Tumor microenvironment-driven resistance to immunotherapy in non-small cell lung cancer: strategies for Cold-to-Hot tumor transformation
Abstract
Non-small cell lung cancer (NSCLC) represents a formidable challenge in oncology due to its molecular heterogeneity and the dynamic suppressive nature of its tumor microenvironment (TME). Despite the transformative impact of immune checkpoint inhibitors (ICIs) on cancer therapy, the majority of NSCLC patients experience resistance, necessitating novel approaches to overcome immune evasion. This review highlights shared and subtype-specific mechanisms of immune resistance within the TME, including metabolic reprogramming, immune cell dysfunction, and physical barriers. Beyond well-characterized components such as regulatory T cells, tumor-associated macrophages, and myeloid-derived suppressor cells, emerging players - neutrophil extracellular traps, tertiary lymphoid structures, and exosomal signaling networks - underscore the TME’s complexity and adaptability. A multi-dimensional framework is proposed to transform cold, immune-excluded tumors into hot, immune-reactive ones. Key strategies include enhancing immune infiltration, modulating immunosuppressive networks, and activating dormant immune pathways. Cutting-edge technologies, such as single-cell sequencing, spatial transcriptomics, and nanomedicine, are identified as pivotal tools for decoding TME heterogeneity and personalizing therapeutic interventions. By bridging mechanistic insights with translational innovations, this review advocates for integrative approaches that combine ICIs with metabolic modulators, vascular normalizers, and emerging therapies such as STING agonists and tumor vaccines. The synergistic potential of these strategies is poised to overcome resistance and achieve durable antitumor immunity. Ultimately, this vision underscores the importance of interdisciplinary collaboration and real-time TME profiling in refining precision oncology for NSCLC, offering a blueprint for extending these advances to other malignancies.
Keywords
INTRODUCTION
Lung cancer remains the leading cause of cancer-related mortality worldwide, with non-small cell lung cancer (NSCLC) constituting approximately 85% of cases[1,2]. Immune checkpoint inhibitors (ICIs) targeting PD-1/PD-L1 have transformed NSCLC treatment, offering prolonged survival and durable responses in a subset of patients[3-6]. However, clinical success is constrained by widespread intrinsic or acquired resistance, emphasizing the urgent need to decipher the underlying mechanisms of treatment failure[7-13].
NSCLC comprises distinct molecular and histological subtypes - adenocarcinoma, squamous cell carcinoma (SCC), and large cell carcinoma (LCC) - each shaped by unique tumor microenvironment (TME) characteristics that influence immunotherapy responses[14-24]. Adenocarcinomas frequently harbor driver mutations such as EGFR, KRAS, and ALK rearrangements, which modulate immune landscapes. EGFR mutations induce immune-cold TMEs characterized by low TMB and Treg accumulation, whereas KRAS co-mutations generate divergent immune profiles, from suppressive environments (KRAS-STK11) to pro-inflammatory states (KRAS-TP53). Similarly, ALK rearrangements foster immune exclusion through reduced PD-L1 expression and impaired T-cell infiltration[25-28].
SCCs, linked to smoking-induced mutagenesis, exhibit higher TMB and neoantigen loads, theoretically enhancing immunogenicity. However, this benefit is countered by PD-L1 and CTLA-4 overexpression, establishing immunosuppressive feedback loops. Additional alterations in FGFR and DNA damage repair pathways further complicate immune responses[29-31]. LCC, though less characterized, demonstrates overlapping TME features with adenocarcinoma or SCC, ranging from immune-cold to immune-inflamed phenotypes[32].
Decoding these subtype-specific immune landscapes is crucial for refining therapeutic interventions. TME-driven resistance stems from a confluence of metabolic reprogramming, cellular exclusion, and immunosuppressive crosstalk, necessitating tailored immunotherapy strategies. Recognizing the interplay between shared immune-suppressive mechanisms and subtype-specific heterogeneity underscores the importance of integrated therapeutic approaches[33-35].
This review dissects immune evasion mechanisms within the TME and presents a multi-dimensional framework to transform cold, immune-excluded tumors into hot, immune-reactive ones. Proposed strategies encompass enhancing immune infiltration, modulating suppressive networks, reactivating dormant immune pathways, and applying emerging technologies. By bridging mechanistic insights with therapeutic innovation, we aim to advance durable antitumor immunity and expand treatment options for NSCLC patients.
SHARED MECHANISMS IN THE TME
The TME in NSCLC serves as a dynamic ecosystem where multiple suppressive mechanisms converge, fostering immune evasion and therapeutic resistance. Despite subtype-specific variations, common processes such as immune cell dysfunction, metabolic reprogramming, and structural barriers collectively drive tumor progression. These interconnected pathways underscore the TME’s critical role in shaping immune suppression, laying the groundwork for therapeutic interventions[33,35,36] [Figure 1 and Table 1].
Figure 1. TME-driven strategies for Cold-to-Hot tumor transformation in lung cancer. This figure illustrates a comprehensive framework for reprogramming the TME to overcome immunotherapy resistance in lung cancer, enabling cold-to-hot tumor transformation. The central schematic represents the lung TME, encompassing key components and their interconnections, with surrounding annotations detailing targeted therapeutic strategies. Core immune-suppressive cells, including Tregs, MDSCs, and TAMs, act as critical barriers to effective antitumor immunity. Strategies such as depleting or reprogramming these cells aim to restore immune responsiveness. Emerging players, such as NK cells, B cells, DCs, and NETs, are highlighted as potential contributors to immune activation when properly targeted. The ECM and CAFs are identified as physical and biochemical barriers that impede immune infiltration; therapeutic approaches include ECM-degrading enzymes, angiogenesis inhibitors, and CAF reprogramming therapies. Metabolic reprogramming focuses on addressing tumor-induced metabolic dysregulation, including glycolysis, lactate accumulation, and nutrient imbalances, through interventions that enhance mitochondrial function and restore metabolic homeostasis. Cellular communication within the TME, mediated by chemokines and exosomes, reinforces immunosuppressive networks; strategies such as CRISPR-Cas9, neutral sphingomyelinase blockers, and RNA-based exosome editing hold promise for disrupting these interactions. The integrative networks within the TME underscore the dynamic interplay of immune, metabolic, and structural components, necessitating combined approaches. Highlighted therapeutic strategies include immuno-metabolic reprogramming, vascular remodeling, immune co-activation, and next-generation adaptive combinations. This figure provides a unified view of the TME’s complexity and offers insights into multi-modal strategies to reprogram the TME for improved immunotherapy outcomes. TME: Tumor microenvironment; Tregs: regulatory T cells; MDSCs: myeloid-derived suppressor cells; TAMs: tumor-associated macrophages; NK cells: natural killer cells; DCs: dendritic cells; NETs: neutrophil extracellular traps; ECM: extracellular matrix; CAFs: cancer-associated fibroblasts; CRISPR-Cas9: clustered regularly interspaced short palindromic repeats-associated protein 9; TCA: tricarboxylic acid.
TME-driven mechanisms of immunotherapy resistance and cold tumor characteristics
Category | Mechanism/Characteristic | Clinical implication | References | |
Immunosuppressive cells | Treg | Tregs secrete IL-10 and TGF-β, suppressing effector T cells | Inhibits antitumor immune response | [37,46,47] |
MDSC | MDSCs deplete arginine and tryptophan via ARG1 and IDO, disrupting T cell metabolism, while ROS production impairs TCR signaling | Promotes metabolic exhaustion of T cells and increases oxidative stress, weakening antitumor immunity. | [48] | |
TAM | M2-polarized TAMs secrete VEGF and remodel ECM, forming barriers to immune infiltration | Promotes vascular abnormalities and immune exclusion, creating a pro-tumor niche resistant to immunotherapy | [49,50] | |
Emerging players | NETs | Neutrophils form NETs via NETosis, releasing DNA and enzymes that shield tumor cells and suppress immune surveillance | Induces CD8+ T cell exhaustion and supports metastases via A2AR-CCL5 and NF-κB/NLRP3 pathways | [51-53] |
TLSs | TLSs, composed of B cell follicles, T cell zones, and HEVs, recruit and activate immune cells through CXCL13 and CCL19 signaling | Organized TLSs enhance antigen presentation and boost ICI responses, while disorganized TLSs attract Tregs, promoting immune evasion | [54-56] | |
B cells | B cells and plasma cells support TLS formation and enhance immune surveillance via antigen presentation | Strengthen responses to immune checkpoint blockade in NSCLC | [55,57,58] | |
Complement system | The complement system promotes immunosuppression through elevated C5a levels, driven by circASCC3-mediated miR-432-5p sponging | Drives immune evasion and metastasis, correlating with poor immunotherapy outcomes | [59] | |
Tex | Tex suppress immune responses by losing cytotoxic functions and secreting immunosuppressive cytokines, while maintaining persistent expression of inhibitory receptors like PD-1, TIM-3, and LAG-3 | A progenitor-like Tex subset, regulated by TCF-1, retains proliferative potential and responsiveness to ICIs | [60-63] | |
NK cells | NK cells lose cytotoxicity in the tumor microenvironment due to TGF-β and lactate, which downregulate activating receptors (e.g., NKG2D) and upregulate inhibitory receptors (e.g., KIR) | Restoring NK cell function through IL-15, bispecific NK cell engagers, and lactate-targeted metabolic interventions offers new therapeutic avenues | [65,66] | |
Metabolic reprogramming | Lactate | Lactate accumulation acidifies the TME, suppresses CTL and NK cell activity, and induces M2 macrophage polarization by stabilizing HIF-1α | Acidification limits immune cell infiltration and activity, while lactate-driven VEGF and IL-10 secretion reinforces tumor growth and immune evasion | [66,68,69,70,71,72] |
Nutrient depletion | Nutrient deprivation induces M2 macrophage polarization through arginine and tryptophan depletion, stabilizing HIF-1α and enhancing VEGF and IL-10 secretion | Limits immune effector cell function, reduces the response to ICIs, and enhances resistance | [67,73,74,75,76,77,78] | |
Fatty acid | Altered fatty acid metabolism drives immune dysfunction by promoting fatty acid oxidation (FAO) in tumor cells and lipid accumulation in dendritic cells (DCs), disrupting CTL function and antigen presentation | Fatty acid metabolism reshapes the TME to impair CTL-mediated cytotoxicity and adaptive immunity, reducing the effectiveness of ICIs and supporting tumor progression | [79-82] | |
Physical and biochemical barriers | ECM | ECM remodeling by CAFs generates a dense fibrotic matrix, excluding CTLs and NK cells and disrupting immune cell migration through increased tissue stiffness | ECM remodeling promotes immune exclusion, impairs T cell and NK cell-mediated tumor killing, and reduces responsiveness to ICIs | [83,84] |
Angiogenesis | Abnormal angiogenesis driven by VEGF produces structurally defective, leaky vessels, hindering immune cell trafficking and creating a hypoxic, nutrient-deprived niche | Impaired vascular integrity promotes immune exclusion, MDSC recruitment, and TAM polarization, reducing ICI efficacy | [85-87] | |
Chemokine | Chemokine dysregulation shifts the balance toward immunosuppressive signals (e.g., CCL2, CXCL12), recruiting MDSCs, TAMs, and Tregs while reducing effector chemokines (CXCL9, CXCL10) needed for CTL infiltration | Chemokine imbalances create immune-cold tumor regions, hindering CTL recruitment and reducing ICI efficacy, contributing to immune exclusion | [88] | |
Cellular communication | Cytokine and chemokine networks | Cytokine and chemokine networks amplify immunosuppression by sustaining CAF, TAM, and Treg activity through IL-6 and TGF-β signaling while impairing CTL and NK cell functions | Reinforces immune suppression locally and systemically, reducing ICI efficacy and facilitating tumor immune evasion | [89-91] |
EVs | EVs, particularly tumor-derived exosomes, transport PD-L1 and TGF-β to inhibit TCR activation, disrupt antigen presentation, and prime pre-metastatic niches | EV-mediated immune suppression facilitates long-range immune evasion, reduces ICI efficacy, and promotes metastatic progression | [92-95] | |
Signaling pathways | Signaling pathways like cGAS-STING and RIG-I/MAVS regulate immune activation through IFN-I production, balancing pro- and antitumor immune responses. Chronic cGAS-STING activation recruits MDSCs, while RIG-I/MAVS enhances CTL function and antitumor immunity | Dysregulated signaling pathways contribute to immune suppression and tumor progression, presenting opportunities to reprogram the TME for enhanced ICI efficacy | [96-102] | |
Integrative networks | Feedback loops | Hypoxia-induced glycolysis elevates lactate production, stabilizing |
Feedback loops between metabolic reprogramming and ECM remodeling create immune-excluded zones, impairing ICI efficacy and promoting tumor progression. Targeting these loops through ECM normalization and metabolic interventions offers potential therapeutic strategies | [103-106] |
Systemic coordination | Tumor-derived exosomes carrying PD-L1 and IDO suppress TCR signaling and deplete nutrients, while the cGAS-STING pathway bridges local and systemic immunity through type I interferon responses and chronic MDSC recruitment | Systemic immunosuppression impairs ICI efficacy and promotes tumor progression. Targeting both local and systemic drivers offers the potential for reversing immune escape | [96,97,98,99,107,108] | |
Adaptive resistance | Adaptive resistance in the TME emerges through compensatory mechanisms, such as VEGF inhibition-induced TAM and MDSC recruitment or lactate targeting triggering oxidative stress, impairing CTL function | Single-target therapies often fail due to dynamic adaptations in the TME. Multi-modal strategies targeting interconnected suppressive networks are essential to sustain immune activation and improve ICI efficacy | [109-114] |
Core immune-suppressive cells in the TME
Key immunosuppressive cells - including regulatory T cells (Tregs), myeloid-derived suppressor cells (MDSCs), and tumor-associated macrophages (TAMs) - form the immunosuppressive core of the NSCLC TME by suppressing cytotoxic T lymphocytes (CTLs), dampening antigen presentation, and promoting tumor progression[37-45].
Tregs are actively recruited through tumor-derived chemokines such as CCL22 and CXCL17. Upon infiltration, they secrete suppressive cytokines, including transforming growth factor-beta (TGF-β) and IL-10, which inhibit CTL proliferation and impair dendritic cells (DCs)-mediated antigen presentation[37]. This mechanism is particularly pronounced in KRAS-mutant adenocarcinomas, where MEK-ERK-AP1 signaling fosters Treg recruitment, exacerbating immune resistance[46,47].
MDSCs, mobilized by tumor-secreted factors such as GM-CSF, VEGF, and IL-6, suppress immune responses through multiple pathways. They deplete essential metabolites like arginine and tryptophan through enzymes such as arginase 1 (ARG1) and indoleamine 2,3-dioxygenase (IDO), respectively. Additionally, they produce reactive oxygen species (ROS), which impair T cell receptor (TCR) signaling and increase oxidative stress, correlating with ICI resistance[48].
TAMs are the most abundant immune cells in the TME, predominantly adopting an M2-like immunosuppressive phenotype driven by hypoxia and cytokines such as IL-10 and TGF-β[49]. These M2-polarized TAMs promote angiogenesis via VEGF secretion, remodel the extracellular matrix (ECM), and create physical barriers that block immune cell infiltration. Hypoxia stabilizes HIF-1α, reinforcing TAM-mediated suppression through a self-sustaining feedback loop[50].
The synergistic interactions among Tregs, MDSCs, and TAMs reinforce an immunosuppressive environment, limiting the efficacy of ICIs. Understanding these cellular dynamics provides a foundation for developing targeted strategies to reinvigorate antitumor immunity.
