Non-coding RNA and drug resistance in head and neck cancer
Abstract
Head and neck cancer (HNC) is ranked as the sixth most common malignant tumor, and the overall survival rate with current treatment options remains concerning, primarily due to drug resistance that develops following antitumor therapy. Recent studies indicate that non-coding RNAs play a crucial role in drug resistance among HNC patients. This article systematically reviews the current research landscape, explores novel targets and treatment strategies related to non-coding RNAs and HNC resistance, raises some unresolved issues, and discusses five promising research directions in this field: ferroptosis, nanomedicine, exosomes, proteolysis-targeting chimeras (PROTACs), and artificial intelligence. We hope that our work will contribute to advancing research on overcoming HNC resistance through the regulation of non-coding RNAs.
Keywords
INTRODUCTION
Head and neck cancer (HNC) ranks as the sixth most prevalent malignant neoplasm, presenting significant challenges in terms of treatment[1]. It affects various anatomical sites including the lips, oral cavity, pharynx, larynx, nose, salivary glands, and thyroid. Squamous cell carcinoma (SCC) and its variants constitute more than 90% of the histopathological types[2]. Major risk factors associated with HNC include tobacco and alcohol use, as well as infections with human papillomavirus (HPV) and Epstein-Barr virus (EBV)[3]. Globally, the incidence of HNC has been gradually declining, primarily attributed to the reduction in tobacco use and lifestyle modifications[4,5].
Treatment decisions are guided by the precise location, stage, and pathologic characteristics of the disease. For around 30%-40% of patients diagnosed with early-stage disease (Stage I or II), the typical recommendation involves a single treatment modality such as surgery or radiotherapy. Conversely, roughly 60% of patients presenting with locally or regionally advanced disease upon diagnosis typically receive a multidisciplinary approach encompassing a combination of treatments. These may involve surgery, radiotherapy, chemotherapy, and immunotherapy, as well as additional measures such as nutritional support, psychological counseling, supportive care, and rehabilitation[2]. Various studies have indicated that the overall 5-year survival rate for individuals with HNC falls below 50%, underscoring the persisting challenges in current treatment outcomes[2,6]. The inadequate response of patients to antitumor therapy, and in some cases, the development of drug resistance, contribute to unsatisfactory clinical outcomes and stand as significant factors leading to mortality[7].
Unfortunately, the key determinants underlying this resistance phenomenon remain largely elusive. Advances in molecular biology and gene sequencing have unveiled that approximately 98% of human DNA is designated non-protein coding[8]. Non-coding RNAs constitute a diverse group of RNA transcripts that lack protein-coding potential. Significant subtypes include microRNAs (miRNAs), circular RNAs (circRNAs), and long non-coding RNAs (lncRNAs)[9]. Emerging research has revealed the potential of non-coding RNAs to modulate multiple facets of cellular functions, encompassing growth, proliferation, differentiation, development, metabolism, infection, immunity, cell death, organelle biogenesis, messenger signaling, DNA repair, and self-renewal[10-12]. Moreover, non-coding RNAs exhibit close associations with various common diseases, particularly cancer[9,13-15]. Additionally, non-coding RNAs function as widespread regulators of various cancer-related characteristics, including proliferation, apoptosis, invasion, metastasis, and genomic instability, thus playing a crucial role in mediating resistance to different cancer therapies[16,17]. Consequently, gaining a comprehensive understanding of the mechanisms underlying non-coding RNAs and drug resistance holds significant importance in the context of HNC treatment. In addition, the research on overcoming tumor drug resistance through the regulation of non-coding RNAs offers many advantages[16,18-28] [Table 1].
Advantages of targeting non-coding RNAs to overcome tumor drug resistance
Aspect | Summary | Ref. |
Complexity of regulatory networks | ncRNAs form intricate regulatory networks that influence various biological processes, including gene expression, signal transduction, and the cell cycle. Targeting these nodes can impact tumor growth and drug resistance | [18] |
Specific role | ncRNAs may be uniquely expressed in tumor cells or play critical roles in tumor development and drug resistance, enabling the development of targeted therapies | [19] |
Multi-target intervention | ncRNAs can affect multiple signaling pathways and biological processes simultaneously, making them suitable for multi-target interventions to address multidrug resistance | [20] |
Drug tolerance | ncRNAs may have a lower risk of tolerance development compared to protein targets due to their distinct regulatory mechanisms | [21] |
Diverse treatment strategies | ncRNAs can act as direct drug targets, carriers, or therapeutic agents, offering a range of treatment strategies | [16] |
New drug discovery | Research on ncRNAs provides new molecular targets for drug development, aiding in the discovery of novel antitumor agents | [22] |
Personalized medicine | ncRNA-based biomarkers can guide personalized treatment plans, helping physicians select the most suitable therapy for individual patients | [23-25] |
Combination therapy | ncRNAs can be combined with other therapies (e.g., chemotherapy, radiotherapy, immunotherapy) to improve efficacy and reduce drug resistance | [26] |
Treatment monitoring and evaluation | ncRNA expression levels can be used as biomarkers to monitor treatment efficacy, tumor response, and drug resistance development | [27] |
Gene editing applications | Gene editing technologies like CRISPR/Cas9 offer tools for targeting ncRNAs, enabling precise regulation of their expression and influencing drug resistance | [28] |
This review aims to provide a comprehensive summary of the intricate relationship between non-coding RNAs and drug resistance in HNC. It delves into the various types of non-coding RNAs and their potential roles in mediating resistance to different therapeutic approaches utilized in HNC treatment. Through an analysis of the current literature, this review seeks to enhance our understanding of the mechanisms underlying drug resistance in HNC and identify potential avenues for improving treatment outcomes.
miRNAs IN HNC CELL DRUG RESISTANCE
miRNAs, approximately 22 nucleotides in length, constitute a class of endogenous non-coding RNAs. These molecules regulate diverse biological processes, including cell proliferation, differentiation, and apoptosis, through specific binding to the 3’-untranslated region (3’-UTR) of target gene mRNAs, thereby inducing mRNA degradation or inhibiting translation[29-31]. Numerous studies have demonstrated the involvement of miRNA dysregulation in the development and chemoresistance of HNC[32-34].
miRNAs dysregulation is closely related to chemoresistance in HNC
Research indicates a close association between miRNA dysregulation and chemoresistance in HNC. Zhang et al. observed the downregulation of miR-216a-5p and ZEB1 in laryngeal squamous cell carcinoma (LSCC) tissues. Moreover, they demonstrated that overexpression of miR-216a-5p could reverse the malignant phenotype and cisplatin (CDDP) resistance of LSCC cells by targeting ZEB1[35]. Gao et al. identified that circ_0109291 promoted CDDP resistance in oral squamous cell carcinoma (OSCC) cells. They elucidated its mechanism by showing that circ_0109291 sponged miR-188-3p, leading to upregulation of ABCB1 expression[36]. Cao et al. revealed that HOTAIR induced CDDP resistance in nasopharyngeal carcinoma (NPC) by sponging miR-106a-5p, consequently upregulating SOX4 expression[37]. The miR-200 family constitutes a pivotal group of miRNAs implicated in the regulation of epithelial-mesenchymal transition (EMT). Their downregulation is closely linked to chemoresistance in HNC. For instance, reduced expression of Let-7, miR-200, and miR-203 correlates with docetaxel resistance in OSCC[38-42]. Moreover, the miR-200c/c-myc negative feedback loop orchestrates EMT, stemness, and chemoresistance in NPC[43,44]. Additionally, dysregulation of miR-138, miR-222, miR-101, miR-23a, miR-214, and various other miRNAs has been associated with chemoresistance in HNC[45-47]. In summary, the dysregulation of specific miRNAs plays a key role in the acquired chemoresistance of HNC.
miRNAs affect chemoresistance by regulating autophagy signaling pathways
Autophagy and miRNAs are intricately linked to chemoresistance in HNC. H19 induces CDDP resistance in LSCC cells by upregulating the expression of autophagy-related proteins Atg5 and Beclin1 through the miR-107/HMGB1 axis[48]. MiR-155 inhibitor-loaded exosomes reverse CDDP resistance in OSCC by inducing autophagy through the upregulation of FOXO3a[49]. Yang et al. found that upregulated miR-214 expression could inhibit autophagy in OSCC cells by targeting autophagy-related genes ULK1 and ATG5, consequently enhancing chemosensitivity[50]. CircAP1M2 promotes CDDP resistance and autophagy in OSCC by inhibiting miR-1249-3p to upregulate ATG9A expression[51]. MiR-1278 inhibits autophagy and CDDP resistance in NPC cells by targeting ATG2B[52]. Therefore, targeting autophagy pathways through the regulation of miRNAs may enhance the therapeutic sensitivity of HNC.
miRNAs affect chemoresistance by regulating the cell cycle and metabolism
miRNAs can also modulate the sensitivity of tumor cells to treatment by regulating energy metabolism and cell cycle. Fan et al. discovered that downregulation of mitochondrial miR-2392 expression could lead to impaired mitochondrial oxidative phosphorylation by inhibiting MT-CO3 translation, resulting in a shift of glucose metabolism from oxidative phosphorylation to anaerobic glycolysis, ultimately causing CDDP resistance in tongue squamous cell carcinoma (TSCC)[53]. Chen et al. discovered that miR-5787 contributes to CDDP resistance in TSCC by reprogramming glucose metabolism via inhibiting the translation of
miRNAs affect chemoresistance via exosome-mediated mechanisms
Exosomes, as an important mediator of intercellular communication, can carry functional molecules such as miRNAs to mediate information exchange between tumor cells and the microenvironment, playing a key role in tumor progression and chemoresistance[59-61]. Qin et al. found that CAF-derived exosomal miR-196a could confer CDDP resistance to head and neck squamous carcinoma cells by targeting CDKN1B and ING5[62]. Li et al. discovered that exosomal miR-106a-5p derived from CDDP-resistant cells could promote NPC cell proliferation and suppress apoptosis by targeting ARNT2 and activating AKT phosphorylation, thereby regulating tumorigenesis[63]. This study revealed that tumor cells could remodel the tumor microenvironment through exosomal miRNAs to acquire a chemoresistant phenotype.
