sci Technol 2022;56:2677-88.
Cite This Article
How to Cite
Peng, Y.; Tan Q.; Huang H.; Zhu Q.; Kang X.; Zhong C.; Han B. Customization of functional MOFs by a modular design strategy for target applications. Chem. Synth. 2022, 2, 15. http://dx.doi.org/10.20517/cs.2022.15
Download Citation
Export Citation File:
Type of Import
Tips on Downloading Citation
Citation Manager File Format
Type of Import
Direct Import: When the Direct Import option is selected (the default state), a dialogue box will give you the option to Save or Open the downloaded citation data. Choosing Open will either launch your citation manager or give you a choice of applications with which to use the metadata. The Save option saves the file locally for later use.
Indirect Import: When the Indirect Import option is selected, the metadata is displayed and may be copied and pasted as needed.
About This Article
Copyright
Author Biographies
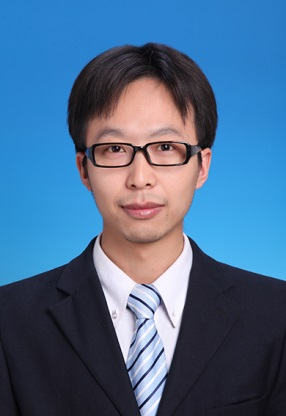
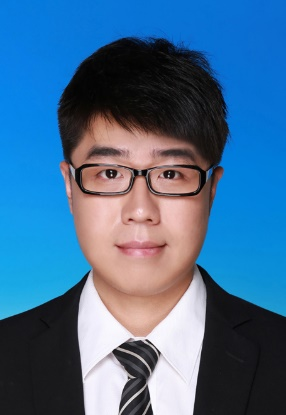
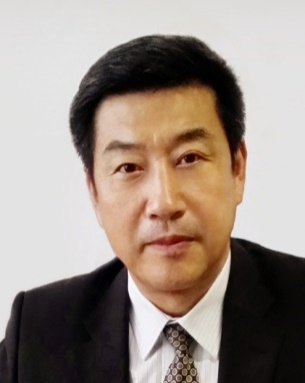
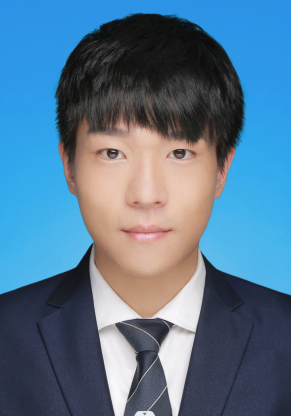
Comments
Comments must be written in English. Spam, offensive content, impersonation, and private information will not be permitted. If any comment is reported and identified as inappropriate content by OAE staff, the comment will be removed without notice. If you have any queries or need any help, please contact us at support@oaepublish.com.