Emerging players in the TME
Beyond well-characterized suppressive components such as Tregs, MDSCs, and TAMs, several emerging players in the TME further complicate immune resistance in NSCLC. These include neutrophil extracellular traps (NETs), tertiary lymphoid structures (TLSs), B cells, exhausted T cells (Tex), and natural killer (NK) cells. Their integration into established immunosuppressive networks underscores the need for multi-targeted therapeutic approaches.
NETs and NETosis: immune suppression and structural barriers
Neutrophils contribute to immune suppression through NETosis, releasing DNA, histones, and enzymes that form NETs. These web-like structures shield tumor cells from immune surveillance while fostering a pro-inflammatory but immunosuppressive environment[51]. NETs promote CD8+ T cell exhaustion by activating A2AR-CCL5 signaling[52], driving both local and distant metastases in an NF-κB/NLRP3-dependent manner in NSCLC[53]. Their association with poor clinical outcomes highlights NETs as potential therapeutic targets.
TLSs: immune hubs or immunosuppressive niches
TLSs are ectopic lymphoid formations composed of B cell follicles, T cell zones, and high endothelial venules (HEVs), facilitating immune cell recruitment and activation. Well-organized TLSs enhance local antigen presentation, boosting antitumor immunity and correlating with improved responses to ICIs. However, disorganized TLSs can attract suppressive immune cells such as Tregs, contributing to immune evasion. Key regulators such as CXCL13 and CCL19 modulate TLS functionality, offering therapeutic targets for TLS-driven immunomodulation[54-56].
B cells and the complement system
Recently, B cells and plasma cells, once considered minor contributors to antitumor immunity, have emerged as key players in checkpoint blockade responses in NSCLC patients[57,58]. They contribute to TLS formation, promoting immune surveillance through antigen presentation[55]. Conversely, the complement system, through elevated C5a levels driven by circASCC3-mediated miR-432-5p sponging, promotes NSCLC progression and fosters an immunosuppressive TME, underscoring its role in shaping resistance to NSCLC immunotherapy[59]. Their context-dependent roles highlight the complexity of targeting TME components in NSCLC.
Tex: reversible dysfunction
Chronic antigen stimulation, coupled with suppressive signals from Tregs, hypoxia, and metabolic stress, drives T cell exhaustion, characterized by the loss of effector functions and persistent expression of inhibitory receptors such as PD-1, TIM-3, and LAG-3[60,61]. Recent research highlights a progenitor-like Tex subset with proliferative potential and responsiveness to ICIs in NSCLC, regulated by transcription factors such as TCF-1[62,63]. Unlocking this potential through checkpoint inhibitors and epigenetic modulators remains a promising therapeutic strategy.
NK cells: restoring innate immunity
NK cells, essential for innate immunity, are often suppressed due to tumor-microenvironment-derived factors such as TGF-β and lactate. These factors downregulate activating receptors (e.g., NKG2D) while upregulating inhibitory receptors (e.g., KIR), impairing NK cell-mediated cytotoxicity[64]. Therapeutic strategies to restore NK cell function include cytokine-based therapies such as IL-15, bispecific NK cell engagers, and metabolic interventions targeting lactate accumulation[65,66].
Emerging players such as NETs, TLSs, B cells, Tex, and NK cells integrate into established immunosuppressive networks, reinforcing immune evasion through complementary and overlapping mechanisms. Their deep interconnection with traditional components underscores the necessity of multi-pronged therapeutic strategies targeting the full complexity of the TME.
Metabolic reprogramming: a hostile microenvironment
Metabolic reprogramming within the TME establishes a hostile, nutrient-deprived niche that promotes immune evasion and tumor progression. Tumor cells exploit metabolic shifts to meet their energy demands, outcompete immune cells, and create a suppressive environment[67].
Lactate accumulation: a barrier to immune activation
Driven by aerobic glycolysis (the Warburg effect), tumor cells produce excessive lactate, acidifying the TME and impairing CTLs and NK cells[66,68-70]. Lactate induces M2 polarization in TAMs, enhancing their secretion of VEGF and IL-10 while stabilizing HIF-1α to sustain angiogenesis and hypoxia[71,72]. This forms a self-reinforcing feedback loop that fortifies immune exclusion.
Nutrient depletion: immune starvation
Resource competition exacerbates immune suppression as tumor cells outcompete effector T cells for glucose, impairing their cytokine production and proliferation[67]. Enzymes like ARG1 and IDO deplete essential amino acids such as arginine and tryptophan, further restricting T cell activation[73] while fostering Treg and MDSC expansion[74-76]. Glutamine metabolism fuels anabolic processes in tumor cells, deepening resource depletion and further crippling immune responses[77,78].
Fatty acid metabolism and immune dysfunction
Altered fatty acid metabolism adds another dimension of immune dysfunction. Tumor cells rely on fatty acid oxidation (FAO) to produce lipid mediators that suppress CTL function[79,80]. Simultaneously, lipid accumulation in DCs disrupts antigen presentation, weakening adaptive immunity[81,82]. These interlinked metabolic alterations collectively promote tumor progression and immune resistance.
Metabolic reprogramming drives an interconnected web of immune evasion, where tumor-promoting pathways create a deeply suppressive ecosystem. Targeting these processes holds significant potential for therapeutic intervention in NSCLC.
Physical and biochemical barriers: excluding effector cells
Physical and biochemical barriers in the TME synergistically limit immune cell infiltration and effector function. These barriers form a dynamic network closely integrated with metabolic and cellular processes, reinforcing immune evasion and therapeutic resistance.
ECM remodeling
Cancer-associated fibroblasts (CAFs) play a central role in reshaping the ECM, generating a fibrotic scaffold that impedes immune cell infiltration. By producing dense matrix proteins such as collagen and fibronectin, CAFs create a structural barrier excluding CTLs and NK cells from the tumor core. Tissue stiffness caused by ECM remodeling further disrupts immune cell migration. In parallel, CAF-derived cytokines, notably, promote immune suppression by enhancing Treg recruitment and limiting effector cell activity[83,84].
Abnormal angiogenesis
Tumor-induced angiogenesis results in structurally defective, poorly perfused blood vessels characterized by excessive branching and leakiness. These abnormal vessels hinder immune cell trafficking and nutrient delivery, creating a hypoxic and nutrient-deprived niche. Vascular endothelial growth factor (VEGF), a key angiogenic driver, selectively recruits MDSCs while excluding CTLs[85,86]. Hypoxia-induced stabilization of hypoxia-inducible factor 1-alpha (HIF-1α) exacerbates immunosuppression by polarizing TAMs toward the pro-tumorigenic M2 phenotype[87].
Chemokine dysregulation
Chemokine imbalances further intensify immune exclusion in the TME. Tumor and stromal cells secrete immunosuppressive chemokines such as CCL2 and CXCL12, which attract MDSCs, TAMs, and Tregs while downregulating effector chemokines like CXCL9 and CXCL10 that recruit CTLs. This selective chemokine gradient creates spatially segregated immune suppression, particularly in immune-cold tumor regions resistant to ICIs[88].
By interweaving these structural and biochemical barriers, the TME constructs a formidable immune-evasive network, limiting therapeutic efficacy and underscoring the need for multifaceted strategies targeting these barriers.
Cellular communication: expanding immune regulation networks
Tumor progression and immune evasion in NSCLC are not driven by isolated molecular events but by an intricate network of cellular communication. This includes the integration of cytokines, extracellular vesicles (EVs), and intracellular signaling pathways, which collectively amplify immune suppression and sustain therapeutic resistance.
Cytokine and chemokine networks: amplifying immunosuppression
Building on chemokine-driven immune exclusion, cytokines further expand immunosuppressive networks within the TME[88]. Pro-inflammatory mediators such as IL-6 and TGF-β establish a feedback loop that sustains CAF, TAM and Treg activity while impairing CTL and NK cell functions[89-91]. These cytokines not only reinforce local immune suppression but also enable systemic immunosuppressive signaling, promoting tumor persistence despite immune surveillance. Their dynamic interplay with chemokines underscores the tightly orchestrated immune-modulating circuits that define the TME.
EVs: mediators of long-range immune evasion
EVs, particularly tumor-derived exosomes (TDEs), extend immunosuppressive signaling beyond the local TME[92]. By transporting PD-L1 and TGF-β, EVs inhibit TCR activation, disrupt antigen presentation, and establish pre-metastatic niches in distant tissues[93-95]. This vesicle-mediated crosstalk not only sustains immune evasion but also primes sites for metastatic colonization, presenting a promising target for therapeutic disruption.
Signaling pathways: orchestrating immune escape and tumor progression
Central to immune regulation are signaling pathways that modulate immune activation and suppression. The cGAS-STING pathway, for instance, mediates innate immune activation through IFN-I production upon detecting cytosolic DNA[96,97]. However, chronic activation of this pathway paradoxically recruits MDSCs and triggers immune suppression[98,99]. Similarly, RIG-I/MAVS signaling, while detecting cytosolic RNA and activating IFN-I responses[100], contributes to enhancing antitumor immunity, thereby suppressing tumor progression[101,102]. Balancing these pathways could unlock new therapeutic opportunities by shifting the TME toward an immune-reactive state.
The TME constructs a local-to-systemic immune evasion network through cytokines, exosomes, and signaling pathways, dynamically regulating immunosuppression and tumor progression while offering critical targets for multi-level therapeutic interventions.
Integrative networks in the TME
The TME operates as a dynamic and interdependent system where immune-suppressive mechanisms integrate through metabolic, structural, and cellular communication networks. These feedback loops collectively sustain an immunosuppressive milieu resistant to therapeutic intervention, necessitating a multi-targeted therapeutic approach.
Feedback loops between metabolic and structural barriers
Metabolic reprogramming and ECM remodeling form a tightly interconnected suppressive axis within the TME. Hypoxia-induced glycolysis elevates lactate production, which acidifies the TME and stabilizes HIF-1α, promoting TAM polarization and VEGF-mediated angiogenesis[103,104]. Simultaneously, CAF-driven ECM remodeling disrupts vascular integrity, worsening hypoxia and reinforcing nutrient deprivation[105,106]. This dual mechanism generates physical and metabolic barriers that hinder immune cell infiltration and function, creating immune-excluded zones. Disrupting these feedback loops through ECM normalization and metabolic targeting holds promise for reversing immune suppression.
Systemic coordination through cellular communication
Beyond localized interactions, the TME exerts systemic immune modulation via EVs and immunomodulatory signaling. TDEs transport PD-L1 and IDO, amplifying immune suppression by suppressing TCR signaling and depleting critical nutrients[107,108]. Similarly, the cGAS-STING pathway bridges local and systemic immunity by initiating type I interferon responses but can paradoxically sustain immunosuppression through chronic MDSC recruitment and inflammation[96-99]. These mechanisms emphasize the need to target both local and systemic immunosuppressive drivers.
The challenge of adaptive resistance
The TME’s resilience is driven by its adaptive capacity. For instance, VEGF inhibition may transiently normalize vasculature but induce compensatory recruitment of suppressive TAMs and MDSCs[109-112]. Similarly, targeting metabolic vulnerabilities like lactate production risks triggering oxidative stress, further impairing CTL activity[113,114]. These dynamic adaptations highlight the limitations of single-target therapies, underscoring the importance of multi-modal strategies that disrupt interdependent suppressive networks and sustain immune activation.
HETEROGENEITY IN THE TME
The TME operates as a dynamic and adaptive system driven by interlinked suppressive networks involving metabolic shifts, cellular communication, and structural remodeling. However, the degree to which these mechanisms manifest is far from uniform. TME exhibit striking heterogeneity shaped by distinct tumor subtypes, mutational landscapes, and external pressures from therapeutic interventions. This spatial and molecular diversity creates unique immune landscapes within different lung cancer subtypes, posing a significant challenge for developing universal therapeutic strategies. Understanding this heterogeneity is essential for tailoring precision therapies that target the specific vulnerabilities of each immune-suppressive niche[33-35] [Figure 2].
Figure 2. Integrative analysis of tumor heterogeneity and emerging technologies in lung cancer immunotherapy. This concentric illustration highlights the multi-dimensional heterogeneity of the TME, emphasizing molecular, cellular, and spatial complexity in lung cancer. Molecular heterogeneity, driven by genetic mutations (e.g., EGFR, KRAS) and epigenetic alterations activating oncogenic pathways such as RAS-MEK signaling, forms the core of tumor progression and immune evasion. Cellular heterogeneity emerges through complex interactions among immune and stromal cell subsets - including Tregs, NK cells, CTLs, MDSCs, TAMs, CAFs, and NETs - facilitating immunosuppression. At the outer layer, spatial heterogeneity reflects cell organization into immune-active hotspots and immunologically cold zones shaped by physical barriers, such as the ECM. Advanced technologies, including spatial omics, single-cell sequencing, liquid biopsy, organoids, AI analytics, and nanotechnology, enable precise characterization and therapeutic targeting of these heterogeneities. TME: Tumor microenvironment; EGFR: epidermal growth factor receptor; KRAS: Kirsten rat sarcoma viral oncogene homolog; RAS-MEK: RAS- mitogen-activated protein kinase kinase; Tregs: regulatory T cells; NK cells: natural killer cells; CTLs: cytotoxic T lymphocytes; MDSCs: myeloid-derived suppressor cells; TAMs: tumor-associated macrophages; CAFs: cancer-associated fibroblasts; ECM: extracellular matrix; NETs: neutrophil extracellular traps.
Molecular heterogeneity: genetic and epigenetic drivers
Molecular heterogeneity arises from genetic mutations, epigenetic modifications, and transcriptomic variability, creating distinct immunological profiles within NSCLC subtypes. EGFR, KRAS, and ALK alterations influence TMB, neoantigen presentation, and immune evasion. Epigenetic modifiers, such as histone deacetylases, further regulate immune gene expression, shaping the suppressive molecular milieu. Comprehensive molecular profiling enables subtype-specific therapeutic approaches, including combining ICIs with targeted inhibitors or epigenetic reprogrammers[12-15,19-23].
Cellular heterogeneity: diverse immunosuppressive ecosystems
The cellular landscape of the TME is defined by a dynamic balance between immune effector and suppressor populations. While the presence of Tregs, MDSCs, and TAMs is well-characterized, emerging players like neutrophils, B cells, and progenitor-like Tex contribute additional complexity. In NSCLC, distinct immune cell compositions correlate with specific molecular subtypes. For instance, EGFR-mutant adenocarcinomas exhibit elevated Tregs and low effector T cell infiltration, while SCCs display robust but suppressed immune infiltrates due to extensive immune checkpoint upregulation[22,26,28-30,33,37,48,51,55]. The balance among these components shapes the immune contexture and determines the therapeutic potential of immunotherapy.
Spatial heterogeneity: immune zoning and functional niches
Spatial compartmentalization within the TME creates immune-exclusive zones that limit the effectiveness of immunotherapy. Tumor cores often exhibit dense ECM barriers, hypoxic niches, and metabolic deprivation, forming “cold” regions resistant to immune infiltration. Conversely, peritumoral regions may host active TLSs or effector T cell clusters, contributing to localized immune activation. Techniques such as spatial transcriptomics and multiplex imaging have revealed this heterogeneity, emphasizing the need for therapies targeting both immune-excluded and inflamed regions simultaneously[36,58,115-120].