miRNAs affect chemoresistance by regulating cancer stem cells
Cancer stem cells play a key role in tumor development, metastasis, and chemoresistance[64-66]. MiRNAs are involved in the chemoresistance process of HNC by regulating cancer stemness. Recent studies have shown that miRNA-485-5p can regulate the stemness and chemotherapy resistance of OSCC by targeting keratin 17 (KRT17)[67]. MiR-21-3p overexpression maintained the stemness of this subpopulation. Lin et al. discovered that activation of the miR-371/372/373 cluster could enhance the tumorigenicity and drug tolerance of OSCC cells[68]. Cai et al. found that EBV-encoded miR-BART7-3p enhanced the stemness and chemotherapy resistance of NPC by inhibiting SMAD7 to activate the TGF-β pathway[69].
miRNAs affect chemoresistance by regulating other signaling pathways
In addition, miRNAs are also involved in chemoresistance of HNC by regulating multiple signaling pathways. Zhang et al. found that miR-205-5p could induce EMT and CDDP resistance in NPC by targeting PTEN to activate the PI3K/Akt pathway[70]. Gu et al. discovered that miR-552 could promote the proliferation and metastasis of laryngeal cancer cells by targeting p53[71]. Sheng et al. discovered that miR-21 enhances proliferation, apoptosis inhibition, and CDDP resistance in head and neck squamous cell carcinoma (HNSCC) through the PTEN/PI3K/AKT pathway[72]. Wu et al. found that the miR-577/EIF5A2 axis suppresses the proliferation of CDDP-resistant NPC by blocking the TGF-β signaling pathway and inhibiting EMT[73]. In a study by Chen et al., miR-132 was shown to inhibit proliferation and invasion while enhancing CDDP chemosensitivity in OSCC cells via the TGF-β1/Smad2/3 signaling pathway[74]. Furthermore, miRNA-296-5p was found to increase the sensitivity of NPC cells to CDDP by targeting and suppressing the STAT3/KLF4 signaling axis[75]. These results highlight specific miRNAs play a key role in the acquired chemoresistance of HNC by fine-tuning multiple signaling pathways.
miRNAs regulate chemoresistance in HNC by competitively binding to upstream lncRNAs
miRNAs also regulate chemoresistance in HNC by competitively binding to upstream lncRNAs. FOXD1 promotes OSCC chemoresistance by upregulating LPP expression via sponging miR-1252-5p and miR-3148 through CYTOR[76]. PVT1 promotes cetuximab resistance in head and neck squamous carcinoma by inhibiting miR-124-3p[77]. KCNQ1OT1 promotes CDDP resistance in NPC through the miR-454/USP47 axis[78]. NEAT1 promotes CDDP resistance in thyroid cancer(TC) through the miR-9-5p/SPAG9 axis[79]. CircRNAs also regulate chemoresistance in HNC by sponging miRNAs, such as circCRIM1 promoting docetaxel resistance in NPC through the miR-422a/FOXQ1 axis[80].
Furthermore, miRNAs can also be involved in the chemoresistance of HNC by affecting drug resistance-related enzymes, membrane transporters, angiogenesis, and other factors. Yuan et al. found that LINC-PINT could reverse laryngeal cancer stemness and chemotherapy resistance through the miR-425-5p/PTCH1/SHH axis[81]. MiR-340 reverses multidrug resistance in NPC by inhibiting P-gp and BCRP[82]. Docetaxel can induce IL-8 secretion in OSCC cells, thereby promoting angiogenesis.
In recent years, strategies targeting miRNAs to reverse chemoresistance have received widespread attention. Li et al. found that miR-101-3p mimics could inhibit the proliferation and CDDP resistance of NPC cells through ZIC5[47]. Song et al. discovered that miR-619-5p inhibitors could enhance the CDDP sensitivity of OSCC cells by activating ATXN3[83] [Table 2 and Figure 1]. Although miRNAs have shown good antitumor and chemoresistance reversal effects at the animal level, their clinical application still faces many challenges, such as the construction of miRNA delivery systems, in vivo stability, and off-target effects[84-87]. In addition, the role of circRNAs and lncRNAs as endogenous miRNA sponges in reversing chemoresistance requires further in-depth research.
Figure 1. Overview of the main molecular mechanisms of microRNAs in HNC drug resistance. HNC: Head and neck cancer.
HNC cell drug resistance-related microRNAs
Tumor type | MiRNAs | Cell line | Expression level | Target | Functions | Corresponding drugs | Ref. |
NPC | miR-106a-5p | C666-1, CNE2 | Down | SOX4 | Enhance DDP resistance in NPC cells | CDDP | [37] |
NPC | miR-200c | CNE1, CNE2, 5-8F | Up | c-Myc | Promising approach to overcome the oncogenic role of c-Myc in NPC | Cisplatin | [43] |
NPC | miR-101-3p | HNE-1/DDP, C666-1/DDP | Up | SOX2 | Inhibit cisplatin resistance through miR-101-3p/SOX2/ZIC5 axis | CDDP | [47] |
NPC | miR-1278 | CNE-1, CNE-2, C666-1, 5-8F, HONE-1 | Up | ATG2B | Sensitize NPC cells to DDP and reduce autophagy | CDDP | [52] |
NPC | miR-106a-5p | CNE1 | Down | ARNT2 | Improve recipient cell proliferation, metastasis, and chemoresistance | CDDP | [63] |
NPC | miR-205-5p | HNE1/DDP | Down | PTEN | Restrain EMT progression of HNE1/DDP cells | CDDP | [70] |
NPC | miR-454 | 5-8F, SUNE-1 | Down | USP47 | Suppress NPC cell viability and DDP resistance | CDDP | [78] |
NPC | miR-422a | S18, S26 | Down | FOXQ1 | Promote NPC cell metastasis and EMT | CDDP | [80] |
NPC | miR-181a | C666-1, SUNE1 | Up | KDM5C | Delay NPC cell progression | / | [82] |
LSCC | miR-216a-5p | Tu-686, SNU899, SNU46, Tu-177 | Up | ZEB1 | Downregulate the cell proliferation and migration and invasive ability | / | [35] |
LSCC | miR-107 | TU-177, AMC-HN-8 | Down | HMGB1 | Increase cisplatin resistance | CDDP | [48] |
LSCC | miR-425-5p | Hep-2 | Up | PTCH1 | Regulate laryngeal carcinoma cells through miR‐425‐5p/PTCH1 | CDDP | [81] |
OSCC | miR-188-3p | SCC-4, SCC-9, CAL-27, UM1, UM2 | Down | ABCB1 | Promote cisplatin resistance of Oral Squamous Cell Carcinoma | CDDP | [36] |
OSCC | miR-155 | UPCI-SCC-131, UPCI-SCC-131R | Down | FOXO3a | Suppress the stem-cell-like property and drug efflux transporter protein expression | CDDP | [49] |
OSCC | miR-1249-3p | CAL27, SCC15 | Down | ATG9A | Promote autophagy and induce cisplatin resistance | CDDP | [51] |
OSCC | miR-34 | OECM-1/PTX | Up | p53 | Increase DNA damage and apoptosis in a p53-depended manner | Paclitaxel | [55] |
OSCC | miR-494 | HSC3, HSC4 | Down | NQO1 | Induce drug resistance and increase stemness | CDDP | [56] |
OSCC | miR-371/372/373 | SAS subclones | Up | AKT, β-catenin and Src | Increase both oncogenicity and drug resistance | CDDP | [68] |
OSCC | miR-1252-5p | CAL-27 and SCC4 | Down | FOXD1 | Upregulate LPP expression | CDDP | [76] |
OSCC | miR-3148 | CAL-27 and SCC4 | Down | FOXD1 | Upregulate LPP expression | CDDP | [76] |
OSCC | miR-619-5p | HN6 and CAL27 | Up | ATXN3 | Inhibit proliferation and arrest cell cycle progression | CDDP | [84] |
TC | miR-9-5p | Nthy-ori 3-1, SW1736, 8505C | Up | SPAG9 | Sensitize ATC cells to DDP | CDDP | [79] |
TSCC | miR-200c | HSC-3 | Down | TUBB3 and PPP2R1B | Increase resistance to DTX, migration, and invasion and decrease apoptosis | Paclitaxel | [38] |
TSCC | miR-214 | CAL-27 and CP-H203 | Up | ULK1 | Antagonize antitumor effect | CDDP, paclitaxel | [50] |
TSCC | miR-2392 | CAL-27 and SCC-9 | Up | AGO2 | Inhibit apoptosis and cisplatin sensitivity | CDDP | [53] |
TSCC | miR-5787 | Cal27 | Down | MT-CO3 | Inhibit the translation of MT-CO3 to regulate cisplatin resistance | CDDP | [54] |
LONG NON-CODING RNAs IN HNC CELL DRUG RESISTANCE
LncRNAs are extensively expressed and have specific interactions with DNA, RNA, and proteins, allowing them to regulate chromatin function, modulate the assembly and function of membraneless nucleosomes, influence the stability and translation of cytoplasmic mRNA, and participate in the regulation of signaling pathways[88,89]. Moreover, lncRNAs play a crucial role in regulating processes such as apoptosis, drug efflux, drug metabolism, DNA repair, EMT, autophagy, and ferroptosis. Consequently, they emerge as pivotal mediators of tumor drug resistance[90-93]. In recent years, there has been a notable increase in the number of lncRNAs associated with cancer development and progression. These lncRNAs can be explored and investigated in meticulously curated databases like Lnc2Cancer3.0[94] or Cancer LncRNA Census[95].
