Circular RNAs modulate cancer drug resistance: advances and challenges
Abstract
Acquired drug resistance is a main factor contributing to cancer therapy failure and high cancer mortality, highlighting the necessity to develop novel intervention targets. Circular RNAs (circRNAs), an abundant class of RNA molecules with a closed loop structure, possess characteristics including high stability, which provide unique advantages in clinical application. Growing evidence indicates that aberrantly expressed circRNAs are associated with resistance against various cancer treatments, including targeted therapy, chemotherapy, radiotherapy, and immunotherapy. Therefore, targeting these aberrant circRNAs may offer a strategy to improve the efficiency of cancer therapy. Herein, we present a summary of the most recently studied circRNAs and their regulatory roles on cancer drug resistance. With the advances in artificial intelligence (AI)-based bioinformatics algorithms, circRNAs could emerge as promising biomarkers and intervention targets in cancer therapy.
Keywords
INTRODUCTION
Despite significant advances in cancer treatment over the decades, cancer remains one of the most clinically lethal diseases, mainly due to the development of acquired drug resistance[1,2]. Patients initially exhibit a high response rate to cancer treatment, with a noticeable suppression of cancer progression. However, acquired drug resistance develops when a substantial proportion of cancer cells become resistant to cancer treatment options, leading to cancer recurrence or progression[1,3,4]. A variety of factors contribute to the formation of drug-resistant cancer cells, such as tumor heterogeneity, tumor microenvironment and so on[4-7]. Therefore, it is urgent to develop novel intervention targets and effective strategies to reverse cancer drug resistance, which facilitates the clinical outcomes of cancer treatment.
Circular RNAs (circRNAs) are a special type of non-coding RNA molecules that form a circular conformation via non-canonical splicing or back-splicing events[8]. CircRNAs were initially considered by-products of transcription with low abundance[8,9]. With the rapid development of high-throughput sequencing and bioinformatics algorithms, thousands of circRNAs have been identified across a wide range of species, from viruses to mammals. Notably, aberrant expression of circRNAs in various diseases prompted further research into their potential regulatory functions. Accumulating evidence has demonstrated that circRNAs are associated with specific hallmarks of cancer, including sustaining proliferative signaling, evading growth suppressors, and activation of invasion and metastasis[10]. Moreover, a series of circRNAs with significantly altered expression following cancer treatment are involved in modulating cancer drug resistance[11-13]. Therefore, elucidating the functions and mechanisms of these circRNAs could provide a deeper understanding of cancer drug resistance.
In this review, we summarize the current research on circRNAs in various cancer treatment strategies, including resistance to targeted therapy, chemotherapy, radiotherapy, and immunotherapy. The development of emerging technologies, such as artificial intelligence (AI)-aided design, has greatly improved the efficiency of circRNA synthesis and delivery. Several challenges regarding the clinical translation and application of circRNAs are also discussed. We aim to shed new light on circRNAs-mediated cancer drug resistance and their translational potential as intervention targets for cancer therapy, developing clinical protocols for circRNAs-based therapies to overcome cancer drug resistance.
BIOGENESIS AND REGULATORY ROLES OF CIRCRNAS
CircRNAs are a class of endogenous RNA molecules derived from precursor mRNA (pre-mRNA) via back-splicing, in which a downstream splice site is joined with an upstream splice site[8]. Reverse complementary sequences in the flanking regions of circularized exons, along with RNA-binding proteins, are the major factors contributing to circRNA biogenesis[8,14]. CircRNAs can be mainly divided into two groups based on their sequence origin. One group consists of cytosolic genome-derived circRNAs, including exonic circRNA (EcircRNA), exon-intron circRNA (EIciRNA), and intronic circRNA (CiRNA), while the other comprises the mitochondria-encoded circRNAs (MecciRNAs)[6,15].
The function of circRNAs is associated with their subcellular location patterns. Generally, cytoplasmic circRNAs exert their regulatory roles by acting as microRNA sponges to remove the inhibitory effects on the targets, serving as RNA-binding protein decoys to modulate downstream biological processes and acting as templates for translation to generate functional polypeptides. In contrast, nucleus-localized circRNAs typically regulate the expression of target genes by affecting their transcriptional processes in the promoter region[15].