Temporal heterogeneity: dynamic evolution of immune contexture
Tumor heterogeneity in NSCLC is a dynamic process that evolves across disease progression and under therapeutic pressure, known as temporal heterogeneity. Early immune-evasive mechanisms - marked by increased immune checkpoint expression (PD-L1, CTLA-4, IDO1) - occur even in preinvasive stages, accompanied by structural segregation of immune and epithelial cells, highlighting early temporal remodeling of the TME[121]. As lung adenocarcinoma progresses from preinvasive to invasive stages, genomic subclonal mutations accumulate, closely paralleling changes in immune infiltration patterns, including significantly increased infiltration of CD8+ T cells[122]. Single-cell analyses further reveal ongoing temporal evolution within tumor-infiltrating T cell populations, emphasizing continuous immune adaptation during tumor progression[123]. Importantly, therapeutic interventions, particularly anti-PD-1 therapies, profoundly reshape the tumor immune landscape by driving substantial infiltration of novel T cell clones, a process termed "clonal replacement," rather than merely reactivating existing Tex[124]. These findings collectively underscore temporal heterogeneity as a critical factor influencing treatment responses, advocating longitudinal TME monitoring through serial biopsies and spatial omics to optimize personalized NSCLC therapy.
The profound heterogeneity within the TME highlights the need for multifaceted therapeutic approaches. A single-target strategy is insufficient against such a complex, evolving ecosystem. Exploring innovative strategies to transform cold, immune-excluded tumors into hot, immune-responsive environments by leveraging new technologies and combination therapies is imperative for maximizing therapeutic potential.
STRATEGIES FOR COLD-TO-HOT TUMOR TRANSFORMATION
Transforming immune-cold tumors into immune-reactive or “hot” tumors is a central challenge in overcoming immunotherapy resistance in NSCLC[116-120]. Achieving this requires targeting the interconnected mechanisms of immune suppression, cellular exclusion, and metabolic reprogramming within TME[33-35]. Building on the insights into shared mechanisms and subtype-specific heterogeneity, strategies to enhance immune infiltration, counteract suppressive cells, reactivate dormant immune pathways, and modulate metabolic constraints form the cornerstone of this transformation. By integrating these approaches with advanced technologies and combination therapies, it becomes possible to dismantle cold tumor barriers and unlock the full potential of immunotherapy, enabling sustained and effective antitumor responses [Figure 1 and Table 2].
Strategies for cold tumor transformation and overcoming resistance
Combination strategy | Underlying mechanism | Preclinical/Clinical evidence | Challenges and future directions | References |
VEGF inhibitors | Normalizes vasculature, improving oxygenation and T cell infiltration | Atezolizumab + bevacizumab showed clinical benefits (IMbrave150) | Requires optimized dosing and biomarker-based patient selection | [125-131] |
ECM-targeting approaches | Disrupts fibrotic barriers and reprograms CAFs to support immune infiltration | Antifibrotic agents (e.g., losartan) and ECM-degrading enzymes (e.g., PEGPH20) enhanced CTL migration in preclinical studies | Long-term safety and combination strategies need validation | [132-139] |
Chemokine modulation | Rebalances chemokine gradients to enhance CTL recruitment and limit MDSC/TAM attraction | CCR2 inhibitors block CCL2, and engineered DCs expressing CXCL9/10 restore CTL attraction in preclinical studies | Requires validation of long-term efficacy and safety of gene-editing technologies (e.g., CRISPR) | [140-146] |
Treg-targeting therapies | Depletes Tregs in the TME while sparing effector T cells using CCR4 antagonists, anti-CD25 agents, and anti-CTLA-4 variants | Anti-CTLA-4 variants with modified Fc domains show preclinical success in enhancing antitumor immunity | Requires balancing Treg depletion with maintaining immune tolerance to avoid autoimmunity | [147-149] |
MDSC-targeting therapies | Inhibit MDSC-mediated suppression via arginase inhibitors (e.g., INCB001158), IDO inhibitors (e.g., epacadostat), and CXCR2 blockade | INCB001158 + pembrolizumab demonstrated enhanced antitumor activity in phase I/II trials for solid tumors | Requires integration of real-time MDSC profiling to optimize timing and combination strategies | [150-152] |
TAM reprogramming | Converts TAMs from M2-like (immunosuppressive) to M1-like (pro-inflammatory) states using CSF-1R inhibitors, PI3Kγ inhibitors, and synthetic TLR or STING agonists | CSF-1R inhibitor LY3022855 and PI3Kγ inhibitors demonstrated enhanced M1 polarization and T cell priming in phase I trials | Optimizing combination with ICIs and addressing potential off-target effects | [153-156] |
TDE-targeting therapies | Disrupt TDE secretion via neutral sphingomyelinase inhibitors (e.g., GW4869) or neutralize TDE cargo (e.g., PD-L1) using engineered nanobodies | Nanobodies neutralizing exosomal PD-L1 reversed T cell exhaustion in preclinical models | Translating exosome-editing therapies into clinical applications requires addressing delivery and specificity challenges | [157-159] |
TCR signaling modulation | Restores TCR function through dual checkpoint blockade (e.g., PD-1/LAG-3) and engineered T cell therapies, including CAR-T cells with advanced costimulatory domains | PD-1/LAG-3 blockade demonstrated improved T cell persistence in clinical trials; CAR-T cells integrating ICOS or 4-1BB costimulation enhanced antitumor responses in preclinical models | Balancing specificity and off-target risks in CAR-T design; optimizing scalability of allogeneic CAR-T platforms | [160-168] |
Memory T cell enhancement | Promotes memory T cell differentiation and survival via IL-7/IL-15 superagonists, mitochondrial health regulation (PGC-1α), and mRNA vaccines targeting neoantigens | IL-7/IL-15 agonists and neoantigen mRNA vaccines have shown clinical efficacy in enhancing long-term tumor control | Optimizing personalized vaccine platforms and addressing variability in T cell memory formation | [169-176] |
NK cell restoration | Enhances NK cell cytotoxicity via metabolic reprogramming (e.g., AMPK agonists) and precise targeting with bispecific NK engagers (e.g., AFM24) | CAR-NKs and bispecific NK engagers (e.g., AFM24) have shown superior persistence and tumor targeting in clinical trials | Overcoming TME resistance and optimizing NK cell delivery systems for broad clinical application | [177-180] |
DC activation strategies | Enhances antigen cross-presentation and adaptive immunity through TLR agonists (e.g., TLR7/8), STING agonists (e.g., ADU-S100), and DC vaccines | DC vaccines loaded with tumor-specific peptides demonstrated improved T cell responses in checkpoint inhibitor-resistant NSCLC patients | Optimizing delivery systems (e.g., biomaterial scaffolds) and ensuring long-term safety of adjuvant therapies | [181-187] |
Immune pathway activation |
Reprograms suppressive TME through RIG-I agonists (e.g., FLT3L analogs) and cGAS-STING agonists (e.g., ADU-S100), enhancing antigen presentation and type I interferon signaling | RIG-I agonists synergized with checkpoint inhibitors to improve antigen cross-presentation in preclinical studies; ADU-S100 demonstrated innate and adaptive immune activation in early-phase trials | Overcoming variability in patient responses and optimizing delivery mechanisms for pathway-specific agonists | [188,189] |
Tumor vaccines | Neoantigen-based mRNA vaccines deliver precise tumor antigen targeting, enhancing T cell priming and memory formation | BioNTech’s individualized mRNA vaccines showed clinical efficacy in enhancing antitumor immunity | Optimizing vaccine personalization and ensuring scalability for broader clinical applications | [190-192] |
Oncolytic viruses (OVs) |
Combines tumor lysis with immune activation; engineered OVs release cytokines (e.g., GM-CSF) to enhance antigen presentation and immune recruitment | T-VEC and CG0070 demonstrated clinical efficacy in enhancing immune responses and tumor control | Addressing resistance to viral infection and optimizing cytokine payloads for safety and efficacy | [193,194] |
Cancer-specific adjuvants | Enhance APC activation and immune responses through TLR7/8 agonists, STING activators, and synthetic adjuvants, amplifying vaccine efficacy | Adjuvants combined with mRNA vaccines and OVs improved immune responses in checkpoint-refractory patients | Optimizing adjuvant combinations to minimize toxicity and ensure robust immune activation | [199-202] |
Glycolysis and Lactate targeting | Reduces lactate-driven immunosuppression by inhibiting glycolytic enzymes (e.g., LDHA) and lactate transporters (e.g., MCT1) | MCT1 inhibitor AZD3965 demonstrated immune activation and metabolic correction in early-phase trials | Addressing potential off-target effects and optimizing combination with ICIs | [199-201] |
Nutrient rebalancing | Restores T cell function under nutrient scarcity by blocking arginase/IDO pathways and modulating glucose allocation via GLUT inhibitors | IDO inhibitor epacadostat showed promising T cell restoration in preclinical studies | Balancing tumor and immune cell metabolism to minimize unintended effects on normal tissues | [202-204] |
Mitochondrial function enhancement | Restores immune cell metabolism by boosting mitochondrial biogenesis (e.g., PGC-1α agonists) and reducing oxidative stress via ROS scavengers | PGC-1α agonists and mitochondrial autophagy enhancers showed improved T cell persistence and antitumor immunity in preclinical studies | Balancing oxidative stress mitigation with sustained immune activation to avoid overcompensation | [205-213] |
Multi-modal immuno-metabolic reprogramming | Combines ICIs with metabolic agents to counteract lactate buildup (e.g., LDH/MCT1 inhibitors) and restore amino acid availability (e.g., IDO inhibitors) | LDH/MCT1 inhibitors and IDO blockade demonstrated enhanced CTL responses and metabolic reprogramming in preclinical studies | Developing dual-target therapies to optimize glycolysis inhibition and mitochondrial function without impairing normal tissues | [67,108,113,199-201,214] |
Vascular remodeling with immune Co-activation | Combines angiogenesis inhibitors (e.g., VEGF/ANG2 blockers) with immune activators (e.g., STING agonists) to normalize vasculature and enhance T cell priming | Anti-VEGF + STING agonist combinations improved immune infiltration and antigen presentation in preclinical models | Balancing vascular normalization with sustained immune activation; optimizing multi-receptor antibody therapies for safety | [126-128,131,215-219] |
Dynamic immune-network modulation | Disrupts immune exclusion via complement inhibitors (e.g., C3a/C5a blockers), NETosis inhibitors, and TLS-enhancing therapies (e.g., CXCL13 inducers) | Complement inhibitors and TLS enhancers demonstrated improved immune infiltration and pro-immunity niches in preclinical studies | Optimizing combinatory approaches to balance immune activation and mitigate potential off-target effects | [220-225] |
Personalized therapeutic frameworks | Integrates cGAS-STING activators, DC vaccines, and oncolytic viruses with engineered cytokines to drive durable immune activation | cGAS-STING activators and DC vaccines showed enhanced immune responses and tumor control in clinical and preclinical studies | Balancing multi-pathway targeting to reduce toxicity and ensure adaptability for resistant tumors | [185,187,193-195] |
Enhancing immune infiltration
Effective immune infiltration is pivotal for overcoming immunotherapy resistance in NSCLC, yet this is often obstructed by structural, biochemical, and cellular barriers. Current advances target tumor vasculature normalization, ECM remodeling, and chemokine rebalancing to promote effector immune cell infiltration and activation.
Overcoming physical barriers
Dysfunctional tumor vasculature driven by VEGF overexpression leads to hypoxia, nutrient deprivation, and immune exclusion. VEGF also downregulates endothelial adhesion molecules essential for T cell trafficking[85,86,125]. New-generation VEGF inhibitors, including bispecific antibodies targeting VEGF and Ang2 (e.g., BI 836880), stabilize tumor vasculature, enhancing immune cell extravasation[126-128]. Moreover, combinations of VEGF inhibitors with ICIs, such as atezolizumab plus bevacizumab, have shown significant clinical benefit by improving oxygenation and effector T cell access[18,20,129-131].
The ECM, largely shaped by CAFs, forms a fibrotic barrier that limits immune infiltration while fostering tumor progression[132,133]. Emerging ECM-targeting approaches, including antifibrotic agents (e.g., losartan)[134,135] and ECM-degrading enzymes (e.g., PEGPH20 targeting hyaluronan)[136,137], disrupt this barrier. Additionally, CAF reprogramming therapies leveraging retinoic acid receptor agonists or CXCR4 inhibitors promote a shift from pro-tumorigenic to immune-supportive fibroblasts, facilitating CTL migration[138,139].
Chemokine rebalancing and immune cell trafficking
Chemokine dysregulation skews immune cell recruitment toward suppressive phenotypes. Tumors frequently secrete CCL2 and CXCL5 to attract MDSCs and TAMs while reducing CXCL9/10, thereby limiting CTL recruitment. Precision-targeted therapies aim to reverse these gradients: CCR2 inhibitors block CCL2-mediated MDSC recruitment, while DCs engineered to express CXCL9/10 restore CTL attraction[140-144]. Furthermore, gene-editing technologies such as CRISPR-Cas9 enable in vivo chemokine modulation, enhancing immune surveillance[145,146].
These integrative strategies collectively reshape the tumor milieu, enabling deeper and more sustained immune infiltration, ultimately converting immune-excluded NSCLC into immune-responsive, treatment-sensitive tumors.
Modulating immunosuppressive mechanisms
The immunosuppressive landscape of NSCLC is sustained by intricate networks involving Tregs, MDSCs, TAMs, and TDEs. Disrupting these suppressive pathways is pivotal for reshaping the TME into a more immune-permissive state.
Targeting tregs
Tregs mediate immune suppression by producing IL-10 and TGF-β while inhibiting effector T cells. Strategies target Treg recruitment and function using chemokine inhibitors, such as CCR4 antagonists[147]. Engineered monoclonal antibodies, including anti-CD25 agents with selective depletion properties, reduce peripheral and intratumoral Treg populations while sparing activated effector T cells[148]. Emerging candidates, such as anti-CTLA-4 variants with modified Fc domains, specifically deplete Tregs in the TME, enhancing antitumor immunity[149].
Disrupting MDSCs
MDSCs suppress immune responses through arginine depletion, oxidative stress induction, and T cell inhibition via immune checkpoints such as PD-L1. Selective inhibitors of arginase (INCB001158) and IDO (epacadostat) are advancing in clinical trials[150,151]. CXCR2 inhibitors (e.g., SX-682) prevent MDSC recruitment and reverse immune exclusion[152]. Multi-omics profiling now supports real-time MDSC monitoring, enabling precision-targeted interventions that adjust based on the tumor’s evolving immune landscape[119,120].
Reprogramming TAMs
TAMs exist along a phenotypic continuum from immunosuppressive M2-like to pro-inflammatory M1-like states. TAM reprogramming strategies include CSF-1R inhibitors (e.g., LY3022855), which reduce M2-TAM recruitment[153]. Small-molecule inhibitors targeting PI3Kγ further enhance M1 polarization, promoting phagocytic activity and boosting T cell priming[154]. Novel synthetic TLR agonists and cGAS-STING activators induce IFN-I production, reinforcing macrophage-driven antitumor responses while complementing checkpoint inhibitors[155,156].
Neutralizing TDEs
TDEs act as vectors for immunosuppressive molecules, including PD-L1, IDO, and suppressive RNAs[93,94]. Innovative inhibitors targeting exosome biogenesis, such as neutral sphingomyelinase blockers (e.g., GW4869), disrupt TDE secretion[157]. Additionally, engineered nanobodies neutralizing exosomal PD-L1 have demonstrated the reversal of T cell exhaustion in preclinical models[158]. RNA-based exosome-editing therapies are emerging as a cutting-edge approach for altering the immunosuppressive cargo of TDEs[159].
By integrating these precision-driven strategies, it becomes possible to dismantle the suppressive TME and restore antitumor immune activity. This comprehensive approach holds promise for advancing immunotherapy effectiveness and prolonging survival in patients with NSCLC.