CDDP resistance
CDDP stands as a highly extensively employed drug in the treatment of diverse solid tumors[96]. Its principal mode of action involves disrupting DNA repair mechanisms, leading to DNA damage and consequently triggering apoptosis in cancer cells[97,98]. Nonetheless, drug resistance poses an inherent challenge in the clinical utilization of CDDP, primarily stemming from three molecular mechanisms: heightened DNA repair, modified cellular accumulation, and augmented drug inactivation[99].In 2014, Galluzzi et al. Systematically categorized CDDP resistance into four distinct stages: Pre-Target, On-Target, Post-Target, and Off-Target[100]. In recent years, the advancement of molecular biology and multi-omics approaches has unveiled additional molecules and mechanisms associated with CDDP resistance in non-NHC. These include epigenetic mechanisms, as well as regulators such as HNRNPU, CHD4, TRPV1, MAFG-AS, and MAST1, among others[101-106]. Furthermore, a study demonstrated that in non-small cell lung cancer, MEK inhibitors successfully surmounted resistance to combination immunotherapy with CDDP by inducing CXCL10 expression in cancer cells[107].
lncRNAs mediate drug resistance of NPC
NPC represents an EBV-associated malignancy that is particularly common in southern China, Southeast Asia, and North Africa. The age-standardized incidence rate in these regions ranges from 4 to 25 cases per 100,000 individuals, as reported by GLOBOCAN[5,108]. Patients diagnosed with NPC generally exhibit a favorable prognosis, with a minimum 5-year survival rate of 80% in cases of locally advanced NPC. However, the 5-year survival rate drops to approximately 20% in recurrent or distant metastatic NPC[109,110]. The study found that CDDP-resistant NPC exhibits a high expression of lncRNA TINCR, which contributes to the development of chemoresistance via the TINCR/ACLY/PADI1/MAPK/MMP2/9 axis[111]. Another study identified elevated expression of LncRNA NEAT1 in NPC cells resistant to histone deacetylase inhibitors (HDACis). This upregulation is mediated by the NEAT1/miR-129/Bcl-2 axis, which plays a role in NPC's resistance to HDACis[112]. Consequently, an epigenetic therapeutic approach involving the use of HDACis in combination with other targeted agents holds promise as a novel strategy for future NPC treatment[113,114].
lncRNAs mediate drug resistance of HNC
The majority of HNCs originate from the mucosal epithelium of the oral cavity, pharynx, and larynx, making them the most prevalent malignant tumors in the head and neck region[115]. In head and neck squamous cell carcinoma (HNSCC), STAT3 promotes the transcription of lncRNA HOTAIR and its interaction with pEZH2-S21, which contributes to resistance against both CDDP and cetuximab[116]. Another study demonstrated that VN1R5 upregulates lncRNA POP1-1, leading to the promotion of CDDP resistance in HNSCC through its interaction with MCM5[117]. LSCC represents a highly prevalent subtype of laryngeal cancer, ranking second in incidence among respiratory tumors. Chemotherapy is regarded as the established first-line treatment for patients with advanced LSCC[118]. A study reported that the activation of the STAT3 signaling pathway by lncRNA FOXD2-AS1 enhances CDDP resistance in LSCC[119]. OSCC is the prevailing malignant tumor of the oral mucosa, with 57.5% of global oral cancer cases being reported in Asia, particularly in India[120]. FOXD1 upregulates the expression of lncRNA CYTOR, promoting EMT and conferring CDDP resistance in OSCC[76]. Additionally, it has been reported that in OSCC, HOXA11-AS upregulates the expression of NQO1 by sponging microRNA-494 while downregulating the expression of NQO2. This regulatory mechanism promotes tumor progression and contributes to drug resistance[56]. TSCC is characterized by a significant upregulation in the expression of miRNA processing-related lncRNA (MPRL), which disrupts pre-miRNA processing. This disruption promotes mitochondrial fission and enhances CDDP chemosensitivity in TSCC[121]. TC is a prevalent endocrine malignancy, comprising approximately 1% of all malignant tumors. Moreover, its incidence is consistently rising on a global scale[122]. Papillary thyroid cancer (PTC) is the most common histological subtype of TC, accounting for 89.1% of cases. Studies have shown that the LncRNA Glycolysis-Associated Regulator of LDHA post-transcriptional modification (GLTC) is significantly upregulated in PTC tissues and correlates with more extensive distant metastasis, increased tumor size, and poorer prognosis. GLTC facilitates the succinylation-dependent activation of LDHA, thus promoting resistance to radioiodine therapy. These findings provide a theoretical foundation for considering the GLTC-LDHA pathway a potential target for therapeutic intervention in PTC[123].
Several lncRNAs have been found to have close associations with the malignant biology of tumors. In LSCC, HCP5 exhibits high expression levels. The knockdown of HCP5 has been shown to inhibit malignant biological functions through the regulation of miR-216a-5p/ZEB1 signaling pathway[35]. In OSCC,
Figure 2. Overview of the main molecular mechanisms of lncRNAs in HNC drug resistance. HNC: Head and neck cancer.
HNC cell drug resistance-related lncRNAs
Tumor type | lncRNA | Cell line | Expression level | Target | Functions | Corresponding drugs | Ref. |
NPC | TINCR | NP69 N2Tert CNE-1, HK-1 | Up | ACLY | Activate TINCR-ACLY-PADI1-MAPK-MMP2/9 axis | CDDP | [111] |
NPC | NEAT1 | C666-1, CNE-1, CNE-2 | Up | miR-129 | Modulate the miR-129/Bcl-2 axis | HDACis | [112] |
HNSCC | HOTAI | SCC25, Cal27, UM1 | Up | EZH2 | Promote the growth of HNSCC cells. | CDDP/cetuximab | [116] |
HNSCC | POP1-1 | HN4, HN30 | Up | MCM5 | Facilitate the repair of DNA damage | CDDP | [117] |
LSCC | FOXD2-AS1 | Hep2, TU-212 | Up | STAT3 | Promote STAT3 transcriptional activity | CDDP | [119] |
OSCC | CYTOR | CAL-27, SCC4 | Up | ceRNA | Activate CYTOR/LPP axis | CDDP | [76] |
OSCC | HOXA11-AS | HSC3, HSC4 | Up | microRNA-494 | Facilitate tumor growth | Dicoumarol | [56] |
OSCC | EGFR-AS1 | primary cell cultures | Up | / | Resistance to EGFR TKIs | TKIs | [126] |
PTC | GLTC | BCPAP, TPC-1, KTC-1 | Up | LDHA | Resistance to RAI | RAI | [123] |
Substantial evidence supports the dependence of cellular homeostasis on lncRNAs[125,127]. However, despite a small fraction of the thousands of expressed lncRNAs potentially having functional roles in cancer cells, the extent of their involvement remains inadequately studied[128]. Further research is warranted to explore the role of lncRNAs in various aspects concerning tumor chemotherapy, targeted therapy, and immunotherapy, as well as their impact on the tumor microenvironment[129,130].
circRNAs IN HNC CELL DRUG RESISTANCE
The discovery of single-stranded covalently closed circular RNA dates back to 1976, with subsequent findings revealing their common presence in both viruses and mammals[131]. circRNAs exhibit a diverse range of functions, encompassing their role as protein scaffolds or miRNA sponges, and their capacity for translation into polypeptides[132]. The unique structure of circRNAs grants them a longer half-life compared to linear RNA and provides resistance against RNase R degradation[133]. Consequently, circRNAs have garnered significant attention as reliable diagnostic and prognostic biomarkers in cancer diagnosis, treatment, and prevention[13,16,134-137].
circRNAs mediate drug resistance of NPC
Patients with distant metastases of NPC exhibit specific overexpression of circIPO7, and its knockdown enhances sensitivity to CDDP treatment by inhibiting YBX1 nuclear localization[138]. Furthermore, studies have reported an association between aging and tumor metastasis and chemoresistance. Notably, circWDR37 facilitates the activation of PKR, leading to the initiation of SASP transcription through NF-κB, thereby promoting metastasis and chemoresistance in NPC[139]. A separate study demonstrated the role of circCRIM1 as a ceRNA in promoting metastasis and conferring docetaxel chemoresistance in NPC through the upregulation of FOXQ1. Additionally, the study successfully developed a prognostic model based on circCRIM1 expression and TMN staging to assess the risk of NPC metastasis[80].
circRNAs mediate drug resistance of HNC
CDDP resistance in HNC is strongly linked to autophagy, a process essential for maintaining protein homeostasis, organelle integrity, cellular homeostasis, cell viability, and the degradation/recirculation of various cellular components to the lysosome[140,141]. In OSCC, significant upregulation of circAP1M2 leads to the induction of autophagy-associated CDDP resistance via the miR-1249-3p/ATG9A axis[51]. In LSCC, circPARD3 hinders autophagy by serving as a sponge for miR-145-5p, which activates the PRKCI/Akt/mTOR pathway, thereby promoting tumor progression and contributing to CDDP resistance[142]. TC is characterized by the promotion of autophagy and increased CDDP resistance due to the regulatory effects of circEIF6 on the miR-144-3p/TGF-α axis[143] [Table 4 and Figure 3]. Interestingly, autophagy exhibits dynamic tumor-suppressive or tumor-promoting roles in diverse contexts and stages of cancer development[144]. Therefore, further research is needed to better define the specific roles of autophagy in various types and stages of cancer, and to gain a deeper understanding of how tumors rely on autophagy. Furthermore, several studies have demonstrated the reciprocal regulation between circRNAs and
Figure 3. Overview of the main molecular mechanisms of circRNAs in HNC drug resistance. HNC: Head and neck cancer.