CIRCRNAS HOLD POTENTIAL AS BIOMARKERS FOR TUMOR DIAGNOSIS AND PROGNOSIS
Due to their covalently closed-loop structure and lack of exposed terminal ends, circRNAs are resistant to degradation by exonucleases and highly stable in blood and other body fluids[14,16]. As shown in Figure 1 and Table 1, numerous dysregulated circRNAs and their expression patterns are associated with the progression of multiple cancers, emerging as promising prognostic biomarkers and intervention targets[17-38]. A total of 59,056 circRNAs were identified from 122 pairs of clinical samples among seven solid tumors and their adjacent normal tissues, with dysregulated circRNAs exhibiting cancer-specific expression or shared common expression signatures across cancers[17]. Xu et al. conducted a plasma-derived liquid biopsy profiling to systematically screen upregulated circRNA candidates in pancreatic ductal adenocarcinoma (PDAC), and ultimately established a circRNAs-based biomarker panel in conjunction with cancer antigen 19-9 expression to enable noninvasive detection of early-stage PDAC[18]. Moreover, several consortium projects including The Cancer Genome Atlas (TCGA), ExoRBase, and CircRNADisease have provided vast circRNA sequencing data. DeepBase integrates extensive clinical data on cancer-associated circRNAs, and analyzes the correlation between circRNA expression and survival outcomes of cancer patients[19].
Figure 1. Clinical significance and application of circRNAs. This diagram illustrates the various roles and functions of circRNAs in biological systems. A large number of dysregulated circRNAs and their expression patterns are associated with the progression of multiple cancers, emerging as diagnostic biomarkers and pathological intervention targets. Additionally, circRNAs show the potential to serve as antisense RNAs to interfere with transcription processes, act as aptamers or translational templates to regulate protein expression, and either activate or inhibit immune responses. circRNAs: Circular RNAs.
Clinicopathological characteristic of circRNAs in cancers
CircRNA | Cancer type | Cases | Expression | TNM stage (I + II/III + IV) |
CircRTN4[24] | PDAC | 87 | Upregulation | 73/14 |
CircCAPRIN1[25] | Colorectal cancer | 259 | Upregulation | 95/164 |
CircPDHK1[26] | Clear cell renal cell carcinoma | 148 | Upregulation | 97/51 |
CircARID1A[27] | Gastric cancer | 21 | Upregulation | 3/18 |
CircNFIB[28] | Intrahepatic cholangiocarcinoma | 182 | Downregulation | 63/119 |
CircSHKBP1[29] | Gastric cancer | 76 | Upregulation | 34/118 |
CircCDR1as[30] | Gastric cancer | 82 | Downregulation | 28/54 |
Circ_0018289[31] | Cervical cancer | 192 | Upregulation | 119/73 |
Circ_0003159[32] | Gastric cancer | 108 | Downregulation | 30/78 |
Circ_100876[33] | Non-small cell lung cancer | 101 | Upregulation | 58/43 |
CircNRIP1[34] | Gastric cancer | 80 | Upregulation | 40/40 |
CircUSP7[35] | Non-small cell lung cancer | 126 | Upregulation | 61/65 |
Circ_0001006[36] | Triple-negative breast cancer | 133 | Upregulation | 82/51 |
Circ_001988[37] | Colorectal cancer | 31 | Downregulation | 15/16 |
Circ_0048764[38] | Breast cancer | 41 | Upregulation | 27/14 |
Aberrant expression of regulatory circRNAs may directly or indirectly contribute to oncogenesis and progression. For instance, a downregulated circRERE acts as a competitive endogenous RNA against miR-6837-3p, alleviating the repressive effect on MAVS and suppressing colorectal cancer progression[20]. Li et al. reported that upregulated circLARP1B acts as a protein decoy for hnRNPD to weaken the stability of LKB1 mRNA, facilitating cellular lipid accumulation and hepatocellular carcinoma metastasis[21]. Additionally, activation of circular E-cadherin RNA encodes an oncogenic E-cadherin protein variant through multiple-round open reading frame (ORF) translation, enhancing EGFR-STAT3 signaling transduction and glioblastoma tumorigenicity[22]. Nuclear-localized circMYBL1 enhances the binding of CEBPB to CD44 promoter regions, promoting CD44 transcription and adenoid cystic carcinoma progression[23].