Activating dormant immune pathways
Reactivating dormant immune pathways in NSCLC is essential for reversing immunosuppression and enhancing therapeutic responses. Cutting-edge strategies aim to restore TCR signaling, promote memory T cell formation, reestablish NK cell cytotoxicity, enhance DCs antigen presentation, and leverage emerging immune activation pathways for sustained antitumor immunity.
Reinvigorating adaptive immunity
Enhancing TCR signaling
Restoring TCR signaling is crucial for reversing T cell exhaustion in NSCLC. Dual checkpoint blockade, such as PD-1/LAG-3 inhibition, enhances effector function and T cell persistence[160,161]. TCR-engineered therapies combined with checkpoint inhibitors bypass TME-associated suppression, driving durable antitumor responses[162].
Next-generation cellular therapies integrate advanced costimulatory domains like 4-1BB or ICOS and secrete IL-18 or PD-1-blocking agents to resist TME-induced inhibition[163-165]. Logic-gated chimeric antigen receptors (CAR)-T cells targeting dual antigens enhance specificity, minimizing off-tumor effects[166,167]. Gene-edited allogeneic CAR-T platforms offer scalable off-the-shelf solutions, expediting clinical deployment[168].
Synthetic TCR platforms and TCR-mimic antibodies broaden therapeutic reach by targeting tumor antigens beyond classical TCR recognition. These innovations collectively advance TCR signaling modulation, enhancing adaptive immune activation for durable responses in immunotherapy-resistant NSCLC.
Promoting memory T Cell formation
Long-term immune surveillance hinges on the formation of memory T cells. IL-7 and IL-15 superagonists are pivotal for promoting memory differentiation and survival[169,170]. Recent studies on BCL-6 and TCF-1 activation have highlighted their roles in driving central memory T cell development[140,171]. Additionally, mitophagy regulation through PGC-1α enhances mitochondrial health, enabling memory T cell persistence[172,173].
mRNA vaccine platforms, including personalized neoantigen vaccines, have emerged as promising tools for inducing potent memory T cell response[174-176]. These approaches have shown clinical efficacy by promoting long-term tumor control through persistent antigen stimulation.
Reactivating innate immunity
Restoring NK cell function
Recent breakthroughs in metabolic reprogramming have overcome TME-imposed NK cell dysfunction. AMPK agonists and mTOR inhibitors have restored NK cell metabolism, enhancing cytotoxicity even under nutrient-deprived conditions[177]. Bispecific NK cell engagers, which link NK cells to tumor-specific antigens, such as AFM24, have demonstrated precise tumor targeting with reduced toxicity[178].
Engineered NK cells expressing chimeric antigen receptors (CAR-NKs) have entered clinical trials, exhibiting superior persistence and resistance to TME suppression compared to traditional NK cell therapies[179]. Additionally, IL-12-based cytokine therapies such as NKTR-214 have redefined NK cell persistence in adoptive cell transfer protocols[180].
Boosting antigen presentation via DC activation
DC activation is crucial for initiating adaptive immune responses. Novel TLR agonists (e.g., TLR7/8 and TLR9 agonists) enhance DC maturation, promoting effective antigen cross-presentation[181-184]. Additionally, STING agonists such as ADU-S100 amplify type I interferon production, enhancing DC recruitment and activation[185].
Recent innovations include implantable biomaterial scaffolds delivering sustained antigen and adjuvant release, creating localized immune activation hubs[186]. Precision DC vaccines loaded with tumor-specific peptides have shown success in driving polyclonal T cell responses and enhancing response rates in checkpoint inhibitor-resistant NSCLC patients[187].
Leveraging immune pathways
Emerging immune activation pathways, including RIG-I/MAVS and cGAS-STING, have gained prominence in reprogramming the suppressive TME. Selective RIG-I agonists, such as FLT3L analogs, have amplified antigen cross-presentation while synergizing with checkpoint inhibitors[188]. Meanwhile, cGAS-STING agonists like ADU-S100 have shown promise in activating both innate and adaptive immune responses by boosting type I interferon signaling[189].
Immunotherapy enhancers
Tumor vaccines
Neoantigen-based personalized vaccines, powered by next-generation mRNA technology, have enabled precise tumor antigen targeting. Recent breakthroughs include multi-epitope mRNA vaccines delivering sustained T cell priming and memory formation[190,191]. Platforms like BioNTech’s individualized cancer vaccine have demonstrated clinical efficacy in boosting antitumor immunity[192].
Oncolytic viruses
Oncolytic viruses (OVs), such as T-VEC and CG0070, have emerged as dual-action agents, combining direct tumor lysis with immune activation[193]. Recent advances include tumor-selective OVs armed with immune-stimulating cytokines, such as GM-CSF, enhancing antigen release and immune cell recruitment[194].
Cancer-specific adjuvants
Cancer-specific adjuvants, including TLR7/8 agonists, STING activators, and synthetic adjuvants, have amplified vaccine responses by enhancing APC activation[195-197]. Combined with mRNA vaccines and OVs, these adjuvants have elevated immune responses in checkpoint-refractory patients[198].
Coordinating adaptive and innate immunity is key to overcoming tumor immune evasion and driving durable antitumor responses.
Addressing metabolic dysregulation
Reprogramming the TME necessitates counteracting metabolic dysregulation, a major driver of immune suppression and therapeutic resistance. Tumor cells’ altered metabolism fuels immunosuppressive niches, starving immune cells and creating metabolic barriers. Advanced strategies target key metabolic axes to sustain antitumor immunity and restore TME balance.
Targeting tumor glycolysis and lactate accumulation
Tumor cells’ dependence on aerobic glycolysis produces lactate, acidifying the TME and impairing CTLs and NK cells. Targeting glycolytic regulators such as hexokinase-2 and lactate dehydrogenase A (LDHA) has demonstrated potential in reducing lactate-driven immunosuppression[66,69,199]. Inhibiting lactate transporters like MCT1 prevents extracellular lactate buildup, alleviating its inhibitory effects on TCR signaling and promoting antigen-specific responses[113,200]. Advanced inhibitors such as AZD3965 have entered clinical evaluation, offering metabolic correction and immune activation[201].
Rebalancing nutrient availability
Metabolic competition for essential nutrients like glucose, amino acids, and lipids intensifies within the TME. Tumor-driven depletion of arginine and tryptophan through arginase and IDO pathways disrupts T cell proliferation. Dual blockade of these enzymes, using IDO inhibitors like epacadostat, has shown promise in preclinical models[202]. Recent efforts extend to modulating glucose allocation by targeting glucose transporter (GLUT) or promoting selective nutrient uptake in T cells through engineered cytokine delivery, sustaining effector functions under nutrient scarcity[203,204].
Enhancing mitochondrial function
Mitochondrial fitness underpins immune cell survival, activation, and persistence. Tumor-induced oxidative stress damages mitochondrial integrity, suppressing T cell metabolism. Boosting mitochondrial biogenesis through PGC-1α agonists restores metabolic resilience, supporting cytotoxic immune responses[173,205]. Agents like ROS scavengers and mitochondrial autophagy enhancers such as urushiol A improve oxidative phosphorylation and mitochondrial autophagy separately, promoting memory T cell formation and durable antitumor immunity[206-208].
Emerging metabolic interventions incorporate next-generation strategies such as epigenetic metabolic reprogramming[209,210], engineered cytokines targeting TME metabolic hubs[211,212], and multi-target small molecules that correct key metabolic imbalances[205,213]. Combining these strategies with ICIs or adoptive cell therapies holds transformative potential, establishing metabolically optimized TMEs conducive to durable antitumor responses[202,205,209,213].
Combining strategies for synergistic effects
Addressing the complexity of the TME requires multi-layered therapeutic combinations that target interconnected immunosuppressive, metabolic, and structural pathways. Integrating cutting-edge strategies promises to recalibrate the immune landscape and optimize therapeutic efficacy.
Multi-modal immuno-metabolic reprogramming
Emerging therapies integrate ICIs with metabolic rewiring agents targeting lactate metabolism, amino acid replenishment, and mitochondrial restoration[67]. LDH/MCT1 inhibitors counteract lactate accumulation, enhancing T cell function[113,199,201], while IDO blockade restores tryptophan availability, reactivating CTL responses[75,76,108]. Novel dual-target therapies simultaneously inhibit glycolysis and fuel oxidative phosphorylation, supporting sustained effector cell activation in resistant tumors[214].
Vascular remodeling with immune co-activation
Agents targeting VEGF, ANG2, and endothelin pathways simultaneously normalize vasculature, reduce hypoxia, and promote immune infiltration[86,126-128,131]. Combination strategies pairing anti-VEGF therapies with STING agonists, or engineered cytokines and Adoptive cell transfer amplify antigen presentation and T cell priming[215-218]. Novel multi-receptor antibodies linking angiogenesis inhibitors with immune activators are reshaping the therapeutic landscape, enhancing both vascular function and immune accessibility[219].
Dynamic immune-network modulation
Disrupting immune exclusion mechanisms is advancing through complement-targeting agents, including C3a/C5a inhibitors, which block suppressive feedback loops[220,221]. NETosis inhibitors paired with ECM remodelers clear physical barriers while restoring effector cell infiltration[222]. TLS enhancers, driven by CXCL13-inducing therapies, reshape the immune landscape by establishing pro-immunity niches[223-225]. B cell-directed immunotherapies targeting suppressive signaling redefine B cell functions, supporting antitumor immunity[225,226].
Next-generation adaptive combinations
Personalized therapeutic frameworks leveraging cGAS-STING activators, DC vaccines, and OVs are redefining immune activation[185,187,193]. These platforms synergize with engineered cytokines and T cell-stimulating agents, driving durable immune responses[165,167,169,172,173,194,195]. Emerging multi-pathway therapies targeting immune suppressors, metabolic stress sensors, and checkpoint regulators offer precision-driven, adaptive immune reprogramming for treatment-refractory tumors[156,160,173].
These integrative strategies represent a paradigm shift in immune oncology, forging new pathways to overcome TME resistance and reshape therapeutic outcomes in NSCLC.
Safety considerations of combination strategies
Despite their promising therapeutic potential, integrative combination strategies may carry certain safety considerations. For example, combining ICIs with antiangiogenic agents could increase the risk of vascular-related adverse events such as hypertension and proteinuria[227]. Similarly, therapies targeting metabolic pathways to enhance immune responses might inadvertently alter systemic immune homeostasis, potentially heightening immune-related toxicities[228]. Additionally, combinations utilizing OVs and signaling modulators might trigger excessive inflammatory responses or cytokine release syndrome[229]. Therefore, careful clinical evaluation and rigorous monitoring remain crucial to effectively balancing efficacy and safety.
EMERGING TECHNOLOGIES AND BIOMARKERS
Building on the integration of multi-targeted therapeutic strategies, the next frontier lies in harnessing cutting-edge technologies and precision biomarkers. Advances in molecular profiling, spatial omics, and computational modeling are redefining how tumor heterogeneity is understood and targeted. These innovations enable precise patient stratification, dynamic therapy adjustment, and real-time monitoring, paving the way for next-generation immunotherapy approaches[21,22,26,118,120] [Figure 2 and Table 3].
Emerging technologies and applications in TME research
Technology | Advantages | Application example | Future prospects | References |
Spatial transcriptomics | Maps spatial and molecular heterogeneity of the TME | Defines NSCLC subtypes by analyzing TIM3-HAVCR2 and CD96-NECTIN1 interactions | Integrates spatial and molecular insights to refine patient stratification and guide immunotherapy | [118,119] |
Spatial proteomics | Provides multiplexed protein profiling to identify immune-structural dynamics | Detects CD163+ macrophage and FOXP3+ Treg niches in high-grade NSCLC | Develops therapies targeting immunosuppressive niches and spatial immune organization | [230] |
Multi-omics integration | Combines spatial and single-cell data to reveal metabolic and immune reprogramming | Identifies TAM-driven cholesterol efflux (via ABCA1) and iron export (via SLC40A1) as targets | Enhances precision therapies by linking metabolic remodeling with immune cell dynamics | [118] |
Predictive spatial modeling | Simulates TME dynamics to forecast treatment outcomes | Predicts cytotoxic T cell spatial proximity as a prognostic marker in NSCLC | Implements real-time TME monitoring to support adaptive immunotherapy strategies | [230] |
AI-powered spatial analytics | Unveils spatially resolved immune phenotypes (e.g., inflamed, immune-excluded) strongly linked to therapy response | AI-powered TIL analyzers predict ICI responsiveness and correlate TIL distribution with survival in NSCLC | Advances patient stratification and biomarker discovery to refine immunotherapy strategies | [233] |
GeoMx DSP | Quantifies immune and stromal markers across distinct TME compartments | Identifies spatial correlations of immune markers (e.g., CD56⁺ NK cells) with survival and resistance mechanisms (e.g., VISTA) | Enables actionable biomarker-based pathways to overcome immune evasion and personalize treatments | [234] |
Liquid biopsy and CyTOF | Provides non-invasive, high-dimensional monitoring of immune cell dynamics and therapeutic response | Characterizes exhausted CD8⁺ T cells (e.g., CD8⁺CD101hiTIM3⁺) and identifies baseline immune predictors for PD-1 therapy | Optimizes real-time treatment monitoring and adaptive immunotherapy adjustments | [235,236] |
Nanoparticle-based delivery | Enables precise, localized delivery of immunomodulatory agents with minimal systemic toxicity | LNP-based RNA vaccines targeting KRAS mutations demonstrated durable immune responses in preclinical NSCLC models | Advances stimuli-responsive nanocarriers for targeted payload release in hypoxic and acidic TMEs | [237-243] |
Smart tumor-specific carriers | Enhances selectivity and efficacy through ligand-functionalized and TME-triggered delivery mechanisms | EGFR-functionalized nanoparticles achieved localized delivery while sparing healthy tissues in NSCLC models | Develops multifunctional nanocarriers integrating diagnostic and therapeutic capabilities (theranostics) | [244-248] |
ToC | Precise, real-time modeling of tumor-stroma interactions and immune dynamics; enables the assessment of personalized therapeutic responses | Evaluating patient-specific immune responses and resistance mechanisms to anti-PD-1 immunotherapy in NSCLC | Integration into clinical workflows for routine immunotherapy prediction and optimization | [231] |
PDOs | High-throughput screening of drug sensitivity; recapitulates patient-specific genomic and phenotypic tumor | Personalized ex vivo testing to predict clinical response and guide individualized NSCLC treatment strategies | Expansion into routine clinical practice for tailored precision oncology | [232] |
Decoding spatial and molecular heterogeneity
Understanding spatial and molecular heterogeneity in NSCLC has progressed from theoretical frameworks to actionable clinical insights, driven by advanced spatial profiling technologies. These technologies provide unprecedented clarity on how cellular composition and spatial organization within the TME influence therapeutic responses, reshaping NSCLC classification and treatment strategies[58,118,119].
Emerging spatial omics technologies, including spatial transcriptomics and multi-omics integration platforms, have transcended conventional histopathology by enabling molecular profiling with spatial resolution[36,119]. Recent large-scale studies integrating single-cell RNA sequencing (scRNA-seq) and spatial transcriptomics have redefined NSCLC subtypes by unveiling precise immune-epithelial interactions. For example, LUAD-specific immune landscapes feature TIM3-HAVCR2 interactions, while lung squamous cell carcinoma (LUSC) exhibits CD96-NECTIN1-mediated cytotoxicity evasion. This study identifies TAMs, especially STAB1+ macrophages, as reprogrammed with enhanced cholesterol efflux via ABCA1 and iron export through SLC40A1, fostering immune suppression and tumor progression, positioning them as key players in metabolic remodeling and promising therapeutic targets[118].