HNC cell drug resistance-related circRNAs
Tumor type | circRNA | Cell line | Expression level | Target | Functions | Corresponding drugs | Ref. |
NPC | IPO7 | CNE2, HNE1, HONE1, SUNE1, HK1, C666-1 | Up | YBX1 | Promote cell migration, invasion, and cisplatin resistance | CDDP | [138] |
NPC | WDR37 | S18, S26 | Up | PKR | CCND1 | CDDP, gemcitabine | [139] |
NPC | CRIM1 | S18, S26 | Up | miR-422a | Promote metastasis and EMT | Docetaxel | [80] |
OSCC | AP1M2 | CAL27/CDDP | Up | miR-1249-3p | modulate miR-1249-3p-ATG9A axis | CDDP | [51] |
LSCC | PARD3 | Tu 177, HOK, FD-LSC-1 | Up | miR-145-5p w | Activate the Akt-mTOR axis | CDDP | [142] |
ATC | EIF6 | TPC1, BHT101 | Up | miR-144-3p | Regulate miR144-3p/TGF-α axis | CDDP | [143] |
FUTURE PERSPECTIVES
Based on our persistent dedication to this field and thorough literature research, we firmly assert that the following areas of research exhibit dynamism and merit sustained attention.
Ferroptosis
Ferroptosis, also known as iron-induced apoptosis, is a type of intracellular cell death that relies on iron and is distinguished by the excessive accumulation of reactive oxygen species (ROS) and lipid peroxidation. It is distinct from apoptosis, necrosis, and autophagy[150,151]. Numerous studies have reported that tumor cells can enhance their defense mechanisms against oxidative stress by inhibiting ferroptosis, ultimately promoting their survival and drug resistance[152,153]. Studies have demonstrated that in NPC, infection with EBV leads to the upregulation of GPX4 expression, resulting in the inhibition of ferroptosis. Consequently, the elevated levels of GPX4 contribute to the progression of NPC and its resistance to chemotherapy through the activation of the TAK1-JNK and IKK/NF-κB signaling pathways. Moreover, clearance of the EBV genome has been shown to enhance the sensitivity of NPC cells to ferroptosis[154]. Artesunate exhibits selective cytotoxicity against HNC cells. In specific cases of CDDP-resistant HNC, inhibiting the Nrf2-ARE pathway enhances the sensitivity of these cells to artesunate and reverses their resistance to ferroptosis[155,156]. Additional studies have further demonstrated that inhibiting GLRX5 renders CDDP-resistant HNC cells more susceptible to ferroptosis[157]. Furthermore, the upregulation of HMGA1 in ESCC serves as a crucial factor responsible for CDDP resistance by suppressing ferroptosis. This effect is achieved through HMGA1’s role in maintaining intracellular redox homeostasis via its assistance to ATF4 in activating SLC7A11 transcription. Inhibition of HMGA1 has been shown to enhance the sensitivity of ESCC to ferroptosis[158]. Multiple studies have now established that regulating ferroptosis can impact the effectiveness of cancer treatment and potentially overcome resistance to chemotherapy, targeted therapy, and immunotherapy[159-164]. Several non-coding RNAs, including miR-324-3p, miR-375, miR-144-3p,
Nanodrugs
CDDP, carboplatin, and oxaliplatin are commonly employed in tumor therapy. Nevertheless, their clinical utility is severely restricted due to the side effects associated with platinum drugs, including poor selectivity, high systemic toxicity, and drug resistance[169]. A novel CDDP nanocarrier system with dual targeting properties has been developed. This system selectively attaches to the A54 receptor, which is highly expressed on the cell surface of hepatocellular carcinoma (HCC) cells. Additionally, it utilizes the drug-resistance gene NOR1 shRNA as a piggyback mechanism to enable precise tumor-targeted therapy and overcome drug resistance[170]. Numerous studies have also highlighted the effectiveness of platinum nanocarriers in reducing systemic toxicity and overcoming drug resistance[171-174]. Currently, there is considerable research focus on strategies involving nanomedicines for regulating ferroptosis, as well as nanomedicines utilizing non-coding RNAs as carriers to target tumors[85,175-179].
Exosomes
Exosomes are nanovesicles derived from cells, ranging in size from 30 to 150 nm. They are released when multivesicular bodies fuse with the cell surface and play a crucial role in intercellular communication by transporting nucleic acids, proteins, and lipids. Furthermore, exosomes can activate signaling pathways in target cells[180,181]. In recent years, there has been significant interest in the role of exosomes carrying non-coding RNA in tumor drug resistance[93,163,182-185]. Several studies have reported that intervention with RNA carried by exosomes can potentially overcome tumor chemoresistance[61,186,187]. For instance, CAFs emerge as crucial regulators of CDDP resistance in HNC. They achieve this by transporting functional miR-196a from CAFs to tumor cells through exosomes[62]. Although our understanding of the role of RNAs carried within exosomes in the mechanisms of drug resistance in HNC is still in the early stages, exosomal circRNAs have emerged as innovative genetic information carriers. These molecules enable communication between tumor cells and cells in the microenvironment, thereby regulating critical aspects of cancer progression. As a result, they contribute significantly to chemotherapeutic drug resistance across different types of cancer. Based on this, synthetic exosomal circRNA holds the potential to introduce new avenues for cancer therapy[188,189].
Proteolysis-targeting chimeras
Proteolysis-targeting chimeras (PROTACs) are emerging as promising therapeutic modalities for the degradation of disease-causing proteins. They are composed of a ligand that binds to a protein of interest (POI) and another ligand that recruits the E3 ubiquitin ligase. This recruitment induces chemical proximity between the POI and the E3 ligase, resulting in ubiquitination and subsequent degradation of the POI through the ubiquitin-proteasome system[190-193].PROTACs have been developed to target several proteins, including PD-L1, BTK, STAT3, EGFR, MEK1/2, VEGFR2, FLT-3, and SHP2[194]. These compounds offer advantages such as high enzymatic reaction efficiency, overall degradation of target proteins, and the ability to target small-molecule non-druggable proteins. Several studies have reported the successful inhibition of HNSCC growth and metastasis using PROTAC technology[195,196]. This finding highlights the importance of continued attention to PROTAC and related technologies due to their ability to specifically degrade oncogenic proteins or RNAs[194,197,198].
CONCLUSION
In summary, chemotherapy, targeted therapy, and immunotherapy are crucial components of integrated tumor therapy. However, drug resistance significantly limits the effectiveness of clinical drug therapy for HNC. Non-coding RNA dysregulation plays a critical role in the known mechanisms of drug resistance in HNC. It is essential to continually focus on new technologies and theories to address the challenges in HNC drug resistance research. This includes the construction or utilization of clinical databases similar to UK biobank[199], and integrating clinical information with multi-omics sequencing data. These approaches aim to establish a robust foundation for further research on integrative oncology treatment[200]. Additionally, leveraging ChatGPT automated development and analysis (ADA) technology to build machine learning models based on real clinical trial data[201] can facilitate efficient research on cancer precision treatment, prognosis, and drug resistance markers.
DECLARATIONS
Acknowledgments
We appreciate and acknowledge the support from Dr. Rensen Ran and Lei Chen for their guidance and assistance in drawing.
Authors’ contributions
Conceived the contents and structure: Yang S, Deng F, Yang X, Li A, Xia W, Gao C, Lei S
Wrote the original manuscript: Zhang Y, Peng Y, Lin B
Revised and improved the manuscript: Liao W, Zeng Q
All authors have read and agreed to the published version of the manuscript.
Financial support and sponsorship
Project of Administration of Traditional Chinese Medicine of Guangdong Province of China (No. 20231070).
Conflicts of interest
All authors declared that there are no conflicts of interest.
Ethical approval and consent to participate
Not applicable.
Consent for publication
Not applicable.
Copyright
© The Author(s) 2024.
REFERENCES
1. Li Q, Tie Y, Alu A, Ma X, Shi H. Targeted therapy for head and neck cancer: signaling pathways and clinical studies. Signal Transduct Target Ther 2023;8:31.
2. Pfister DG, Spencer S, Adelstein D, et al. Head and neck cancers, version 2.2020, NCCN clinical practice guidelines in oncology. J Natl Compr Canc Netw 2020;18:873-98.
5. Wong KCW, Hui EP, Lo KW, et al. Nasopharyngeal carcinoma: an evolving paradigm. Nat Rev Clin Oncol 2021;18:679-95.
6. Leemans CR, Braakhuis BJ, Brakenhoff RH. The molecular biology of head and neck cancer. Nat Rev Cancer 2011;11:9-22.
7. Dai F, Dai L, Zheng X, et al. Non-coding RNAs in drug resistance of head and neck cancers: a review. Biomed Pharmacother 2020;127:110231.
8. Romano G, Veneziano D, Acunzo M, Croce CM. Small non-coding RNA and cancer. Carcinogenesis 2017;38:485-91.
9. Nemeth K, Bayraktar R, Ferracin M, Calin GA. Non-coding RNAs in disease: from mechanisms to therapeutics. Nat Rev Genet 2024;25:211-32.
11. Kabekkodu SP, Shukla V, Varghese VK, D’Souza J, Chakrabarty S, Satyamoorthy K. Clustered miRNAs and their role in biological functions and diseases. Biol Rev Camb Philos Soc 2018;93:1955-86.
12. Ransohoff JD, Wei Y, Khavari PA. The functions and unique features of long intergenic non-coding RNA. Nat Rev Mol Cell Biol 2018;19:143-57.
13. Kristensen LS, Jakobsen T, Hager H, Kjems J. The emerging roles of circRNAs in cancer and oncology. Nat Rev Clin Oncol 2022;19:188-206.
14. Wen SY, Qadir J, Yang BB. Circular RNA translation: novel protein isoforms and clinical significance. Trends Mol Med 2022;28:405-20.
15. Williams M, Cheng YY, Phimmachanh M, Winata P, van Zandwijk N, Reid G. Tumour suppressor microRNAs contribute to drug resistance in malignant pleural mesothelioma by targeting anti-apoptotic pathways. Cancer Drug Resist 2019;2:1193-206.