CIRCRNAS ARE WIDELY INVOLVED IN REGULATING CANCER DRUG RESISTANCE
Despite significant progress in cancer treatments, nearly 90% of cancer-related deaths are attributed to the emergence of cancer drug resistance or drug resistance[39]. Therefore, identifying novel regulatory targets that mediate cancer drug resistance is necessary to develop effective intervention strategies. CircRNAs are associated with multiple mechanisms of acquired cancer drug resistance, such as epithelial to mesenchymal transition, DNA damage repair, immune evasion, tumor-promoting inflammation, and genome instability[40-43]. As depicted in Table 2, circRNAs regulate various pathways involved in drug resistance, demonstrating strong potential in reversing drug resistance. Here, we illustrate the commonly proposed mechanisms by which circRNAs contribute to cancer drug resistance, with selected examples.
CircRNAs regulate various pathways involved in drug resistance
CircRNAs | Drug resistance | Mechanisms or pathway |
CircDCAF8 | Regorafenib | Exosome transmission[44] |
CircVAPA | BMS536924 | MiR-377-3p & miR-494-3p/IGF1R[45] |
CircMED27 | Lenvatinib | MiR-655-3p/USP28[46] |
CircESRP1 | Cisplatin | MiR-93-5p/Smad7/p21-mediated epithelial-mesenchymal transition[47] |
CircPDIA3 | Oxaliplatin | CircPDIA3/miR-449a/XBP1 feedback loop[48] |
CircATG4B | Oxaliplatin | Encoding circATG4B-222aa that prevents TMED10 from binding to ATG4B[49] |
CircMETRN | Radiotherapy | MiR-4709-3p/GRB14/PDGFR[50] |
CircCDYL2 | Radiotherapy | RAD51-mediated homologous recombination repair capability[51] |
CircNOP14 | Radiotherapy | Ku70-dependent DNA damage repair[52] |
CircKEAP1 | Immunotherapy | IFN-γ[53] |
CircNCOA3 | Immunotherapy | MiR-203a-3p.1/CXCL1[54] |
CircCCAR1 | Immunotherapy | CircCCAR1/miR-127-5p/WTAP feedback loop[55] |
CircPVT1 | Chemotherapy | MiR-145-5p/ABCC1[56] |
CircPVT1 | Radiotherapy | MiR-1208/PI3K/AKT/mTOR[57] |
CircPPAPDC1A | Osimertinib | MiR-30a-3p/IGF1R[58] |
CircITGB6 | Cisplatin | CircITGB6/IGF2BP2/FGF9 RNA-protein complex[59] |
CircRNAs and resistance to targeted therapy
Targeted therapy is an approach to inhibit tumor growth or metastasis by blocking cancer-specific genes and signaling pathways based on gene mutations/expression[60-63]. Compared with traditional chemotherapy, targeted therapy can specifically kill tumor cells with minimal impact on normal cells and reduce drug side effects[64]. It has been widely accepted that the high failure rate of clinical targeted therapy may be attributed to cancer drug resistance. However, the role of circRNAs in targeted therapy drug resistance remains to be fully elucidated. A series of studies have demonstrated that targeting circRNAs could sensitize cancer cells to cancer therapy. Regorafenib is a multi-targeted receptor tyrosine kinase inhibitor that shows high antitumor and anti-angiogenic activity in hepatocellular carcinoma[65-67]. Gong et al. reported that circDCAF8 potentiates regorafenib resistance through exosome transmission, providing a promising protocol of circDCAF8 inhibition in combination with regorafenib for treating regorafenib-resistant hepatocellular carcinoma[44]. Aberrant regulation of the IGF1R signaling pathway has been recognized as a well-established therapeutic target in small cell lung cancer[68,69]. Hua et al. revealed that circVAPA knockdown enhances the dual repressive role of miR-377-3p and miR-494-3p on IGF1R expression, ultimately inhibiting small cell lung cancer progression[45]. Zhang et al. demonstrated that upregulated circMED27 is correlated with poor prognosis of hepatocellular carcinoma and elevated lenvatinib resistance via the miR-655-3p/USP28 axis, concluding that circMED27 inhibition may represent a promising strategy to overcome lenvatinib resistance[46].