Beyond transcriptional profiling, spatial proteomics has emerged as a pivotal tool in dissecting protein-level heterogeneity. Technologies such as Imaging Mass Cytometry have enabled multiplexed spatial proteomics to map the NSCLC microenvironment with unprecedented resolution. This study uncovered key immune-structural dynamics, including the enrichment of CD163+ macrophage and FOXP3+ Treg interactions in high-grade tumors, creating immunosuppressive niches. In contrast, CD8+ T cells in low-grade tumors showed stronger spatial proximity to tumor cells, indicating enhanced cytotoxic potential and better prognostic implications. Additionally, B cell-enriched neighborhoods were associated with improved survival, underscoring the spatial organization of immune cells as a determinant of clinical outcomes. These findings advocate for therapeutic strategies tailored to the spatial architecture of TIME[230].
Recent advancements in 3D ex vivo cancer models, particularly tumor-on-chip (ToC) and patient-derived organoids (PDOs), have further complemented spatial and molecular characterization efforts. The lung ToC system effectively recapitulates tumor-stroma interactions and immune cell dynamics within a physiologically relevant context, allowing precise, real-time assessment of patient-specific immune responses to anti-PD-1 therapies[231]. Similarly, patient-derived lung organoids accurately capture genomic and phenotypic heterogeneity of clinical tumors, serving as robust platforms for ex vivo drug sensitivity screening and personalized response predictions[232]. Despite current limitations, including variable efficiency in organoid establishment and challenges in sustaining long-term fidelity to original tumor phenotypes, these innovative 3D models represent critical advancements for personalized medicine.
Looking ahead, the fusion of spatial omics, predictive modeling, and high-throughput clinical screening holds the promise of transforming NSCLC treatment paradigms. By integrating these technologies into clinical workflows, researchers aim to achieve continuous, real-time monitoring of TME dynamics, enabling precision-guided immunotherapy.
Leveraging novel biomarkers for precision therapy
The heterogeneous nature of NSCLC necessitates an adaptive biomarker-driven treatment strategy. The integration of spatial omics and artificial intelligence-driven analytics has catalyzed biomarker discovery with unprecedented precision. Recently, an AI-powered spatial tumor-infiltrating lymphocytes (TILs) analyzer has demonstrated that the spatial distribution of TILs reveals critical immune phenotypes - such as inflamed, immune-excluded, and immune-desert - strongly associated with ICI responsiveness, tumor mutational burden, and patient survival, highlighting the value of AI analytics in enhancing biomarker interpretability and therapeutic prediction[233].
Offering a distinct perspective, the GeoMx Digital Spatial Profiling (DSP) System has significantly advanced the deciphering of spatial TME architecture in NSCLC. By quantifying immune markers across distinct compartments - tumor, immune cells, macrophages, and stroma - it has revealed spatial immune associations, such as enriched CD56+ NK/NKT cells and CD4+ T cells correlating positively with patient survival. Conversely, elevated VISTA and CD127 expression within tumor compartments indicate immune-resistance mechanisms, highlighting actionable pathways to overcome immune evasion. These findings underscore spatially resolved immune markers’ critical role in refining patient stratification and personalized immunotherapy[234].
Furthermore, liquid biopsy has emerged as a cornerstone of real-time tumor monitoring, complemented by cytometry by time-of-flight (CyTOF), which enables high-dimensional, longitudinal immune-cell characterization from peripheral blood. CyTOF and multiplex cytokine profiling studies in NSCLC patients receiving anti-PD-1 therapy have shown that higher baseline frequencies of exhausted CD8+ T cells, particularly the CD8+CD101hiTIM3+ subset, correlate with poor therapeutic outcomes. In contrast, increased expression of cytotoxic markers, such as Granzyme B and T-bet, identifies potential responders. Baseline immune cell profiles - including classical monocytes, NK cells, and ICOS+ CD4+ T cells - also predict pembrolizumab efficacy, supporting non-invasive approaches for treatment monitoring and optimization[235,236].
However, despite these technological advances, limitations remain. AI-powered analytics and spatial profiling techniques often require extensive high-quality datasets and complex computational infrastructures. Additionally, current platforms face constraints in the resolution, sensitivity, and interpretability of results, necessitating further technological refinement and validation. Addressing these challenges will accelerate the translation of multi-omics technologies, liquid biopsy innovations, and AI-driven predictive models into routine clinical practice, marking the next frontier in precision oncology for NSCLC.
Precision durg delivery through nanotechnology
Recent advancements in nanoparticle-based delivery platforms have demonstrated remarkable potential in improving immunotherapy efficacy. Lipid nanoparticles (LNPs), as seen in mRNA vaccine technology, enable precise delivery of tumor-specific antigens, cytokines, and siRNAs targeting key immunosuppressive genes. Preclinical and early clinical studies have reported durable immune responses with LNP-based RNA vaccines targeting KRAS mutations in NSCLC, highlighting their translational potential[237,238]. Similarly, polymeric nanoparticles engineered for controlled release of immunomodulatory agents, such as PD-L1 inhibitors and STING agonists, have demonstrated superior therapeutic outcomes with minimal systemic toxicity[239-241]. Notably, hypoxia-responsive polymeric carriers incorporating oxygen boosters have successfully enhanced T cell infiltration in preclinical NSCLC models, offering a novel approach to overcoming hypoxic TME barriers[242,243].
Tumor-specific nanocarriers that leverage ligand-functionalization and stimuli-responsive mechanisms provide enhanced selectivity and therapeutic efficacy. Nanoparticles functionalized with EGFR antibodies or folate receptor ligands have shown promising results by achieving localized drug delivery while sparing healthy tissues[244,245]. In parallel, TME-triggered carriers that respond to acidic pH, enzymatic activity, or oxidative stress ensure precise payload release at the tumor site[246-248]. Such smart delivery platforms are rapidly advancing toward clinical application, driven by their ability to amplify therapeutic effects while minimizing off-target toxicity.
CONCLUSION
Advancing immunotherapy in NSCLC requires overcoming the intricate web of TME-driven resistance. This review has highlighted pivotal mechanisms such as immune suppression, metabolic reprogramming, and spatial heterogeneity that hinder therapeutic efficacy. Despite significant progress, persistent challenges remain in understanding dynamic TME remodeling and its evolving interplay with emerging therapies.
Future research must focus on leveraging spatial multi-omics, real-time biomarker monitoring, and precision delivery systems to enhance treatment specificity and adaptability. Single-cell technologies integrated with spatial proteomics and AI-powered predictive models hold the potential to uncover novel therapeutic targets while enabling patient-specific therapeutic designs. These innovations will likely redefine how we interpret TME dynamics and guide adaptive therapy regimens. Clinical translation will benefit from next-generation combination therapies, including ICIs paired with metabolic modulators, TME-targeting agents, and personalized cancer vaccines. Expanding biomarker-driven patient stratification and optimizing treatment timing through longitudinal molecular profiling will further improve therapeutic outcomes. Achieving sustainable progress in NSCLC immunotherapy will require multi-disciplinary collaboration spanning oncology, bioinformatics, immunology, and materials science.
Looking ahead, the integration of nanotechnology, AI-driven analytics, and immunotherapy offers an unprecedented opportunity to overcome resistance mechanisms and reshape the treatment landscape of NSCLC. As research converges toward personalized and adaptive immunotherapy, the path toward long-term survival and even potential cures becomes more tangible, driving a hopeful future in the fight against lung cancer.
DECLARATIONS
Authors’ contributions
Made contributions to conception and design: Kong X, Feng Y, Yu J
Writing and revising articles: Yu J, Kong X, Feng Y
Availability of data and materials
Not applicable.
Financial support and sponsorship
This work was supported by the National Natural Science Foundation of China (82370582 to Kong X), and Shanghai Frontiers Science Center of Disease and Syndrome Biology of Inflammatory Cancer Transformation.
Conflicts of interest
All authors declared that there are no conflicts of interest.
Ethical approval and consent to participate
Not applicable.
Consent for publication
Not applicable.
Copyright
© The Author(s) 2025.
REFERENCES
1. Bray F, Laversanne M, Sung H, et al. Global cancer statistics 2022: GLOBOCAN estimates of incidence and mortality worldwide for 36 cancers in 185 countries. CA Cancer J Clin. 2024;74:229-63.
2. Howlader N, Forjaz G, Mooradian MJ, et al. The effect of advances in lung-cancer treatment on population mortality. N Engl J Med. 2020;383:640-9.
3. Johnson ML, Cho BC, Luft A, et al; POSEIDON investigators. Durvalumab with or without tremelimumab in combination with chemotherapy as first-line therapy for metastatic non-small-cell lung cancer: the phase III POSEIDON study. J Clin Oncol. 2023;41:1213-27.
4. Paz-Ares L, Ciuleanu TE, Cobo M, et al. First-line nivolumab plus ipilimumab combined with two cycles of chemotherapy in patients with non-small-cell lung cancer (CheckMate 9LA): an international, randomised, open-label, phase 3 trial. Lancet Oncol. 2021;22:198-211.
5. Paz-Ares L, Vicente D, Tafreshi A, et al. A randomized, placebo-controlled trial of pembrolizumab plus chemotherapy in patients with metastatic squamous NSCLC: protocol-specified final analysis of KEYNOTE-407. J Thorac Oncol. 2020;15:1657-69.
6. Garassino MC, Gadgeel S, Speranza G, et al. Pembrolizumab plus pemetrexed and platinum in nonsquamous non-small-cell lung cancer: 5-year outcomes from the phase 3 KEYNOTE-189 study. J Clin Oncol. 2023;41:1992-8.
7. Schoenfeld JD, Giobbie-Hurder A, Ranasinghe S, et al. Durvalumab plus tremelimumab alone or in combination with low-dose or hypofractionated radiotherapy in metastatic non-small-cell lung cancer refractory to previous PD(L)-1 therapy: an open-label, multicentre, randomised, phase 2 trial. Lancet Oncol. 2022;23:279-91.
8. de Castro G Jr, Kudaba I, Wu YL, et al. Five-year outcomes with pembrolizumab versus chemotherapy as first-line therapy in patients with non-small-cell lung cancer and programmed death ligand-1 tumor proportion score ≥ 1% in the KEYNOTE-042 study. J Clin Oncol. 2023;41:1986-91.
9. Schoenfeld AJ, Hellmann MD. Acquired resistance to immune checkpoint inhibitors. Cancer Cell. 2020;37:443-55.
10. Gettinger SN, Wurtz A, Goldberg SB, et al. Clinical features and management of acquired resistance to PD-1 axis inhibitors in 26 patients with advanced non-small cell lung cancer. J Thorac Oncol. 2018;13:831-9.
11. Schoenfeld AJ, Antonia SJ, Awad MM, et al. Clinical definition of acquired resistance to immunotherapy in patients with metastatic non-small-cell lung cancer. Ann Oncol. 2021;32:1597-607.
12. Ricciuti B, Lamberti G, Puchala SR, et al. Genomic and immunophenotypic landscape of acquired resistance to PD-(L)1 blockade in non-small-cell lung cancer. J Clin Oncol. 2024;42:1311-21.
13. Li Y, Hu L, Peng X, Xu H, Tang B, Xu C. Resistance to immune checkpoint inhibitors in KRAS-mutant non-small cell lung cancer. Cancer Drug Resist. 2022;5:129-46.
14. Passaro A, Peters S. Setting the benchmark for KRASG12C -mutated NSCLC. N Engl J Med. 2022;387:180-3.
15. Liu C, Zheng S, Jin R, et al. The superior efficacy of anti-PD-1/PD-L1 immunotherapy in KRAS-mutant non-small cell lung cancer that correlates with an inflammatory phenotype and increased immunogenicity. Cancer Lett. 2020;470:95-105.
16. Herbst RS, Giaccone G, de Marinis F, et al. Atezolizumab for first-line treatment of PD-L1-selected patients with NSCLC. N Engl J Med. 2020;383:1328-39.
17. Socinski MA, Jotte RM, Cappuzzo F, et al; IMpower150 Study Group. Atezolizumab for first-line treatment of metastatic nonsquamous NSCLC. N Engl J Med. 2018;378:2288-301.
18. Shiraishi Y, Kishimoto J, Sugawara S, et al. Atezolizumab and platinum plus pemetrexed with or without bevacizumab for metastatic nonsquamous non-small cell lung cancer: a phase 3 randomized clinical trial. JAMA Oncol. 2024;10:315-24.
19. Watanabe S, Furuya N, Nakamura A, et al. A phase II study of atezolizumab with bevacizumab, carboplatin, and paclitaxel for patients with EGFR-mutated NSCLC after TKI treatment failure (NEJ043 study). Eur J Cancer. 2024;197:113469.
20. Provencio M, Ortega AL, Coves-Sarto J, et al. Atezolizumab plus bevacizumab as first-line treatment for patients with metastatic nonsquamous non-small cell lung cancer with high tumor mutation burden: a nonrandomized controlled trial. JAMA Oncol. 2023;9:344-53.
21. Nassar AH, Jayakrishnan R, Feng J, et al. Consolidation ALK tyrosine kinase inhibitors versus durvalumab or observation after chemoradiation in unresectable stage III ALK-positive NSCLC. J Thorac Oncol. 2025;20:109-18.
22. Li H, Liu J, Zhang L, et al. Mutation-guided chemotherapy-free strategy in first-line immunotherapy for low PD-L1-expressing non-squamous NSCLC. J Immunother Cancer. 2024;12:e009693.
23. Skoulidis F, Goldberg ME, Greenawalt DM, et al.
24. Zhou C, Hu Y, Arkania E, et al; ASTRUM-004 Investigators. A global phase 3 study of serplulimab plus chemotherapy as first-line treatment for advanced squamous non-small-cell lung cancer (ASTRUM-004). Cancer Cell. 2024;42:198-208.e3.
25. Xu JY, Zhang C, Wang X, et al. Integrative proteomic characterization of human lung adenocarcinoma. Cell. 2020;182:245-61.e17.
26. Soltis AR, Bateman NW, Liu J, et al; APOLLO Research Network. Proteogenomic analysis of lung adenocarcinoma reveals tumor heterogeneity, survival determinants, and therapeutically relevant pathways. Cell Rep Med. 2022;3:100819.
27. Devarakonda S, Li Y, Martins Rodrigues F, et al. Genomic profiling of lung adenocarcinoma in never-smokers. J Clin Oncol. 2021;39:3747-58.
28. Chen J, Yang H, Teo ASM, et al. Genomic landscape of lung adenocarcinoma in East Asians. Nat Genet. 2020;52:177-86.
29. Wang C, Yu Q, Song T, et al. The heterogeneous immune landscape between lung adenocarcinoma and squamous carcinoma revealed by single-cell RNA sequencing. Signal Transduct Target Ther. 2022;7:289.
30. Lau SCM, Pan Y, Velcheti V, Wong KK. Squamous cell lung cancer: current landscape and future therapeutic options. Cancer Cell. 2022;40:1279-93.
31. Satpathy S, Krug K, Jean Beltran PM, et al; Clinical Proteomic Tumor Analysis Consortium. A proteogenomic portrait of lung squamous cell carcinoma. Cell. 2021;184:4348-71.e40.
32. Guo JH, Ma YS, Lin JW, et al. Whole-exome and targeted gene sequencing of large-cell lung carcinoma reveals recurrent mutations in the PI3K pathway. Br J Cancer. 2023;129:366-73.
33. Bagaev A, Kotlov N, Nomie K, et al. Conserved pan-cancer microenvironment subtypes predict response to immunotherapy. Cancer Cell. 2021;39:845-65.e7.