16. Chen B, Dragomir MP, Yang C, Li Q, Horst D, Calin GA. Targeting non-coding RNAs to overcome cancer therapy resistance. Signal Transduct Target Ther 2022;7:121.
17. Zhang X, Xie K, Zhou H, et al. Role of non-coding RNAs and RNA modifiers in cancer therapy resistance. Mol Cancer 2020;19:47.
18. Mattick JS, Amaral PP, Carninci P, et al. Long non-coding RNAs: definitions, functions, challenges and recommendations. Nat Rev Mol Cell Biol 2023;24:430-47.
20. Yuan JH, Yang F, Wang F, et al. A long noncoding RNA activated by TGF-β promotes the invasion-metastasis cascade in hepatocellular carcinoma. Cancer Cell 2014;25:666-81.
21. Winkle M, El-Daly SM, Fabbri M, Calin GA. Noncoding RNA therapeutics - challenges and potential solutions. Nat Rev Drug Discov 2021;20:629-51.
24. Cavaliere AF, Perelli F, Zaami S, et al. Towards personalized medicine: non-coding RNAs and endometrial cancer. Healthcare 2021;9:965.
25. Piergentili R, Basile G, Nocella C, et al. Using ncRNAs as tools in cancer diagnosis and treatment-the way towards personalized medicine to improve patients’ health. Int J Mol Sci 2022;23:9353.
26. Rupaimoole R, Slack FJ. MicroRNA therapeutics: towards a new era for the management of cancer and other diseases. Nat Rev Drug Discov 2017;16:203-22.
27. Naorem LD, Prakash VS, Muthaiyan M, Venkatesan A. Comprehensive analysis of dysregulated lncRNAs and their competing endogenous RNA network in triple-negative breast cancer. Int J Biol Macromol 2020;145:429-36.
28. Mahato RK, Bhattacharya S, Khullar N, et al. Targeting long non-coding RNAs in cancer therapy using CRISPR-Cas9 technology: a novel paradigm for precision oncology. J Biotechnol 2024;379:98-119.
29. Kilikevicius A, Meister G, Corey DR. Reexamining assumptions about miRNA-guided gene silencing. Nucleic Acids Res 2022;50:617-34.
30. Shang R, Lee S, Senavirathne G, Lai EC. microRNAs in action: biogenesis, function and regulation. Nat Rev Genet 2023;24:816-33.
31. Agbu P, Carthew RW. MicroRNA-mediated regulation of glucose and lipid metabolism. Nat Rev Mol Cell Biol 2021;22:425-38.
32. He B, Zhao Z, Cai Q, et al. miRNA-based biomarkers, therapies, and resistance in Cancer. Int J Biol Sci 2020;16:2628-47.
33. Pan G, Liu Y, Shang L, Zhou F, Yang S. EMT-associated microRNAs and their roles in cancer stemness and drug resistance. Cancer Commun 2021;41:199-217.
34. Meng X, Lou QY, Yang WY, et al. The role of non-coding RNAs in drug resistance of oral squamous cell carcinoma and therapeutic potential. Cancer Commun 2021;41:981-1006.
35. Zhang S, Huangfu H, Zhao Q, Li Y, Wu L. Downregulation of long noncoding RNA HCP5/miR-216a-5p/ZEB1 axis inhibits the malignant biological function of laryngeal squamous cell carcinoma cells. Front Immunol 2022;13:1022677.
36. Gao F, Han J, Wang Y, Jia L, Luo W, Zeng Y. Circ_0109291 promotes cisplatin resistance of oral squamous cell carcinoma by sponging miR-188-3p to increase ABCB1 expression. Cancer Biother Radiopharm 2022;37:233-45.
37. Cao W, Sun Y, Liu L, et al. HOTAIR mediates cisplatin resistance in nasopharyngeal carcinoma by regulating miR-106a-5p/SOX4 axis. Bioengineered 2022;13:6567-78.
38. Cui J, Wang H, Zhang X, Sun X, Zhang J, Ma J. Exosomal miR-200c suppresses chemoresistance of docetaxel in tongue squamous cell carcinoma by suppressing TUBB3 and PPP2R1B. Aging 2020;12:6756-73.
39. Proença C, Freitas M, Ribeiro D, Rufino AT, Fernandes E, Ferreira de Oliveira JMP. The role of flavonoids in the regulation of epithelial-mesenchymal transition in cancer: a review on targeting signaling pathways and metastasis. Med Res Rev 2023;43:1878-945.
40. Garinet S, Didelot A, Denize T, et al. Clinical assessment of the miR-34, miR-200, ZEB1 and SNAIL EMT regulation hub underlines the differential prognostic value of EMT miRs to drive mesenchymal transition and prognosis in resected NSCLC. Br J Cancer 2021;125:1544-51.
41. Chien CS, Wang ML, Chu PY, et al. Lin28B/Let-7 regulates expression of Oct4 and Sox2 and reprograms oral squamous cell carcinoma cells to a stem-like state. Cancer Res 2015;75:2553-65.
42. Manikandan M, Deva Magendhra Rao AK, Arunkumar G, et al. Oral squamous cell carcinoma: microRNA expression profiling and integrative analyses for elucidation of tumourigenesis mechanism. Mol Cancer 2016;15:28.
43. Yang J, Wu SP, Wang WJ, et al. A novel miR-200c/c-myc negative regulatory feedback loop is essential to the EMT process, CSC biology and drug sensitivity in nasopharyngeal cancer. Exp Cell Res 2020;391:111817.
44. Deng X, Liu Z, Liu X, et al. miR-296-3p negatively regulated by nicotine stimulates cytoplasmic translocation of c-Myc via MK2 to suppress chemotherapy resistance. Mol Ther 2018;26:1066-81.
45. Huni KC, Cheung J, Sullivan M, Robison WT, Howard KM, Kingsley K. Chemotherapeutic drug resistance associated with differential miRNA expression of miR-375 and miR-27 among oral cancer cell lines. Int J Mol Sci 2023;24:1244.
46. Shaw P, Raymond G, Senthilnathan R, et al. Clinical theragnostic relationship between chemotherapeutic resistance, and sensitivity and miRNA expressions in head and neck cancers: a systematic review and meta-analysis protocol. Genes 2021;12:2029.
47. Li T, Zhang G, Li W, et al. MicroRNA-101-3p inhibits nasopharyngeal carcinoma cell proliferation and cisplatin resistance through ZIC5 down-regulation by targeting SOX2. Biol Chem 2023;404:961-75.
48. Chen L, Xu Z, Zhao J, et al. H19/miR-107/HMGB1 axis sensitizes laryngeal squamous cell carcinoma to cisplatin by suppressing autophagy in vitro and in vivo. Cell Biol Int 2021;45:674-85.
49. Sayyed AA, Gondaliya P, Mali M, et al. MiR-155 inhibitor-laden exosomes reverse resistance to cisplatin in a 3D tumor spheroid and xenograft model of oral cancer. Mol Pharm 2021;18:3010-25.
50. Yang Y, Sun X, Li M, Li L, Wang S, Zhu Y. miR-214 modulates the growth and migration of oral cancer before and after chemotherapy through mediating ULK1. J Immunol Res 2022;2022:4589182.
51. Wenhao R, Yali C, Shaoming L, Jingjing Z, Ling G, Keqian Z. circAP1M2 activates ATG9A-associated autophagy by inhibiting miR-1249-3p to promote cisplatin resistance in oral squamous cell carcinoma. J Cell Physiol 2023;238:2612-24.
52. Zhao Y, Wang P, Wu Q. miR-1278 sensitizes nasopharyngeal carcinoma cells to cisplatin and suppresses autophagy via targeting ATG2B. Mol Cell Probes 2020;53:101597.
53. Fan S, Tian T, Chen W, et al. Mitochondrial miRNA determines chemoresistance by reprogramming metabolism and regulating mitochondrial transcription. Cancer Res 2019;79:1069-84.
54. Chen W, Wang P, Lu Y, et al. Decreased expression of mitochondrial miR-5787 contributes to chemoresistance by reprogramming glucose metabolism and inhibiting MT-CO3 translation. Theranostics 2019;9:5739-54.
55. Mortezagholi B, Nasiri K, Movahed E, et al. MiR-34 by targeting p53 induces apoptosis and DNA damage in paclitaxel-resistant human oral squamous carcinoma cells. Chem Biol Drug Des 2023;102:285-91.
56. Nakashima C, Fujiwara-Tani R, Mori S, et al. An axis between the long non-coding RNA HOXA11-AS and NQOs enhances metastatic ability in oral squamous cell carcinoma. Int J Mol Sci 2022;23:10704.
57. Kang SH, Oh SY, Lee KY, et al. Differential effect of cancer-associated fibroblast-derived extracellular vesicles on cisplatin resistance in oral squamous cell carcinoma via miR-876-3p. Theranostics 2024;14:460-79.
58. Li P, Yang Y, Liu H, et al. MiR-194 functions as a tumor suppressor in laryngeal squamous cell carcinoma by targeting Wee1. J Hematol Oncol 2017;10:32.
59. Luo X, Li Y, Hua Z, et al. Exosomes-mediated tumor metastasis through reshaping tumor microenvironment and distant niche. J Control Release 2023;353:327-36.
60. Dai J, Su Y, Zhong S, et al. Exosomes: key players in cancer and potential therapeutic strategy. Signal Transduct Target Ther 2020;5:145.
61. Huang C, Zhou Y, Feng X, Wang J, Li Y, Yao X. Delivery of engineered primary tumor-derived exosomes effectively suppressed the colorectal cancer chemoresistance and liver metastasis. ACS Nano 2023;17:10313-26.
62. Qin X, Guo H, Wang X, et al. Exosomal miR-196a derived from cancer-associated fibroblasts confers cisplatin resistance in head and neck cancer through targeting CDKN1B and ING5. Genome Biol 2019;20:12.