CircRNAs and chemotherapy resistance
Chemotherapy combats cancers by disrupting DNA/RNA/protein synthesis or function to induce apoptosis[42,70-72]. However, the susceptibility of chemotherapy resistance remains the leading cause of treatment failure[73]. Multiple complex etiologies associated with chemoresistance contribute to poor prognosis of cancer, such as the inhibition of pathways mediating cell death, promotion of damaged DNA repair, and reduction of cellular drug accumulation[74]. Recent studies indicate that circRNAs regulate chemotherapy response as potential therapeutic targets to overcome drug resistance and improve outcomes.
Cisplatin is a well-known chemotherapeutic drug used in the treatment of various cancers. It induces cancer cells apoptosis of cancer cells by interfering with DNA repair[75-81]. CircESRP1 expression is aberrantly downregulated in the cisplatin-resistant cells compared with the parental cisplatin-sensitive cells, enhancing cisplatin sensitivity of small cell lung cancer via the miR-93-5p/Smad7/p21(CDKN1A) axis[47]. Since circESRP1 overexpression has a potent inhibitory effect on epithelial-mesenchymal transition, Huang et al. suggested that circESRP1 overexpression may be a strategy to overcome chemotherapy resistance in small cell lung cancer[47]. Oxaliplatin is a platinum-based chemotherapeutic agent that induces DNA damage by forming intra- and interstrand crosslinks, widely used in colorectal cancer treatment[82]. However, the development of oxaliplatin resistance severely limits its clinical use and efficacy. CircPDIA3 is negatively associated with disease-free survival of colorectal cancer patients, inducing oxaliplatin resistance via circPDIA3/miR-449a/XBP1 feedback loop[48]. Pan et al. concluded that circATG4B-222aa, a novel protein encoded by circATG4B, could act as a decoy that prevents TMED10 from binding to ATG4B, ultimately contributing to increased autophagy and oxaliplatin resistance[49].
CircRNAs and radiotherapy resistance
Radiation therapy combats cancers by inducing DNA damage and triggering cell cycle arrest, senescence, and apoptosis via ionizing radiation[83-86]. CircRNAs have also been implicated in radiation therapy. For example, Wang et al. used RNA sequencing techniques to assess the expression profiles of aberrant circRNAs undergoing glioma radiotherapy, demonstrating that low-dose radiation-induced exosome circMETRN could promote DNA damage repair process and radiotherapy resistance in glioblastoma cells through the miR-4709-3p/GRB14/PDGFRα axis[50]. Qu et al. revealed that circCDYL2 facilitates the initiation of RAD51 translation and homologous recombination repair capability through recruitment of EIF3D protein to the 5’-untranslated regions (UTR) of RAD51 mRNA, ultimately leading to radiotherapy resistance in nasopharyngeal carcinoma[51]. Similarly, circNOP14 enhances the radiosensitivity of hepatocellular carcinoma cells via Ku70 interaction to suppress Ku70-dependent DNA repair, providing a potential therapeutic target for hepatocellular carcinoma radiotherapy[52]. These studies provide mechanistic insights into the roles of circRNAs and identify valuable markers for overcoming cancer radiotherapy resistance.