34. Binnewies M, Roberts EW, Kersten K, et al. Understanding the tumor immune microenvironment (TIME) for effective therapy. Nat Med. 2018;24:541-50.
35. Bejarano L, Jordāo MJC, Joyce JA. Therapeutic targeting of the tumor microenvironment. Cancer Discov. 2021;11:933-59.
36. Yan Y, Sun D, Hu J, et al. Multi-omic profiling highlights factors associated with resistance to immuno-chemotherapy in non-small-cell lung cancer. Nat Genet. 2025;57:126-39.
37. Tay C, Tanaka A, Sakaguchi S. Tumor-infiltrating regulatory T cells as targets of cancer immunotherapy. Cancer Cell. 2023;41:450-65.
38. Exposito F, Redrado M, Houry M, et al. PTEN loss confers resistance to anti-PD-1 therapy in non-small cell lung cancer by increasing tumor infiltration of regulatory T cells. Cancer Res. 2023;83:2513-26.
39. Kumagai S, Koyama S, Itahashi K, et al. Lactic acid promotes PD-1 expression in regulatory T cells in highly glycolytic tumor microenvironments. Cancer Cell. 2022;40:201-18.e9.
40. Skoulidis F, Araujo HA, Do MT, et al. CTLA4 blockade abrogates KEAP1/STK11-related resistance to PD-(L)1 inhibitors. Nature. 2024;635:462-71.
41. Yin N, Liu Y, Weems C, et al. Protein kinase Cι mediates immunosuppression in lung adenocarcinoma. Sci Transl Med. 2022;14:eabq5931.
42. Li R, Salehi-Rad R, Crosson W, et al. Inhibition of granulocytic myeloid-derived suppressor cells overcomes resistance to immune checkpoint inhibition in LKB1-deficient non-small cell lung cancer. Cancer Res. 2021;81:3295-308.
43. La Fleur L, Botling J, He F, et al. Targeting MARCO and IL37R on immunosuppressive macrophages in lung cancer blocks regulatory T cells and supports cytotoxic lymphocyte function. Cancer Res. 2021;81:956-67.
44. Yang L, Li A, Yu W, et al. Blockade of purine metabolism reverses macrophage immunosuppression and enhances anti-tumor immunity in non-small cell lung cancer. Drug Resist Updat. 2025;78:101175.
45. Liu M, Tong Z, Ding C, et al. Transcription factor c-Maf is a checkpoint that programs macrophages in lung cancer. J Clin Invest. 2020;130:2081-96.
46. Granville CA, Memmott RM, Balogh A, et al. A central role for Foxp3+ regulatory T cells in K-Ras-driven lung tumorigenesis. PLoS One. 2009;4:e5061.
47. Zdanov S, Mandapathil M, Abu Eid R, et al. Mutant KRAS conversion of conventional T cells into regulatory T cells. Cancer Immunol Res. 2016;4:354-65.
48. Lasser SA, Ozbay Kurt FG, Arkhypov I, Utikal J, Umansky V. Myeloid-derived suppressor cells in cancer and cancer therapy. Nat Rev Clin Oncol. 2024;21:147-64.
49. Zhang H, Liu L, Liu J, et al. Roles of tumor-associated macrophages in anti-PD-1/PD-L1 immunotherapy for solid cancers. Mol Cancer. 2023;22:58.
50. Bai R, Li Y, Jian L, Yang Y, Zhao L, Wei M. The hypoxia-driven crosstalk between tumor and tumor-associated macrophages: mechanisms and clinical treatment strategies. Mol Cancer. 2022;21:177.
51. Adrover JM, McDowell SAC, He XY, Quail DF, Egeblad M. NETworking with cancer: the bidirectional interplay between cancer and neutrophil extracellular traps. Cancer Cell. 2023;41:505-26.
52. Lei Q, Zhen S, Zhang L, Zhao Q, Yang L, Zhang Y. A2AR-mediated CXCL5 upregulation on macrophages promotes NSCLC progression via NETosis. Cancer Immunol Immunother. 2024;73:108.
53. Wang Y, Liu F, Chen L, et al. Neutrophil extracellular traps (NETs) promote non-small cell lung cancer metastasis by suppressing lncRNA MIR503HG to activate the NF-κB/NLRP3 inflammasome pathway. Front Immunol. 2022;13:867516.
55. Fridman WH, Meylan M, Petitprez F, Sun CM, Italiano A, Sautès-Fridman C. B cells and tertiary lymphoid structures as determinants of tumour immune contexture and clinical outcome. Nat Rev Clin Oncol. 2022;19:441-57.
56. Liu Y, Xiong L, Chen Y, et al. Complete pathological remission and tertiary lymphoid structures are associated with the efficacy of resectable NSCLC receiving neoadjuvant chemoimmunotherapy: a double-center retrospective study. Hum Vaccin Immunother. 2023;19:2285902.
57. Patil NS, Nabet BY, Müller S, et al. Intratumoral plasma cells predict outcomes to PD-L1 blockade in non-small cell lung cancer. Cancer Cell. 2022;40:289-300.e4.
58. Hao D, Han G, Sinjab A, et al. The single-cell immunogenomic landscape of B and plasma cells in early-stage lung adenocarcinoma. Cancer Discov. 2022;12:2626-45.
59. Gao J, Zhang LX, Ao YQ, et al. Elevated circASCC3 limits antitumor immunity by sponging miR-432-5p to upregulate C5a in non-small cell lung cancer. Cancer Lett. 2022;543:215774.
60. Gebhardt T, Park SL, Parish IA. Stem-like exhausted and memory CD8+ T cells in cancer. Nat Rev Cancer. 2023;23:780-98.
62. Li H, Liu Z, Liu L, et al. AXL targeting restores PD-1 blockade sensitivity of STK11/LKB1 mutant NSCLC through expansion of TCF1+ CD8 T cells. Cell Rep Med. 2022;3:100554.
63. Koh J, Kim S, Woo YD, et al. TCF1+PD-1+ tumour-infiltrating lymphocytes predict a favorable response and prolonged survival after immune checkpoint inhibitor therapy for non-small-cell lung cancer. Eur J Cancer. 2022;174:10-20.
64. Zhou Y, Cheng L, Liu L, Li X. NK cells are never alone: crosstalk and communication in tumour microenvironments. Mol Cancer. 2023;22:34.
65. Myers JA, Miller JS. Exploring the NK cell platform for cancer immunotherapy. Nat Rev Clin Oncol. 2021;18:85-100.
66. Brand A, Singer K, Koehl GE, et al. LDHA-associated lactic acid production blunts tumor immunosurveillance by T and NK cells. Cell Metab. 2016;24:657-71.
67. Faubert B, Solmonson A, De Berardinis RJ. Metabolic reprogramming and cancer progression. Science. 2020;368:eaaw5473.
68. Poznanski SM, Singh K, Ritchie TM, et al. Metabolic flexibility determines human NK cell functional fate in the tumor microenvironment. Cell Metab. 2021;33:1205-20.e5.
69. Feng J, Yang H, Zhang Y, et al. Tumor cell-derived lactate induces TAZ-dependent upregulation of PD-L1 through GPR81 in human lung cancer cells. Oncogene. 2017;36:5829-39.
70. Elia I, Rowe JH, Johnson S, et al. Tumor cells dictate anti-tumor immune responses by altering pyruvate utilization and succinate signaling in CD8+ T cells. Cell Metab. 2022;34:1137-50.e6.
71. Zhang D, Tang Z, Huang H, et al. Metabolic regulation of gene expression by histone lactylation. Nature. 2019;574:575-80.
72. Liu N, Luo J, Kuang D, et al. Lactate inhibits ATP6V0d2 expression in tumor-associated macrophages to promote HIF-2α-mediated tumor progression. J Clin Invest. 2019;129:631-46.
73. Sosnowska A, Chlebowska-Tuz J, Matryba P, et al. Inhibition of arginase modulates T-cell response in the tumor microenvironment of lung carcinoma. Oncoimmunology. 2021;10:1956143.
74. Miret JJ, Kirschmeier P, Koyama S, et al. Suppression of myeloid cell arginase activity leads to therapeutic response in a NSCLC mouse model by activating anti-tumor immunity. J Immunother Cancer. 2019;7:32.
75. Yamasuge W, Yamamoto Y, Fujigaki H, et al. Indoleamine 2,3-dioxygenase 2 depletion suppresses tumor growth in a mouse model of Lewis lung carcinoma. Cancer Sci. 2019;110:3061-7.
76. Luke JJ, Gelmon K, Siu LL, et al. Phase 1/2 study of the indoleamine 2,3-dioxygenase 1 inhibitor linrodostat mesylate combined with nivolumab or nivolumab and ipilimumab in advanced solid tumors or hematologic malignancies. Clin Cancer Res. 2024;Epub ahead of print.
77. Huang M, Xiong D, Pan J, et al. Targeting glutamine metabolism to enhance immunoprevention of EGFR-driven lung cancer. Adv Sci. 2022;9:e2105885.
78. Galan-Cobo A, Sitthideatphaiboon P, Qu X, et al. LKB1 and KEAP1/NRF2 pathways cooperatively promote metabolic reprogramming with enhanced glutamine dependence in KRAS-mutant lung adenocarcinoma. Cancer Res. 2019;79:3251-67.
79. Chen X, Liu Y, Wang Y, et al. CYP4F2-catalyzed metabolism of arachidonic acid promotes stromal cell-mediated immunosuppression in non-small cell lung cancer. Cancer Res. 2022;82:4016-30.
80. Kerk SA, Papagiannakopoulos T, Shah YM, Lyssiotis CA. Metabolic networks in mutant KRAS-driven tumours: tissue specificities and the microenvironment. Nat Rev Cancer. 2021;21:510-25.
81. Ugolini A, Tyurin VA, Tyurina YY, et al. Polymorphonuclear myeloid-derived suppressor cells limit antigen cross-presentation by dendritic cells in cancer. JCI Insight. 2020;5:138581.
82. Menzner AK, Rottmar T, Voelkl S, et al. Hydrogen-peroxide synthesis and LDL-uptake controls immunosuppressive properties in monocyte-derived dendritic cells. Cancers. 2021;13:461.
83. Wu F, Yang J, Liu J, et al. Signaling pathways in cancer-associated fibroblasts and targeted therapy for cancer. Signal Transduct Target Ther. 2021;6:218.
84. Cruz-Bermúdez A, Laza-Briviesca R, Vicente-Blanco RJ, et al. Cancer-associated fibroblasts modify lung cancer metabolism involving ROS and TGF-β signaling. Free Radic Biol Med. 2019;130:163-73.
85. Fukumura D, Kloepper J, Amoozgar Z, Duda DG, Jain RK. Enhancing cancer immunotherapy using antiangiogenics: opportunities and challenges. Nat Rev Clin Oncol. 2018;15:325-40.
86. Liu Y, Li Y, Wang Y, et al. Recent progress on vascular endothelial growth factor receptor inhibitors with dual targeting capabilities for tumor therapy. J Hematol Oncol. 2022;15:89.
87. Di Carlo SE, Raffenne J, Varet H, et al. Depletion of slow-cycling PDGFRα+ADAM12+ mesenchymal cells promotes antitumor immunity by restricting macrophage efferocytosis. Nat Immunol. 2023;24:1867-78.
89. Zhao Y, Jia Y, Wang J, et al. circNOX4 activates an inflammatory fibroblast niche to promote tumor growth and metastasis in NSCLC via FAP/IL-6 axis. Mol Cancer. 2024;23:47.
90. Kuo IY, Yang YE, Yang PS, et al. Converged Rab37/IL-6 trafficking and STAT3/PD-1 transcription axes elicit an immunosuppressive lung tumor microenvironment. Theranostics. 2021;11:7029-44.
91. Matsuda S, Revandkar A, Dubash TD, et al. TGF-β in the microenvironment induces a physiologically occurring immune-suppressive senescent state. Cell Rep. 2023;42:112129.
92. Yue M, Hu S, Sun H, et al. Extracellular vesicles remodel tumor environment for cancer immunotherapy. Mol Cancer. 2023;22:203.
93. de Miguel-Perez D, Ak M, Mamindla P, et al. Validation of a multiomic model of plasma extracellular vesicle PD-L1 and radiomics for prediction of response to immunotherapy in NSCLC. J Exp Clin Cancer Res. 2024;43:81.
94. Eslami-S Z, Cortés-Hernández LE, Sinoquet L, et al. Circulating tumour cells and PD-L1-positive small extracellular vesicles: the liquid biopsy combination for prognostic information in patients with metastatic non-small cell lung cancer. Br J Cancer. 2024;130:63-72.
95. de Miguel-Perez D, Russo A, Gunasekaran M, et al. Baseline extracellular vesicle TGF-β is a predictive biomarker for response to immune checkpoint inhibitors and survival in non-small cell lung cancer. Cancer. 2023;129:521-30.
97. Liu Z, Wang D, Zhang J, et al. cGAS-STING signaling in the tumor microenvironment. Cancer Lett. 2023;577:216409.
98. Hou Y, Liang H, Rao E, et al. Non-canonical NF-κB antagonizes STING sensor-mediated DNA sensing in radiotherapy. Immunity. 2018;49:490-503.e4.
99. Liang H, Deng L, Hou Y, et al. Host STING-dependent MDSC mobilization drives extrinsic radiation resistance. Nat Commun. 2017;8:1736.
100. Thoresen D, Wang W, Galls D, Guo R, Xu L, Pyle AM. The molecular mechanism of RIG-I activation and signaling. Immunol Rev. 2021;304:154-68.
101. Huang T, Ao X, Liu J, et al. m6A methyltransferase METTL3 promotes non-small-cell lung carcinoma progression by inhibiting the RIG-I-MAVS innate immune pathway. Transl Oncol. 2025;51:102230.
102. Jiang Y, Zhang H, Wang J, et al. Exploiting RIG-I-like receptor pathway for cancer immunotherapy. J Hematol Oncol. 2023;16:8.
103. Colegio OR, Chu NQ, Szabo AL, et al. Functional polarization of tumour-associated macrophages by tumour-derived lactic acid. Nature. 2014;513:559-63.
104. Cui Z, Ruan Z, Li M, et al. Intermittent hypoxia inhibits anti-tumor immune response via regulating PD-L1 expression in lung cancer cells and tumor-associated macrophages. Int Immunopharmacol. 2023;122:110652.
105. Zhang F, Ma Y, Li D, et al. Cancer associated fibroblasts and metabolic reprogramming: unraveling the intricate crosstalk in tumor evolution. J Hematol Oncol. 2024;17:80.
106. Kugeratski FG, Atkinson SJ, Neilson LJ, et al. Hypoxic cancer-associated fibroblasts increase NCBP2-AS2/HIAR to promote endothelial sprouting through enhanced VEGF signaling. Sci Signal. 2019;12:eaan8247.
107. Chen G, Huang AC, Zhang W, et al. Exosomal PD-L1 contributes to immunosuppression and is associated with anti-PD-1 response. Nature. 2018;560:382-6.
108. Zhou C, Zhang Y, Yan R, et al. Exosome-derived miR-142-5p remodels lymphatic vessels and induces IDO to promote immune privilege in the tumour microenvironment. Cell Death Differ. 2021;28:715-29.
109. Bauer R, Udonta F, Wroblewski M, et al. Blockade of myeloid-derived suppressor cell expansion with all-trans retinoic acid increases the efficacy of antiangiogenic therapy. Cancer Res. 2018;78:3220-32.
110. Patel SA, Herynk MH, Cascone T, et al. Estrogen promotes resistance to bevacizumab in murine models of NSCLC. J Thorac Oncol. 2021;16:2051-64.