63. Li J, Hu C, Chao H, et al. Exosomal transfer of miR-106a-5p contributes to cisplatin resistance and tumorigenesis in nasopharyngeal carcinoma. J Cell Mol Med 2021;25:9183-98.
64. Babaei G, Aziz SG, Jaghi NZZ. EMT, cancer stem cells and autophagy; The three main axes of metastasis. Biomed Pharmacother 2021;133:110909.
65. Rao X, Zhang C, Luo H, et al. Targeting gastric cancer stem cells to enhance treatment response. Cells 2022;11:2828.
66. Lu H, Ju DD, Yang GD, et al. Targeting cancer stem cell signature gene SMOC-2 Overcomes chemoresistance and inhibits cell proliferation of endometrial carcinoma. EBioMedicine 2019;40:276-89.
67. Jang TH, Huang WC, Tung SL, et al. MicroRNA-485-5p targets keratin 17 to regulate oral cancer stemness and chemoresistance via the integrin/FAK/Src/ERK/β-catenin pathway. J Biomed Sci 2022;29:42.
68. Lin SC, Wu HL, Yeh LY, Yang CC, Kao SY, Chang KW. Activation of the miR-371/372/373 miRNA cluster enhances oncogenicity and drug resistance in oral carcinoma cells. Int J Mol Sci 2020;21:9442.
69. Cai L, Long Y, Chong T, et al. EBV-miR-BART7-3p imposes stemness in nasopharyngeal carcinoma cells by suppressing SMAD7. Front Genet 2019;10:939.
70. Zhang P, Lu X, Shi Z, et al. miR-205-5p regulates epithelial-mesenchymal transition by targeting PTEN via PI3K/AKT signaling pathway in cisplatin-resistant nasopharyngeal carcinoma cells. Gene 2019;710:103-13.
71. Gu J, Han T, Sun L, Yan AH, Jiang XJ. miR-552 promotes laryngocarcinoma cells proliferation and metastasis by targeting p53 pathway. Cell Cycle 2020;19:1012-21.
72. Sheng S, Su W, Mao D, et al. MicroRNA-21 induces cisplatin resistance in head and neck squamous cell carcinoma. PLoS One 2022;17:e0267017.
73. Wu R, Zhong Q, Liu H, Liu S. MicroRNA-577/EIF5A2 axis suppressed the proliferation of DDP-resistant nasopharyngeal carcinoma cells by blocking TGF-β signaling pathway. Chem Biol Drug Des 2023;102:815-27.
74. Chen L, Zhu Q, Lu L, Liu Y. MiR-132 inhibits migration and invasion and increases chemosensitivity of cisplatin-resistant oral squamous cell carcinoma cells via targeting TGF-β1. Bioengineered 2020;11:91-102.
75. Luo HQ, Wang Y, Ren J, et al. MiRNA-296-5p promotes the sensitivity of nasopharyngeal carcinoma cells to cisplatin via targeted inhibition of STAT3/KLF4 signaling axis. Sci Rep 2024;14:6681.
76. Chen S, Yang M, Wang C, et al. Forkhead box D1 promotes EMT and chemoresistance by upregulating lncRNA CYTOR in oral squamous cell carcinoma. Cancer Lett 2021;503:43-53.
77. Yang S, Yuan ZJ, Zhu YH, Chen X, Wang W. lncRNA PVT1 promotes cetuximab resistance of head and neck squamous cell carcinoma cells by inhibiting miR-124-3p. Head Neck 2021;43:2712-23.
78. Yuan F, Lou Z, Zhou Z, Yan X. [Retracted] Long non‑coding RNA KCNQ1OT1 promotes nasopharyngeal carcinoma cell cisplatin resistance via the miR‑454/USP47 axis. Int J Mol Med 2024;53:31.
79. Yan P, Su Z, Zhang Z, Gao T. LncRNA NEAT1 enhances the resistance of anaplastic thyroid carcinoma cells to cisplatin by sponging miR‑9‑5p and regulating SPAG9 expression. Int J Oncol 2019;55:988-1002.
80. Hong X, Liu N, Liang Y, et al. Circular RNA CRIM1 functions as a ceRNA to promote nasopharyngeal carcinoma metastasis and docetaxel chemoresistance through upregulating FOXQ1. Mol Cancer 2020;19:33.
81. Yuan Z, Xiu C, Liu D, et al. Long noncoding RNA LINC-PINT regulates laryngeal carcinoma cell stemness and chemoresistance through miR-425-5p/PTCH1/SHH axis. J Cell Physiol 2019;234:23111-22.
82. Liu J, Zhu M, Tang Q. Human umbilical cord mesenchymal stem cells-derived exosomal microRNA-181a retards nasopharyngeal carcinoma development by mediating KDM5C. J Cancer Res Clin Oncol 2021;147:2867-77.
83. Song A, Wu Y, Chu W, et al. Involvement of miR-619-5p in resistance to cisplatin by regulating ATXN3 in oral squamous cell carcinoma. Int J Biol Sci 2021;17:430-47.
84. Diener C, Keller A, Meese E. Emerging concepts of miRNA therapeutics: from cells to clinic. Trends Genet 2022;38:613-26.
85. Kara G, Calin GA, Ozpolat B. RNAi-based therapeutics and tumor targeted delivery in cancer. Adv Drug Deliv Rev 2022;182:114113.
86. Jiménez-Morales JM, Hernández-Cuenca YE, Reyes-Abrahantes A, et al. MicroRNA delivery systems in glioma therapy and perspectives: a systematic review. J Control Release 2022;349:712-30.
87. Han J, LaVigne CA, Jones BT, Zhang H, Gillett F, Mendell JT. A ubiquitin ligase mediates target-directed microRNA decay independently of tailing and trimming. Science 2020;370:eabc9546.
88. Statello L, Guo CJ, Chen LL, Huarte M. Gene regulation by long non-coding RNAs and its biological functions. Nat Rev Mol Cell Biol 2021;22:96-118.
89. Nojima T, Proudfoot NJ. Mechanisms of lncRNA biogenesis as revealed by nascent transcriptomics. Nat Rev Mol Cell Biol 2022;23:389-406.
90. Zhang Y, Luo M, Cui X, O’Connell D, Yang Y. Long noncoding RNA NEAT1 promotes ferroptosis by modulating the miR-362-3p/MIOX axis as a ceRNA. Cell Death Differ 2022;29:1850-63.
91. Singh D, Assaraf YG, Gacche RN. Long non-coding RNA mediated drug resistance in breast cancer. Drug Resist Updat 2022;63:100851.
92. Eptaminitaki GC, Stellas D, Bonavida B, Baritaki S. Long non-coding RNAs (lncRNAs) signaling in cancer chemoresistance: From prediction to druggability. Drug Resist Updat 2022;65:100866.
93. Yu Z, Tang H, Chen S, et al. Exosomal LOC85009 inhibits docetaxel resistance in lung adenocarcinoma through regulating ATG5-induced autophagy. Drug Resist Updat 2023;67:100915.
94. Gao Y, Shang S, Guo S, et al. Lnc2Cancer 3.0: an updated resource for experimentally supported lncRNA/circRNA cancer associations and web tools based on RNA-seq and scRNA-seq data. Nucleic Acids Res 2021;49:D1251-8.
95. Carlevaro-Fita J, Lanzós A, Feuerbach L, et al; PCAWG Drivers and Functional Interpretation Group; PCAWG Consortium. Cancer LncRNA Census reveals evidence for deep functional conservation of long noncoding RNAs in tumorigenesis. Commun Biol 2020;3:56.
97. Dasari S, Tchounwou PB. Cisplatin in cancer therapy: molecular mechanisms of action. Eur J Pharmacol 2014;740:364-78.
98. Perego P. Tackling cisplatin resistance in ovarian cancer: what can we do? Cancer Drug Resist 2021;4:755-7.
99. Amable L. Cisplatin resistance and opportunities for precision medicine. Pharmacol Res 2016;106:27-36.
100. Galluzzi L, Vitale I, Michels J, et al. Systems biology of cisplatin resistance: past, present and future. Cell Death Dis 2014;5:e1257.
101. Xie R, Cheng L, Huang M, et al. NAT10 drives cisplatin chemoresistance by enhancing ac4C-associated DNA repair in bladder cancer. Cancer Res 2023;83:1666-83.
102. Shi ZD, Hao L, Han XX, et al. Targeting HNRNPU to overcome cisplatin resistance in bladder cancer. Mol Cancer 2022;21:37.
103. Wu J, Zhou Z, Li J, et al. CHD4 promotes acquired chemoresistance and tumor progression by activating the MEK/ERK axis. Drug Resist Updat 2023;66:100913.
104. Oh SJ, Lim JY, Son MK, et al. TRPV1 inhibition overcomes cisplatin resistance by blocking autophagy-mediated hyperactivation of EGFR signaling pathway. Nat Commun 2023;14:2691.
105. Xiang L, Zeng Q, Liu J, et al. MAFG-AS1/MAFG positive feedback loop contributes to cisplatin resistance in bladder urothelial carcinoma through antagonistic ferroptosis. Sci Bull 2021;66:1773-88.
106. Tyagi A, Kaushal K, Chandrasekaran AP, et al. CRISPR/Cas9-based genome-wide screening for deubiquitinase subfamily identifies USP1 regulating MAST1-driven cisplatin-resistance in cancer cells. Theranostics 2022;12:5949-70.
107. Limagne E, Nuttin L, Thibaudin M, et al. MEK inhibition overcomes chemoimmunotherapy resistance by inducing CXCL10 in cancer cells. Cancer Cell 2022;40:136-52.e12.
108. Bray F, Ferlay J, Soerjomataram I, Siegel RL, Torre LA, Jemal A. Global cancer statistics 2018: GLOBOCAN estimates of incidence and mortality worldwide for 36 cancers in 185 countries. CA Cancer J Clin 2018;68:394-424.