CircRNAs and immunotherapy resistance
Tumor immunotherapy is an approach designed to activate the immune cells and enhance the antitumor immune response with minimal side effects, specifically targeting small residual tumor lesions and inhibiting tumor growth[87-89]. It is well known that RIG-I signaling is involved in the transcriptional activation and expression of multiple pro-inflammatory genes[90-93]. Zhang et al. revealed that N6-methyladenosine (m6A)-modified circKEAP1 could interact with RIG-I to activate antitumor immunity through the IFN-γ pathway, providing new insights into the immune response in osteosarcoma[53]. Significant clinical progress has been made in various therapies targeting immune checkpoint inhibitors including programmed death receptor 1 (PD1) and PD1 ligand 1 (PD-L1)[94-96]. For example, circNCOA3 is elevated in anti-PD-1-resistant colorectal cancer, functioning as a competing endogenous RNA to modulate CXCL1 expression and facilitate immune evasion[54]. Exosome-derived circCCAR1 accelerates the exhaustion of antitumor CD8+ T cells and promotes resistance to anti-PD1 therapy via the circCCAR1/miR-127-5p/WTAP feedback loop[55]. Zou et al. provided a new strategy to overcome deruxtecan resistance by inhibiting the interaction between the VDAC3-derived circRNA and HSPB1 protein[97]. CircRNAs exhibit low immunogenicity, mainly due to their covalently closed structure lacking a free 5’ cap and 3’ poly-A tail, a common pathogen-associated molecular pattern identified by innate immunosensors[98-100]. In addition, their endogenous source and stable exonuclease resistance minimize interactions with cytoplasmic RNA sensors, thereby reducing the risk of interferon activation. Notably, circRNA avoids the dsRNA-induced immune response observed in linear RNA. Yang et al. comprehensively summarized the progress and application of RNA vaccines in antitumor therapy, while outlining future directions for expanding this promising platform to a variety of therapeutic applications[98]. Li et al. highlighted the potential of exosomal circRNAs as diagnostic and prognostic predictive biomarkers, as well as a new strategy for clinical therapy[99]. Taken together, these findings demonstrate that circRNAs play a significant role in regulating immune therapeutic responses.
CHALLENGES AND APPROACHES OF CIRCRNAS IN CLINICAL APPLICATION
CircRNAs exhibit unique advantages in druggable transformation
A large body of clinical or experimental evidence suggests that circRNAs may serve as promising therapeutic targets to reverse cancer drug resistance[19,100,101], providing new insights into expanding druggable targets from proteins to circRNAs. Based on data from the Gene Expression Omnibus (GEO) and Connectivity Graph (CMap) databases, Cao et al. identified 55 circRNAs for potential drug targeting and 2,802 circRNAs associated with drug resistance[101]. The ncRNADrug database curates validated drug resistance-associated circRNAs, drug-targeted circRNAs, and resistant cancer drug combinations, enabling the prediction of circRNA-drug resistance interactions[101]. The covalently closed-loop structure and the lack of exposed terminal ends enable circRNAs to evade cellular recognition as exogenous entities, thereby reducing their immunogenicity[102,103]. Drugs that target circRNAs, such as antisense oligonucleotides (ASOs) and small interfering RNAs (siRNAs), are RNA-based therapeutics that are not constrained by protein structures. These nucleic acid drugs exhibit advantages including broad druggable targets and high specificity. Multiple ASOs bound to circRNAs through complementary base pairing can effectively hinder their functions or expression. For instance, ASO-circSKA3 inhibits colorectal cancer progression by disrupting the SLUG-circSKA3 interaction, promoting SLUG ubiquitination and degradation[104]. Drugs can also disrupt circRNA-protein/RNA interactions or modulate circRNA stability. For example, circRNA-SORE is significantly upregulated in sorafenib-resistant hepatocellular carcinoma cells[105]. Mechanistically, circRNA-SORE binds to YBX1 and prevents PRP19-mediated ubiquitination and YBX1 degradation, thereby inducing sorafenib resistance. Silencing circRNA-SORE with specific siRNAs is shown to be effective in overcoming chemoresistance to sorafenib[105]. Overall, circRNAs exhibit characteristics such as high stability, abundance, and low immunogenicity, which make them particularly advantageous for cancer vaccines.