111. Dalton HJ, Pradeep S, McGuire M, et al. Macrophages facilitate resistance to anti-VEGF therapy by altered VEGFR expression. Clin Cancer Res. 2017;23:7034-46.
112. La Fargue CJ, Amero P, Noh K, et al. Overcoming adaptive resistance to anti-VEGF therapy by targeting CD5L. Nat Commun. 2023;14:2407.
113. Ammar N, Hildebrandt M, Geismann C, et al. Monocarboxylate transporter-1 (MCT1)-mediated lactate uptake protects pancreatic adenocarcinoma cells from oxidative stress during glutamine scarcity thereby promoting resistance against inhibitors of glutamine metabolism. Antioxidants. 2023;12:1818.
114. Garcia I, Cornely K, Peterson CN, Berkmen MB. Roles of the oncometabolite enantiomers of 2-hydroxyglutarate and their metabolism by diverse dehydrogenases. Essays Biochem. 2024;68:161-71.
115. Chen C, Guo Q, Liu Y, et al. Single-cell and spatial transcriptomics reveal POSTN+ cancer-associated fibroblasts correlated with immune suppression and tumour progression in non-small cell lung cancer. Clin Transl Med. 2023;13:e1515.
116. Wu F, Fan J, He Y, et al. Single-cell profiling of tumor heterogeneity and the microenvironment in advanced non-small cell lung cancer. Nat Commun. 2021;12:2540.
117. Lambrechts D, Wauters E, Boeckx B, et al. Phenotype molding of stromal cells in the lung tumor microenvironment. Nat Med. 2018;24:1277-89.
118. De Zuani M, Xue H, Park JS, et al. Single-cell and spatial transcriptomics analysis of non-small cell lung cancer. Nat Commun. 2024;15:4388.
119. Enfield KSS, Colliver E, Lee C, et al; TRACERx consortium. Spatial architecture of myeloid and T cells orchestrates immune evasion and clinical outcome in lung cancer. Cancer Discov. 2024;14:1018-47.
120. Takano Y, Suzuki J, Nomura K, et al. Spatially resolved gene expression profiling of tumor microenvironment reveals key steps of lung adenocarcinoma development. Nat Commun. 2024;15:10637.
121. Mascaux C, Angelova M, Vasaturo A, et al. Immune evasion before tumour invasion in early lung squamous carcinogenesis. Nature. 2019;571:570-5.
122. Zhang C, Zhang J, Xu FP, et al. Genomic landscape and immune microenvironment features of preinvasive and early invasive lung adenocarcinoma. J Thorac Oncol. 2019;14:1912-23.
123. Zhang L, Yu X, Zheng L, et al. Lineage tracking reveals dynamic relationships of T cells in colorectal cancer. Nature. 2018;564:268-72.
124. Yost KE, Satpathy AT, Wells DK, et al. Clonal replacement of tumor-specific T cells following PD-1 blockade. Nat Med. 2019;25:1251-9.
125. Lanitis E, Irving M, Coukos G. Targeting the tumor vasculature to enhance T cell activity. Curr Opin Immunol. 2015;33:55-63.
126. Hidalgo M, Martinez-Garcia M, Le Tourneau C, et al. First-in-human phase I study of single-agent vanucizumab, a first-in-class bispecific anti-angiopoietin-2/anti-VEGF-A antibody, in adult patients with advanced solid tumors. Clin Cancer Res. 2018;24:1536-45.
127. Kienast Y, Klein C, Scheuer W, et al. Ang-2-VEGF-A CrossMab, a novel bispecific human IgG1 antibody blocking VEGF-A and Ang-2 functions simultaneously, mediates potent antitumor, antiangiogenic, and antimetastatic efficacy. Clin Cancer Res. 2013;19:6730-40.
128. Hofmann I, Baum A, Hofmann MH, et al. Pharmacodynamic and antitumor activity of BI 836880, a dual vascular endothelial growth factor and angiopoietin 2 inhibitor, alone and combined with programmed cell death protein-1 inhibition. J Pharmacol Exp Ther. 2023;384:331-42.
129. Voron T, Colussi O, Marcheteau E, et al. VEGF-A modulates expression of inhibitory checkpoints on CD8+ T cells in tumors. J Exp Med. 2015;212:139-48.
130. Martino EC, Misso G, Pastina P, et al. Immune-modulating effects of bevacizumab in metastatic non-small-cell lung cancer patients. Cell Death Discov. 2016;2:16025.
131. Schmittnaegel M, Rigamonti N, Kadioglu E, et al. Dual angiopoietin-2 and VEGFA inhibition elicits antitumor immunity that is enhanced by PD-1 checkpoint blockade. Sci Transl Med. 2017;9:eaak9670.
132. Hauge A, Rofstad EK. Antifibrotic therapy to normalize the tumor microenvironment. J Transl Med. 2020;18:207.
133. Abyaneh HS, Regenold M, McKee TD, Allen C, Gauthier MA. Towards extracellular matrix normalization for improved treatment of solid tumors. Theranostics. 2020;10:1960-80.
134. Amirkhosravi A, Heidari MR, Karami-Mohajeri S, Torshabi M, Mandegary A, Mehrabani M. Losartan enhances the suppressive effect of pirfenidone on the bleomycin-induced epithelial-mesenchymal transition and oxidative stress in A549 cell line. Iran J Basic Med Sci. 2023;26:972-8.
135. Diop-Frimpong B, Chauhan VP, Krane S, Boucher Y, Jain RK. Losartan inhibits collagen I synthesis and improves the distribution and efficacy of nanotherapeutics in tumors. Proc Natl Acad Sci U S A. 2011;108:2909-14.
136. Whatcott CJ, Han H, Posner RG, Hostetter G, Von Hoff DD. Targeting the tumor microenvironment in cancer: why hyaluronidase deserves a second look. Cancer Discov. 2011;1:291-6.
137. Jeannot V, Gauche C, Mazzaferro S, et al. Anti-tumor efficacy of hyaluronan-based nanoparticles for the co-delivery of drugs in lung cancer. J Control Release. 2018;275:117-28.
138. Chan JSK, Sng MK, Teo ZQ, Chong HC, Twang JS, Tan NS. Targeting nuclear receptors in cancer-associated fibroblasts as concurrent therapy to inhibit development of chemoresistant tumors. Oncogene. 2018;37:160-73.
139. Wang Y, Lan W, Xu M, et al. Cancer-associated fibroblast-derived SDF-1 induces epithelial-mesenchymal transition of lung adenocarcinoma via CXCR4/β-catenin/PPARδ signalling. Cell Death Dis. 2021;12:214.
140. Liu Q, Wu N, Hou P. PRPS2-mediated modulation of the antitumor immune response in lung cancer through CCL2-mediated tumor-associated macrophages and myeloid-derived suppressor cells. Thorac Cancer. 2024;15:1739-48.
141. Mittal P, Wang L, Akimova T, et al. The CCR2/MCP-1 chemokine pathway and lung adenocarcinoma. Cancers. 2020;12:3723.
142. Pant A, Hwa-Lin Bergsneider B, Srivastava S, et al. CCR2 and CCR5 co-inhibition modulates immunosuppressive myeloid milieu in glioma and synergizes with anti-PD-1 therapy. Oncoimmunology. 2024;13:2338965.
143. Cheng CC, Chang J, Ho AS, et al. Tumor-intrinsic IFNα and CXCL10 are critical for immunotherapeutic efficacy by recruiting and activating T lymphocytes in tumor microenvironment. Cancer Immunol Immunother. 2024;73:175.
144. Lim RJ, Salehi-Rad R, Tran LM, et al. CXCL9/10-engineered dendritic cells promote T cell activation and enhance immune checkpoint blockade for lung cancer. Cell Rep Med. 2024;5:101479.
145. Melese ES, Franks E, Cederberg RA, et al. CCL5 production in lung cancer cells leads to an altered immune microenvironment and promotes tumor development. Oncoimmunology. 2022;11:2010905.
146. Tang N, Cheng C, Zhang X, et al. TGF-β inhibition via CRISPR promotes the long-term efficacy of CAR T cells against solid tumors. JCI Insight. 2020;5:133977.
147. Jackson JJ, Ketcham JM, Younai A, et al. Discovery of a potent and selective CCR4 antagonist that inhibits Treg trafficking into the tumor microenvironment. J Med Chem. 2019;62:6190-213.
148. Song D, Liu X, Dong C, et al. Two novel human anti-CD25 antibodies with antitumor activity inversely related to their affinity and in vitro activity. Sci Rep. 2021;11:22966.
149. Peng Y, Fu Y, Liu H, et al. Non-IL-2-blocking anti-CD25 antibody inhibits tumor growth by depleting Tregs and has synergistic effects with anti-CTLA-4 therapy. Int J Cancer. 2024;154:1285-97.
150. Naing A, Papadopoulos KP, Pishvaian MJ, et al. First-in-human phase 1 study of the arginase inhibitor INCB001158 alone or combined with pembrolizumab in patients with advanced or metastatic solid tumours. BMJ Oncol. 2024;3:e000249.
151. Mitchell TC, Hamid O, Smith DC, et al. Epacadostat plus pembrolizumab in patients with advanced solid tumors: phase i results from a multicenter, open-label phase I/II trial (ECHO-202/KEYNOTE-037). J Clin Oncol. 2018;36:3223-30.
152. Tang KH, Li S, Khodadadi-Jamayran A, et al. Combined inhibition of SHP2 and CXCR1/2 promotes antitumor T-cell response in NSCLC. Cancer Discov. 2022;12:47-61.
153. Falchook GS, Peeters M, Rottey S, et al. A phase 1a/1b trial of CSF-1R inhibitor LY3022855 in combination with durvalumab or tremelimumab in patients with advanced solid tumors. Invest New Drugs. 2021;39:1284-97.
154. Kaneda MM, Messer KS, Ralainirina N, et al. PI3Kγ is a molecular switch that controls immune suppression. Nature. 2016;539:437-42.
155. Müller E, Christopoulos PF, Halder S, et al. Toll-like receptor ligands and interferon-γ synergize for induction of antitumor M1 macrophages. Front Immunol. 2017;8:1383.
156. Yu J, Deng H, Xu Z. Targeting macrophage priming by polyphyllin VII triggers anti-tumor immunity via STING-governed cytotoxic T-cell infiltration in lung cancer. Sci Rep. 2020;10:21360.
157. Xu W, Patel N, Deng Y, Ding S, Wang T, Zhang H. Extracellular vesicle-derived LINC00482 induces microglial M2 polarization to facilitate brain metastasis of NSCLC. Cancer Lett. 2023;561:216146.
158. Xu W, Xu J, Li P, et al. Discovery and preclinical evaluation of BPB-101: a novel triple functional bispecific antibody targeting GARP-TGF-β complex/SLC, free TGF-β and PD-L1. Front Immunol. 2024;15:1479399.
159. Jiang M, Zhang K, Meng J, Xu L, Liu Y, Wei R. Engineered exosomes in service of tumor immunotherapy: from optimizing tumor-derived exosomes to delivering CRISPR/Cas9 system. Int J Cancer. 2025;156:898-913.
160. Luke JJ, Patel MR, Blumenschein GR, et al. The PD-1- and LAG-3-targeting bispecific molecule tebotelimab in solid tumors and hematologic cancers: a phase 1 trial. Nat Med. 2023;29:2814-24.
161. Ngiow SF, Manne S, Huang YJ, et al. LAG-3 sustains TOX expression and regulates the CD94/NKG2-Qa-1b axis to govern exhausted CD8 T cell NK receptor expression and cytotoxicity. Cell. 2024;187:4336-54.e19.
162. Adusumilli PS, Zauderer MG, Rusch VW, et al. Regional delivery of mesothelin-targeted CAR T cells for pleural cancers: safety and preliminary efficacy in combination with anti-PD-1 agent. JCO. 2019;37:2511.
163. Guedan S, Posey AD Jr, Shaw C, et al. Enhancing CAR T cell persistence through ICOS and 4-1BB costimulation. JCI Insight. 2018;3:96976.
164. Rafiq S, Yeku OO, Jackson HJ, et al. Targeted delivery of a PD-1-blocking scFv by CAR-T cells enhances anti-tumor efficacy in vivo. Nat Biotechnol. 2018;36:847-56.
165. Hu B, Ren J, Luo Y, et al. Augmentation of antitumor immunity by human and mouse CAR T cells secreting IL-18. Cell Rep. 2017;20:3025-33.
166. Tousley AM, Rotiroti MC, Labanieh L, et al. Co-opting signalling molecules enables logic-gated control of CAR T cells. Nature. 2023;615:507-16.
167. Bagley SJ, Logun M, Fraietta JA, et al. Intrathecal bivalent CAR T cells targeting EGFR and IL13Rα2 in recurrent glioblastoma: phase 1 trial interim results. Nat Med. 2024;30:1320-9.
168. Lei T, Wang Y, Zhang Y, et al. Leveraging CRISPR gene editing technology to optimize the efficacy, safety and accessibility of CAR T-cell therapy. Leukemia. 2024;38:2517-43.
169. Wrangle JM, Velcheti V, Patel MR, et al. ALT-803, an IL-15 superagonist, in combination with nivolumab in patients with metastatic non-small cell lung cancer: a non-randomised, open-label, phase 1b trial. Lancet Oncol. 2018;19:694-704.
170. Song Y, Liu Y, Hu R, Su M, Rood D, Lai L.
172. Zhong X, Wu H, Ouyang C, et al. Ncoa2 promotes CD8+ T cell-mediated antitumor immunity by stimulating T-cell activation via upregulation of PGC-1α critical for mitochondrial function. Cancer Immunol Res. 2023;11:1414-31.
173. Dumauthioz N, Tschumi B, Wenes M, et al. Enforced PGC-1α expression promotes CD8 T cell fitness, memory formation and antitumor immunity. Cell Mol Immunol. 2021;18:1761-71.
174. Li F, Deng L, Jackson KR, et al. Neoantigen vaccination induces clinical and immunologic responses in non-small cell lung cancer patients harboring EGFR mutations. J Immunother Cancer. 2021;9:e002531.
175. Patel MR, Bauer TM, Jimeno A, et al. A phase I study of mRNA-2752, a lipid nanoparticle encapsulating mRNAs encoding human OX40L, IL-23, and IL-36γ, for intratumoral (iTu) injection alone and in combination with durvalumab. JCO. 2020;38:3092.
176. Gangaev A, van Sleen Y, Brandhorst N, et al. mRNA-1273 vaccination induces polyfunctional memory CD4 and CD8 T cell responses in patients with solid cancers undergoing immunotherapy or/and chemotherapy. Front Immunol. 2024;15:1447555.
177. Crist M, Yaniv B, Palackdharry S, et al. Metformin increases natural killer cell functions in head and neck squamous cell carcinoma through CXCL1 inhibition. J Immunother Cancer. 2022;10:e005632.
178. Wingert S, Reusch U, Knackmuss S, et al. Preclinical evaluation of AFM24, a novel CD16A-specific innate immune cell engager targeting EGFR-positive tumors. MAbs. 2021;13:1950264.
179. Zolov S, Chuikov S, Katkam SK, Shield D, Keshamouni VG. Abstract 6360: a novel CAR-NK cell therapy to target lung adenocarcinoma cells. Cancer Research. 2024;84:6360.
180. Diab A, Tannir NM, Bentebibel SE, et al. Bempegaldesleukin (NKTR-214) plus nivolumab in patients with advanced solid tumors: phase I dose-escalation study of safety, efficacy, and immune activation (PIVOT-02). Cancer Discov. 2020;10:1158-73.