109. Hong S, Zhang Y, Yu G, et al. Gemcitabine plus cisplatin versus fluorouracil plus cisplatin as first-line therapy for recurrent or metastatic nasopharyngeal carcinoma: final overall survival analysis of GEM20110714 phase III study. J Clin Oncol 2021;39:3273-82.
110. Tang QN, Liu LT, Qi B, et al. Effect of concurrent chemoradiotherapy with nedaplatin vs cisplatin on the long-term outcomes of survival and toxic effects among patients with stage II to IVB nasopharyngeal carcinoma: a 5-year follow-up secondary analysis of a randomized clinical trial. JAMA Netw Open 2021;4:e2138470.
111. Zheng ZQ, Li ZX, Guan JL, et al. Long noncoding RNA TINCR-mediated regulation of acetyl-CoA metabolism promotes nasopharyngeal carcinoma progression and chemoresistance. Cancer Res 2020;80:5174-88.
112. Xue F, Cheng Y, Xu L, et al. LncRNA NEAT1/miR-129/Bcl-2 signaling axis contributes to HDAC inhibitor tolerance in nasopharyngeal cancer. Aging 2020;12:14174-88.
113. Yang J, Xu J, Wang W, Zhang B, Yu X, Shi S. Epigenetic regulation in the tumor microenvironment: molecular mechanisms and therapeutic targets. Signal Transduct Target Ther 2023;8:210.
114. Tang J, Wang X, Xiao D, Liu S, Tao Y. The chromatin-associated RNAs in gene regulation and cancer. Mol Cancer 2023;22:27.
115. Johnson DE, Burtness B, Leemans CR, Lui VWY, Bauman JE, Grandis JR. Head and neck squamous cell carcinoma. Nat Rev Dis Primers 2020;6:92.
116. Sun S, Wu Y, Guo W, et al. STAT3/HOTAIR signaling axis regulates HNSCC growth in an EZH2-dependent manner. Clin Cancer Res 2018;24:2665-77.
117. Jiang Y, Guo H, Tong T, et al. lncRNA lnc-POP1-1 upregulated by VN1R5 promotes cisplatin resistance in head and neck squamous cell carcinoma through interaction with MCM5. Mol Ther 2022;30:448-67.
118. Steuer CE, El-Deiry M, Parks JR, Higgins KA, Saba NF. An update on larynx cancer. CA Cancer J Clin 2017;67:31-50.
119. Li R, Chen S, Zhan J, et al. Long noncoding RNA FOXD2-AS1 enhances chemotherapeutic resistance of laryngeal squamous cell carcinoma via STAT3 activation. Cell Death Dis 2020;11:41.
120. Pillai J, Chincholkar T, Dixit R, Pandey M. A systematic review of proteomic biomarkers in oral squamous cell cancer. World J Surg Oncol 2021;19:315.
121. Tian T, Lv X, Pan G, et al. Long noncoding RNA MPRL promotes mitochondrial fission and cisplatin chemosensitivity via disruption of pre-miRNA processing. Clin Cancer Res 2019;25:3673-88.
122. Filetti S, Durante C, Hartl D, et al; the ESMO Guidelines Committee. Thyroid cancer: ESMO Clinical Practice Guidelines for diagnosis, treatment and follow-up. Ann Oncol 2019;30:1856-83.
123. Shi L, Duan R, Sun Z et al. LncRNA GLTC targets LDHA for succinylation and enzymatic activity to promote progression and radioiodine resistance in papillary thyroid cancer. Cell Death Differ 2023;30:1517-32.
124. Wang Y, Zhang X, Wang Z, et al. LncRNA-p23154 promotes the invasion-metastasis potential of oral squamous cell carcinoma by regulating Glut1-mediated glycolysis. Cancer Lett 2018;434:172-83.
125. Sang L, Ju HQ, Yang Z, et al. Mitochondrial long non-coding RNA GAS5 tunes TCA metabolism in response to nutrient stress. Nat Metab 2021;3:90-106.
126. Tan DSW, Chong FT, Leong HS, et al. Long noncoding RNA EGFR-AS1 mediates epidermal growth factor receptor addiction and modulates treatment response in squamous cell carcinoma. Nat Med 2017;23:1167-75.
127. Zhang L, Meng X, Zhu XW, et al. Long non-coding RNAs in Oral squamous cell carcinoma: biologic function, mechanisms and clinical implications. Mol Cancer 2019;18:102.
128. Nair L, Chung H, Basu U. Regulation of long non-coding RNAs and genome dynamics by the RNA surveillance machinery. Nat Rev Mol Cell Biol 2020;21:123-36.
129. Zhang L, Xu X, Su X. Noncoding RNAs in cancer immunity: functions, regulatory mechanisms, and clinical application. Mol Cancer 2020;19:48.
130. Jiang W, Pan S, Chen X, Wang ZW, Zhu X. The role of lncRNAs and circRNAs in the PD-1/PD-L1 pathway in cancer immunotherapy. Mol Cancer 2021;20:116.
131. Zhou WY, Cai ZR, Liu J, Wang DS, Ju HQ, Xu RH. Circular RNA: metabolism, functions and interactions with proteins. Mol Cancer 2020;19:172.
132. Cheng J, Li G, Wang W, Stovall DB, Sui G, Li D. Circular RNAs with protein-coding ability in oncogenesis. Biochim Biophys Acta Rev Cancer 2023;1878:188909.
133. Zhang Q, Wang W, Zhou Q, et al. Roles of circRNAs in the tumour microenvironment. Mol Cancer 2020;19:14.
134. Pisignano G, Michael DC, Visal TH, Pirlog R, Ladomery M, Calin GA. Going circular: history, present, and future of circRNAs in cancer. Oncogene 2023;42:2783-800.
135. Niu D, Wu Y, Lian J. Circular RNA vaccine in disease prevention and treatment. Signal Transduct Target Ther 2023;8:341.
136. Wu S, Lu J, Zhu H, et al. A novel axis of circKIF4A-miR-637-STAT3 promotes brain metastasis in triple-negative breast cancer. Cancer Lett 2024;581:216508.
137. Wang Z, Yang L, Wu P, et al. The circROBO1/KLF5/FUS feedback loop regulates the liver metastasis of breast cancer by inhibiting the selective autophagy of afadin. Mol Cancer 2022;21:29.
138. Hong X, Li Q, Li J, et al. CircIPO7 promotes nasopharyngeal carcinoma metastasis and cisplatin chemoresistance by facilitating YBX1 nuclear localization. Clin Cancer Res 2022;28:4521-35.
139. Li Q, Zhao YH, Xu C, et al. Chemotherapy-induced senescence reprogramming promotes nasopharyngeal carcinoma metastasis by circRNA-mediated PKR activation. Adv Sci 2023;10:e2205668.
140. Debnath J, Gammoh N, Ryan KM. Autophagy and autophagy-related pathways in cancer. Nat Rev Mol Cell Biol 2023;24:560-75.
142. Gao W, Guo H, Niu M, et al. circPARD3 drives malignant progression and chemoresistance of laryngeal squamous cell carcinoma by inhibiting autophagy through the PRKCI-Akt-mTOR pathway. Mol Cancer 2020;19:166.
143. Liu F, Zhang J, Qin L, et al. Circular RNA EIF6 (Hsa_circ_0060060) sponges miR-144-3p to promote the cisplatin-resistance of human thyroid carcinoma cells by autophagy regulation. Aging 2018;10:3806-20.
145. Ji X, Lv C, Huang J, Dong W, Sun W, Zhang H. ALKBH5-induced circular RNA NRIP1 promotes glycolysis in thyroid cancer cells by targeting PKM2. Cancer Sci 2023;114:2318-34.
146. Cui Y, Liu J, Liu L, et al. m6A-modified circFOXK2 targets GLUT1 to accelerate oral squamous cell carcinoma aerobic glycolysis. Cancer Gene Ther 2023;30:163-71.
147. Zhao W, Liu J, Wu J, et al. High-throughput microarray reveals the epitranscriptome-wide landscape of m6A-modified circRNA in oral squamous cell carcinoma. BMC Genomics 2022;23:611.
148. Li J, Cao H, Yang J, Wang B. IGF2BP2-m6A-circMMP9 axis recruits ETS1 to promote TRIM59 transcription in laryngeal squamous cell carcinoma. Sci Rep 2024;14:3014.
149. Wu P, Fang X, Liu Y, et al. N6-methyladenosine modification of circCUX1 confers radioresistance of hypopharyngeal squamous cell carcinoma through caspase1 pathway. Cell Death Dis 2021;12:298.
150. Jiang X, Stockwell BR, Conrad M. Ferroptosis: mechanisms, biology and role in disease. Nat Rev Mol Cell Biol 2021;22:266-82.
151. Sun S, Shen J, Jiang J, Wang F, Min J. Targeting ferroptosis opens new avenues for the development of novel therapeutics. Signal Transduct Target Ther 2023;8:372.
152. Zhu J, Wang X, Su Y, et al. Multifunctional nanolocks with GSH as the key for synergistic ferroptosis and anti-chemotherapeutic resistance. Biomaterials 2022;288:121704.
153. Zhang J, Ye ZW, Chakraborty P, et al. Microsomal glutathione transferase 1 controls metastasis and therapeutic response in melanoma. Pharmacol Res 2023;196:106899.
154. Yuan L, Li S, Chen Q, et al. EBV infection-induced GPX4 promotes chemoresistance and tumor progression in nasopharyngeal carcinoma. Cell Death Differ 2022;29:1513-27.
155. Roh JL, Kim EH, Jang H, Shin D. Nrf2 inhibition reverses the resistance of cisplatin-resistant head and neck cancer cells to artesunate-induced ferroptosis. Redox Biol 2017;11:254-62.
156. Shin D, Kim EH, Lee J, Roh JL. Nrf2 inhibition reverses resistance to GPX4 inhibitor-induced ferroptosis in head and neck cancer. Free Radic Biol Med 2018;129:454-62.