Challenges of circRNAs in clinical application
As circRNAs gain more attention, regulatory circRNAs may emerge as novel nucleic acid drugs to overcome cancer drug resistance [Figure 1]. CircRNAs can function as RNA or protein aptamers, bind specifically to certain RNA molecules/proteins, and modulate the process of translating RNA into proteins. These circRNAs can be used as therapeutic tools to treat diseases. However, several challenges remain in the clinical translation of circRNAs, including their synthesis, purification, and delivery[102,103].
The conventional synthesis of circRNAs in vitro mainly uses linearized plasmid DNA as a template for in vitro transcription to produce precursor RNA, which is then cyclized through chemical or enzymatic ligation[106,107]. Various factors, such as gene sequence variations and length constraints, adversely affect both the circularization efficiency and production scalability of these constructs, ultimately leading to suboptimal yields[106,108]. Moreover, it is difficult to separate circRNAs from linear RNA precursors and nicked RNAs of similar molecular weight, highlighting the urgent need to improve the purification efficiency and quality control of circRNA products[109]. Lipid nanoparticles (LNPs) are lipid-based nanoparticles that have been widely used as delivery vehicles for functional RNAs including mRNAs, siRNAs, and circRNAs[110-118]. However, there is a pressing need to substantially improve the modification and delivery tropism of LNPs to extend their application to extrahepatic tissues. Further development of novel nanoparticle systems is essential to improve the delivery efficiency and application range of circRNAs.
Emerging technologies facilitate the clinical translation of circRNAs
Researchers have made notable progress on the above issues in the past decade. Large-scale single-cell transcriptomic studies have unveiled that a substantial proportion of circRNAs exhibit cell-specific expression patterns. Several databases serve as a critical resource for investigating the dynamic changes of circRNAs during embryonic development, tissue differentiation, and cancer biogenesis, while providing a unique and functional platform for the circRNA research community. The superior applicability of circRNAs as cell-type biomarkers in exploring tumor-infiltrating immune cell heterogeneity further underscores their essential biological roles in specific cellular contexts[119]. With the development of emerging technologies including AI-aided design, the clinical translation and application prospects of RNA-based therapeutics and drugs have significantly improved. Recently, a novel algorithm tool called circDesign was developed to optimize open reading frame (ORF) sequences, facilitating the circularization and translation of synthetic circRNAs, as well as enhancing the immune responses in vivo[120]. Lee et al. developed a circRNA engineering strategy involving end-to-end self-targeting and splicing reaction using Tetrahymena group I intron ribozyme, which effectively generates circRNAs in vitro[106]. To purify the circRNAs, candidate RNA bands matching the expected molecular weights were extracted by gel electrophoresis and digested with RNase R[103,106]. In addition, Wesselhoeft et al. combined SEC-HPLC with RNase R digestion to prepare circRNAs with a purity of 90%, significantly outperforming RNase R digestion alone[121]. This strategy further amplifies the differences between circRNAs, linear precursor RNAs, and nicked RNAs, improving the separation efficiency of circRNAs.
Several studies have reported advances in circRNA delivery systems. For instance, Qu et al. used the LNP system to prepare circRNA vaccines for the treatment of SARS-CoV-2 and its emerging variants[122]. The nano-delivery carrier LNPs are low-cost, highly stable, and can be produced on a large scale. However, some disadvantages of LNPs, including their tendency to accumulate in the liver and their limited extrahepatic application, should not be overlooked[123-125]. Xu et al. used tumor-tailored ionizable H1L1A1B3 LNPs to facilitate the delivery of IL-12 circRNAs, inducing a robust immune response in a Lewis lung carcinoma model and marked tumor regression, thereby broadening the prospects for circRNAs drug delivery in cancer therapy[126]. Mitochondria-targeting nanoparticle (Mito-NP) is a multifunctional encapsulated LNP that precisely delivers circSCAR to mitochondria in liver fibroblasts for the treatment of nonalcoholic steatohepatitis[127]. Exosomes, endogenous extracellular vehicles secreted by most cells, are emerging as promising drug delivery vehicles[128-132]. Yu et al. constructed rabies virus glycoprotein-circDYM-extracellular vesicles for targeted delivery of circRNAs across the blood-brain barrier, which inhibited microglial activation, reduced peripheral immune cell infiltration, and attenuated astrocyte dysfunction induced by chronic unpredictable stress[133]. Yang et al. demonstrated that engineered rabies virus glycoprotein-circSCMH1-extracellular vesicles promote functional recovery in rodent and primate ischemic stroke models, highlighting a promising clinical treatment strategy for stroke[134].