181. Costin D, Chen K, Gralla RJ, Garofalo B, Wang M, Kurland E. A phase 2 study of EIK1001, a Toll-like receptor 7/8 (TLR7/8) agonist, in combination with pembrolizumab and chemotherapy in patients with stage 4 non-small cell lung cancer. JCO. 2024;42:TPS8667.
182. Li S, Yao JC, Li JT, Schmidt AP, Link DC. TLR7/8 agonist treatment induces an increase in bone marrow resident dendritic cells and hematopoietic progenitor expansion and mobilization. Exp Hematol. 2021;96:35-43.e7.
183. Fernandez-Rodriguez L, Cianciaruso C, Bill R, et al. Dual TLR9 and PD-L1 targeting unleashes dendritic cells to induce durable antitumor immunity. J Immunother Cancer. 2023;11:e006714.
184. Garon EB, Spira AI, Johnson M, et al. A phase Ib open-label, multicenter study of inhaled DV281, a TLR9 agonist, in combination with nivolumab in patients with advanced or metastatic non-small cell lung cancer. Clin Cancer Res. 2021;27:4566-73.
185. Meric-Bernstam F, Sweis RF, Hodi FS, et al. Phase I dose-escalation trial of MIW815 (ADU-S100), an intratumoral STING agonist, in patients with advanced/metastatic solid tumors or lymphomas. Clin Cancer Res. 2022;28:677-88.
186. Srinivasan S, Babensee JE. Controlled delivery of immunomodulators from a biomaterial scaffold niche to induce a tolerogenic phenotype in human dendritic cells. ACS Biomater Sci Eng. 2020;6:4062-76.
187. Wang QT, Nie Y, Sun SN, et al. Tumor-associated antigen-based personalized dendritic cell vaccine in solid tumor patients. Cancer Immunol Immunother. 2020;69:1375-87.
188. Dong HP, Li Y, Tang Z, et al. Combined targeting of CCL7 and Flt3L to promote the expansion and infiltration of cDC1s in tumors enhances T-cell activation and anti-PD-1 therapy effectiveness in NSCLC. Cell Mol Immunol. 2023;20:850-3.
189. Luo J, Pang S, Hui Z, et al. Blocking Tim-3 enhances the anti-tumor immunity of STING agonist ADU-S100 by unleashing CD4+ T cells through regulating type 2 conventional dendritic cells. Theranostics. 2023;13:4836-57.
190. Ramalingam PS, Arumugam S. Reverse vaccinology and immunoinformatics approaches to design multi-epitope based vaccine against oncogenic KRAS. Med Oncol. 2023;40:283.
191. Dasari V, McNeil LK, Beckett K, et al. Lymph node targeted multi-epitope subunit vaccine promotes effective immunity to EBV in HLA-expressing mice. Nat Commun. 2023;14:4371.
193. Shalhout SZ, Miller DM, Emerick KS, Kaufman HL. Therapy with oncolytic viruses: progress and challenges. Nat Rev Clin Oncol. 2023;20:160-77.
194. Kemp V, van den Wollenberg DJM, Camps MGM, et al. Arming oncolytic reovirus with GM-CSF gene to enhance immunity. Cancer Gene Ther. 2019;26:268-81.
195. Soni D, Borriello F, Scott DA, et al. From hit to vial: precision discovery and development of an imidazopyrimidine TLR7/8 agonist adjuvant formulation. Sci Adv. 2024;10:eadg3747.
196. Herck S, Feng B, Tang L. Delivery of STING agonists for adjuvanting subunit vaccines. Adv Drug Deliv Rev. 2021;179:114020.
197. Pifferi C, Fuentes R, Fernández-Tejada A. Natural and synthetic carbohydrate-based vaccine adjuvants and their mechanisms of action. Nat Rev Chem. 2021;5:197-216.
198. Castro Eiro MD, Hioki K, Li L, et al. TLR9 plus STING agonist adjuvant combination induces potent neopeptide T cell immunity and improves immune checkpoint blockade efficacy in a tumor model. J Immunol. 2024;212:455-65.
199. Qiao T, Xiong Y, Feng Y, et al. Inhibition of LDH-A by oxamate enhances the efficacy of anti-PD-1 treatment in an NSCLC humanized mouse model. Front Oncol. 2021;11:632364.
200. Liu Y, Wang F, Peng D, et al. Activation and antitumor immunity of CD8+ T cells are supported by the glucose transporter GLUT10 and disrupted by lactic acid. Sci Transl Med. 2024;16:eadk7399.
201. Halford S, Veal GJ, Wedge SR, et al. A phase I dose-escalation study of AZD3965, an oral monocarboxylate transporter 1 inhibitor, in patients with advanced cancer. Clin Cancer Res. 2023;29:1429-39.
202. Powderly JD, Klempner SJ, Naing A, et al. Epacadostat plus pembrolizumab and chemotherapy for advanced solid tumors: results from the phase I/II ECHO-207/KEYNOTE-723 study. Oncologist. 2022;27:905-e848.
203. Fu H, Vuononvirta J, Fanti S, et al. The glucose transporter 2 regulates CD8+ T cell function via environment sensing. Nat Metab. 2023;5:1969-85.
204. Toledano Zur R, Atar O, Barliya T, et al. Genetically engineering glycolysis in T cells increases their antitumor function. J Immunother Cancer. 2024;12:e008434.
205. Wan H, Xu B, Zhu N, Ren B. PGC-1α activator-induced fatty acid oxidation in tumor-infiltrating CTLs enhances effects of PD-1 blockade therapy in lung cancer. Tumori. 2020;106:55-63.
206. Aksoylar HI, Patsoukis N. Treatment with exogenously added catalase alters CD8 T cell memory differentiation and function. Adv Biol. 2023;7:e2101320.
207. Ma S, Wu Q, Wu W, et al. Urolithin A hijacks ERK1/2-ULK1 cascade to improve CD8+ T cell fitness for antitumor immunity. Adv Sci. 2024;11:e2310065.
208. Denk D, Petrocelli V, Conche C, et al. Expansion of T memory stem cells with superior anti-tumor immunity by Urolithin A-induced mitophagy. Immunity. 2022;55:2059-73.e8.
209. Chen X, Pan X, Zhang W, et al. Epigenetic strategies synergize with PD-L1/PD-1 targeted cancer immunotherapies to enhance antitumor responses. Acta Pharm Sin B. 2020;10:723-33.
210. Franco F, Jaccard A, Romero P, Yu YR, Ho PC. Metabolic and epigenetic regulation of T-cell exhaustion. Nat Metab. 2020;2:1001-12.
211. Guo Y, Xie YQ, Gao M, et al. Metabolic reprogramming of terminally exhausted CD8+ T cells by IL-10 enhances anti-tumor immunity. Nat Immunol. 2021;22:746-56.
212. Naing A, Infante JR, Papadopoulos KP, et al. PEGylated IL-10 (Pegilodecakin) induces systemic immune activation, CD8+ T cell invigoration and polyclonal T cell expansion in cancer patients. Cancer Cell. 2018;34:775-91.e3.
213. Lontos K, Wang Y, Joshi SK, et al. Metabolic reprogramming via an engineered PGC-1α improves human chimeric antigen receptor T-cell therapy against solid tumors. J Immunother Cancer. 2023;11:e006522.
214. Chen J, Zou L, Lu G, et al. PFKP alleviates glucose starvation-induced metabolic stress in lung cancer cells via AMPK-ACC2 dependent fatty acid oxidation. Cell Discov. 2022;8:52.
215. Yang H, Lee WS, Kong SJ, et al. STING activation reprograms tumor vasculatures and synergizes with VEGFR2 blockade. J Clin Invest. 2019;129:4350-64.
216. Lanitis E, Rota G, Kosti P, et al. Optimized gene engineering of murine CAR-T cells reveals the beneficial effects of IL-15 coexpression. J Exp Med. 2021;218:e20192203.
217. Tao L, Huang G, Shi S, Chen L. Bevacizumab improves the antitumor efficacy of adoptive cytokine-induced killer cells therapy in non-small cell lung cancer models. Med Oncol. 2014;31:777.
218. Chinnasamy D, Yu Z, Kerkar SP, et al. Local delivery of interleukin-12 using T cells targeting VEGF receptor-2 eradicates multiple vascularized tumors in mice. Clin Cancer Res. 2012;18:1672-83.
219. Läubli H, Müller P, D’Amico L, Buchi M, Kashyap AS, Zippelius A. The multi-receptor inhibitor axitinib reverses tumor-induced immunosuppression and potentiates treatment with immune-modulatory antibodies in preclinical murine models. Cancer Immunol Immunother. 2018;67:815-24.
220. Ajona D, Ortiz-Espinosa S, Moreno H, et al. A combined PD-1/C5a blockade synergistically protects against lung cancer growth and metastasis. Cancer Discov. 2017;7:694-703.
221. Nandagopal S, Li CG, Xu Y, Sodji QH, Graves EE, Giaccia AJ. C3aR signaling inhibits NK-cell infiltration into the tumor microenvironment in mouse models. Cancer Immunol Res. 2022;10:245-58.
222. Albrengues J, Shields MA, Ng D, et al. Neutrophil extracellular traps produced during inflammation awaken dormant cancer cells in mice. Science. 2018;361:eaao4227.
223. Cho JW, Park S, Kim G, et al. Dysregulation of TFH-B-TRM lymphocyte cooperation is associated with unfavorable anti-PD-1 responses in EGFR-mutant lung cancer. Nat Commun. 2021;12:6068.
224. de Chaisemartin L, Goc J, Damotte D, et al. Characterization of chemokines and adhesion molecules associated with T cell presence in tertiary lymphoid structures in human lung cancer. Cancer Res. 2011;71:6391-9.
225. Bodogai M, Lee Chang C, Wejksza K, et al. Anti-CD20 antibody promotes cancer escape via enrichment of tumor-evoked regulatory B cells expressing low levels of CD20 and CD137L. Cancer Res. 2013;73:2127-38.
226. Wang Z, Cheng Q, Tang K, et al. Lipid mediator lipoxin A4 inhibits tumor growth by targeting IL-10-producing regulatory B (Breg) cells. Cancer Lett. 2015;364:118-24.
227. Gao L, Yang X, Yi C, Zhu H. Adverse events of concurrent immune checkpoint inhibitors and antiangiogenic agents: a systematic review. Front Pharmacol. 2019;10:1173.
228. Li H, Zhao A, Li M, Shi L, Han Q, Hou Z. Targeting T-cell metabolism to boost immune checkpoint inhibitor therapy. Front Immunol. 2022;13:1046755.
229. Zhu Z, McGray AJR, Jiang W, Lu B, Kalinski P, Guo ZS. Improving cancer immunotherapy by rationally combining oncolytic virus with modulators targeting key signaling pathways. Mol Cancer. 2022;21:196.
230. Sorin M, Rezanejad M, Karimi E, et al. Single-cell spatial landscapes of the lung tumour immune microenvironment. Nature. 2023;614:548-54.
231. Veith I, Nurmik M, Mencattini A, et al. Assessing personalized responses to anti-PD-1 treatment using patient-derived lung tumor-on-chip. Cell Rep Med. 2024;5:101549.
232. Taverna JA, Hung CN, Williams M, et al. Ex vivo drug testing of patient-derived lung organoids to predict treatment responses for personalized medicine. Lung Cancer. 2024;190:107533.
233. Park S, Ock CY, Kim H, et al. Artificial intelligence-powered spatial analysis of tumor-infiltrating lymphocytes as complementary biomarker for immune checkpoint inhibition in non-small-cell lung cancer. J Clin Oncol. 2022;40:1916-28.
234. Zugazagoitia J, Gupta S, Liu Y, et al. Biomarkers associated with beneficial PD-1 checkpoint blockade in non-small cell lung cancer (NSCLC) identified using high-plex digital spatial profiling. Clin Cancer Res. 2020;26:4360-8.
235. Leung EL, Li RZ, Fan XX, et al. Longitudinal high-dimensional analysis identifies immune features associating with response to anti-PD-1 immunotherapy. Nat Commun. 2023;14:5115.
236. Rochigneux P, Lisberg A, Garcia A, et al. Mass cytometry reveals classical monocytes, NK Cells, and ICOS+ CD4+ T cells associated with pembrolizumab efficacy in patients with lung cancer. Clin Cancer Res. 2022;28:5136-48.
237. Anthiya S, Öztürk SC, Yanik H, et al. Targeted siRNA lipid nanoparticles for the treatment of KRAS-mutant tumors. J Control Release. 2023;357:67-83.
238. Wang X, Wang W, Zou S, et al. Combination therapy of KRAS G12V mRNA vaccine and pembrolizumab: clinical benefit in patients with advanced solid tumors. Cell Res. 2024;34:661-4.
239. Liang J, Wang H, Ding W, et al. Nanoparticle-enhanced chemo-immunotherapy to trigger robust antitumor immunity. Sci Adv. 2020;6:eabc3646.
240. Park KS, Xu C, Sun X, Louttit C, Moon JJ. Improving STING agonist delivery for cancer immunotherapy using biodegradable mesoporous silica nanoparticles. Adv Ther. 2020;3:2000130.
241. Kim GB, Sung HD, Nam GH, et al. Design of PD-1-decorated nanocages targeting tumor-draining lymph node for promoting T cell activation. J Control Release. 2021;333:328-38.
242. Liu Y, Dong W, Ma Y, et al. Nanomedicines with high drug availability and drug sensitivity overcome hypoxia-associated drug resistance. Biomaterials. 2023;294:122023.
243. Li Y, Lu A, Long M, Cui L, Chen Z, Zhu L. Nitroimidazole derivative incorporated liposomes for hypoxia-triggered drug delivery and enhanced therapeutic efficacy in patient-derived tumor xenografts. Acta Biomater. 2019;83:334-48.
244. Thambiraj S, Shruthi S, Vijayalakshmi R, Ravi Shankaran D. Evaluation of cytotoxic activity of docetaxel loaded gold nanoparticles for lung cancer drug delivery. Cancer Treat Res Commun. 2019;21:100157.
245. Ruiz-Hernández E, Baeza A, Vallet-Regí M. Smart drug delivery through DNA/magnetic nanoparticle gates. ACS Nano. 2011;5:1259-66.
246. Wagner J, Gößl D, Ustyanovska N, et al. Mesoporous silica nanoparticles as pH-responsive carrier for the immune-activating drug resiquimod enhance the local immune response in mice. ACS Nano. 2021;15:4450-66.
247. Vaghasiya K, Ray E, Sharma A, Katare OP, Verma RK. Matrix metalloproteinase-responsive mesoporous silica nanoparticles cloaked with cleavable protein for “self-actuating” on-demand controlled drug delivery for cancer therapy. ACS Appl Bio Mater. 2020;3:4987-99.
Cite This Article

How to Cite
Download Citation
Export Citation File:
Type of Import
Tips on Downloading Citation
Citation Manager File Format
Type of Import
Direct Import: When the Direct Import option is selected (the default state), a dialogue box will give you the option to Save or Open the downloaded citation data. Choosing Open will either launch your citation manager or give you a choice of applications with which to use the metadata. The Save option saves the file locally for later use.
Indirect Import: When the Indirect Import option is selected, the metadata is displayed and may be copied and pasted as needed.
About This Article
Copyright
Data & Comments
Data
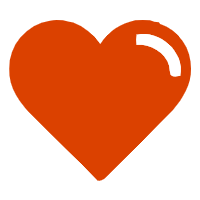
Comments
Comments must be written in English. Spam, offensive content, impersonation, and private information will not be permitted. If any comment is reported and identified as inappropriate content by OAE staff, the comment will be removed without notice. If you have any queries or need any help, please contact us at [email protected].