157. Lee J, You JH, Shin D, Roh JL. Inhibition of glutaredoxin 5 predisposes cisplatin-resistant head and neck cancer cells to ferroptosis. Theranostics 2020;10:7775-86.
158. Yang JY, Lei XY, He KY, et al. HMGA1 drives chemoresistance in esophageal squamous cell carcinoma by suppressing ferroptosis. Cell Death Dis 2024;15:158.
159. Angeli JP, Krysko DV, Conrad M. Ferroptosis at the crossroads of cancer-acquired drug resistance and immune evasion. Nat Rev Cancer 2019;19:405-14.
160. Li B, Yang L, Peng X, et al. Emerging mechanisms and applications of ferroptosis in the treatment of resistant cancers. Biomed Pharmacother 2020;130:110710.
161. Sun X, Niu X, Chen R, et al. Metallothionein-1G facilitates sorafenib resistance through inhibition of ferroptosis. Hepatology 2016;64:488-500.
162. Jiang Z, Lim SO, Yan M, et al. TYRO3 induces anti-PD-1/PD-L1 therapy resistance by limiting innate immunity and tumoral ferroptosis. J Clin Invest 2021;131:139434.
163. Qi R, Bai Y, Li K, et al. Cancer-associated fibroblasts suppress ferroptosis and induce gemcitabine resistance in pancreatic cancer cells by secreting exosome-derived ACSL4-targeting miRNAs. Drug Resist Updat 2023;68:100960.
164. Liang J, Bi G, Huang Y, et al. MAFF confers vulnerability to cisplatin-based and ionizing radiation treatments by modulating ferroptosis and cell cycle progression in lung adenocarcinoma. Drug Resist Updat 2024;73:101057.
165. Zhang X, Xu Y, Ma L, et al. Essential roles of exosome and circRNA_101093 on ferroptosis desensitization in lung adenocarcinoma. Cancer Commun 2022;42:287-313.
166. Jiang M, Jike Y, Liu K, et al. Exosome-mediated miR-144-3p promotes ferroptosis to inhibit osteosarcoma proliferation, migration, and invasion through regulating ZEB1. Mol Cancer 2023;22:113.
167. Xi Y, Shen Y, Wu D, et al. CircBCAR3 accelerates esophageal cancer tumorigenesis and metastasis via sponging miR-27a-3p. Mol Cancer 2022;21:145.
168. Zhang C, Liu X, Jin S, Chen Y, Guo R. Ferroptosis in cancer therapy: a novel approach to reversing drug resistance. Mol Cancer 2022;21:47.
169. Zhang C, Xu C, Gao X, Yao Q. Platinum-based drugs for cancer therapy and anti-tumor strategies. Theranostics 2022;12:2115-32.
170. Huang Y, Kou Q, Su Y, et al. Combination therapy based on dual-target biomimetic nano-delivery system for overcoming cisplatin resistance in hepatocellular carcinoma. J Nanobiotechnology 2023;21:89.
171. Siemer S, Bauer TA, Scholz P, et al. Targeting cancer chemotherapy resistance by precision medicine-driven nanoparticle-formulated cisplatin. ACS Nano 2021;15:18541-56.
172. Zhang R, You X, Luo M, et al. Poly(β-cyclodextrin)/platinum prodrug supramolecular nano system for enhanced cancer therapy: synthesis and in vivo study. Carbohydr Polym 2022;292:119695.
173. Chen J, Wang X, Yuan Y, et al. Exploiting the acquired vulnerability of cisplatin-resistant tumors with a hypoxia-amplifying DNA repair-inhibiting (HYDRI) nanomedicine. Sci Adv 2021;7:eabc5267.
174. Chen Y, Fang L, Zhou W, et al. Nitric oxide-releasing micelles with intelligent targeting for enhanced anti-tumor effect of cisplatin in hypoxia. J Nanobiotechnology 2021;19:246.
175. Pan WL, Tan Y, Meng W, et al. Microenvironment-driven sequential ferroptosis, photodynamic therapy, and chemotherapy for targeted breast cancer therapy by a cancer-cell-membrane-coated nanoscale metal-organic framework. Biomaterials 2022;283:121449.
176. Zhang Z, Ji Y, Hu N, et al. Ferroptosis-induced anticancer effect of resveratrol with a biomimetic nano-delivery system in colorectal cancer treatment. Asian J Pharm Sci 2022;17:751-66.
177. Li K, Lin C, Li M, et al. Multienzyme-like reactivity cooperatively impairs glutathione peroxidase 4 and ferroptosis suppressor protein 1 pathways in triple-negative breast cancer for sensitized ferroptosis therapy. ACS Nano 2022;16:2381-98.
178. Li K, Xu K, He Y, et al. Oxygen self-generating nanoreactor mediated ferroptosis activation and immunotherapy in triple-negative breast cancer. ACS Nano 2023;17:4667-87.
179. Chen D, Liang C, Qu X, et al. Metal-free polymer nano-photosensitizer actuates ferroptosis in starved cancer. Biomaterials 2023;292:121944.
180. Liu J, Ren L, Li S, et al. The biology, function, and applications of exosomes in cancer. Acta Pharm Sin B 2021;11:2783-97.
181. Wu J, Li S, Zhang P. Tumor-derived exosomes: immune properties and clinical application in lung cancer. Cancer Drug Resist 2022;5:102-13.
182. Wang Z, He J, Bach DH, et al. Induction of m6A methylation in adipocyte exosomal LncRNAs mediates myeloma drug resistance. J Exp Clin Cancer Res 2022;41:4.
183. Shi L, Zhu W, Huang Y, et al. Cancer-associated fibroblast-derived exosomal microRNA-20a suppresses the PTEN/PI3K-AKT pathway to promote the progression and chemoresistance of non-small cell lung cancer. Clin Transl Med 2022;12:e989.
184. Pan Z, Zheng J, Zhang J, et al. A novel protein encoded by exosomal circATG4B induces oxaliplatin resistance in colorectal cancer by promoting autophagy. Adv Sci 2022;9:e2204513.
185. Jing X, Xie M, Ding K, et al. Exosome-transmitted miR-769-5p confers cisplatin resistance and progression in gastric cancer by targeting CASP9 and promoting the ubiquitination degradation of p53. Clin Transl Med 2022;12:e780.
186. Liu X, Guo Q, Gao G, et al. Exosome-transmitted circCABIN1 promotes temozolomide resistance in glioblastoma via sustaining ErbB downstream signaling. J Nanobiotechnology 2023;21:45.
187. Deng J, Pan T, Lv C, et al. Exosomal transfer leads to chemoresistance through oxidative phosphorylation-mediated stemness phenotype in colorectal cancer. Theranostics 2023;13:5057-74.
188. Zhang F, Jiang J, Qian H, Yan Y, Xu W. Exosomal circRNA: emerging insights into cancer progression and clinical application potential. J Hematol Oncol 2023;16:67.
189. Guo X, Gao C, Yang DH, Li S. Exosomal circular RNAs: a chief culprit in cancer chemotherapy resistance. Drug Resist Updat 2023;67:100937.
191. Marei H, Tsai WK, Kee YS, et al. Antibody targeting of E3 ubiquitin ligases for receptor degradation. Nature 2022;610:182-9.
192. Xiong Y, Zhong Y, Yim H, et al. Bridged proteolysis targeting chimera (PROTAC) enables degradation of undruggable targets. J Am Chem Soc 2022;144:22622-32.
193. Ghidini A, Cléry A, Halloy F, Allain FHT, Hall J. RNA-PROTACs: degraders of RNA-binding proteins. Angew Chem Int Ed Engl 2021;60:3163-9.
194. Li X, Pu W, Zheng Q, Ai M, Chen S, Peng Y. Proteolysis-targeting chimeras (PROTACs) in cancer therapy. Mol Cancer 2022;21:99.
195. Wu Y, Chang X, Yang G, et al. A physiologically responsive nanocomposite hydrogel for treatment of head and neck squamous cell carcinoma via proteolysis-targeting chimeras enhanced immunotherapy. Adv Mater 2023;35:e2210787.
196. Jin J, Wu Y, Zhao Z, et al. Small-molecule PROTAC mediates targeted protein degradation to treat STAT3-dependent epithelial cancer. JCI Insight 2022;7:e160606.
198. Yang F, Luo Q, Wang Y, et al. Targeted biomolecule regulation platform: a split-and-mix PROTAC approach. J Am Chem Soc 2023;145:7879-87.
200. Zhang C, Xu J, Tang R, et al. Novel research and future prospects of artificial intelligence in cancer diagnosis and treatment. J Hematol Oncol 2023;16:114.
Cite This Article

How to Cite
Zhang, Y.; Peng Y.; Lin B.; Yang S.; Deng F.; Yang X.; Li A.; Xia W.; Gao C.; Lei S.; Liao W.; Zeng Q. Non-coding RNA and drug resistance in head and neck cancer. Cancer. Drug. Resist. 2024, 7, 34. http://dx.doi.org/10.20517/cdr.2024.59
Download Citation
Export Citation File:
Type of Import
Tips on Downloading Citation
Citation Manager File Format
Type of Import
Direct Import: When the Direct Import option is selected (the default state), a dialogue box will give you the option to Save or Open the downloaded citation data. Choosing Open will either launch your citation manager or give you a choice of applications with which to use the metadata. The Save option saves the file locally for later use.
Indirect Import: When the Indirect Import option is selected, the metadata is displayed and may be copied and pasted as needed.
About This Article
Special Issue
Copyright
Data & Comments
Data
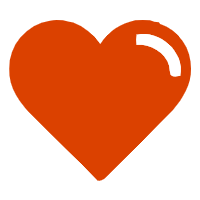
Comments
Comments must be written in English. Spam, offensive content, impersonation, and private information will not be permitted. If any comment is reported and identified as inappropriate content by OAE staff, the comment will be removed without notice. If you have any queries or need any help, please contact us at support@oaepublish.com.