CONCLUSIONS AND PERSPECTIVES
Cancer drug resistance significantly limits the effectiveness of clinical cancer treatment, which has been a major focus of research. Considering that aberrant circRNAs contribute to drug resistance in multiple cancers, a systematic understanding of circRNAs in cancer drug resistance can help identify new therapeutic targets. We summarize several circRNAs that are aberrantly expressed during cancer treatment and discuss current knowledge of how circRNAs modulate cancer drug resistance through various underlying mechanisms. For example, circPVT1 promotes chemotherapy resistance in lung adenocarcinoma via miR-145-5p/ABCC1 axis, while it also decreases the radiosensitivity in non-small cell lung cancer cells by modulating the miR-1208/PI3K/AKT/mTOR signaling axis[56,57]. Identifying and constructing circRNAs-mediated regulatory networks could offer a potential approach to overcome cancer drug resistance.
mRNA-based drugs and therapeutics have been successfully applied in clinical practice[122,126,134]. However, their widespread use of mRNA drugs and therapeutics is still limited by factors such as low stability, short expression duration, and potential immunogenicity[122,126,134]. It is noteworthy that circRNAs possess several advantages, including high stability and being immunosilent. As shown in Figure 1, several circRNAs with aberrant expression can serve as biomarkers for various diseases. CircRNAs can also modulate the immune response, affecting how the body fights against infections and diseases. Some circRNAs have the potential to encode small peptides, which hold diverse biological functions. Moreover, antisense circRNAs may regulate gene expression by interacting with complementary RNA sequences or specific proteins, while their binding capacity could also potentially extend protein expression duration by delaying molecular degradation. Zhu et al. have revealed that gut microbiota can regulate tumor metastasis through circRNA/miRNA networks[135]. This circRNA/miRNA-dependent regulatory mechanism not only deepens our understanding of cancer progression, but also provides a theoretical foundation for future clinical interventions targeting gut microbiota. Notably, the back-spliced junction site of circRNAs plays vital roles in the identification and application of circRNAs. The putative back-spliced junction fragments of circRNAs can be amplified with divergent primers and confirmed by Sanger sequencing[45]. To reduce the impact on the corresponding linear transcripts, RNA interferences against circRNAs are usually designed to target the back-spliced junction site of circRNAs.
Multiple circRNAs are associated with the prognosis of cancer therapy, emerging as promising biomarkers or intervention targets for cancer drug resistance. For instance, an upregulation of nearly 138 circRNAs and a downregulation of 86 circRNAs have been observed in chemotherapy-resistant small cell lung cancer cells, indicating that circRNAs play critical roles in the modulation of cancer resistance[47]. Tang et al. discovered that circPPAPDC1A decreases the susceptibility of non-small cell lung cancer cells to osimertinib via the miR-30a-3p/IGF1R axis, suggesting that circPPAPDC1A may be an appropriate candidate for the treatment of osimertinib-resistant non-small cell lung cancer cases[58]. In another study by Li et al., the circITGB6/IGF2BP2/FGF9 RNA-protein complex enhances cisplatin resistance in ovarian cancer cells by facilitating the polarization of tissue-associated macrophages toward M2 macrophages, providing a clear perspective for the treatment of cisplatin-resistant ovarian cancer patients[59].
Although the role of circRNAs in cancer drug resistance in physiological and pathological conditions has been well established, the clinical translation of circRNAs is still in its infancy. Synthesis, purification, delivery, and quality control remain significant challenges for circRNAs-based drugs. While advances have been made in circRNAs-based therapeutics[136], several challenges, including synthesis efficiency, delivery precision, pharmacokinetics, and biodistribution of circRNAs, remain to be addressed. Advances in emerging technologies, such as AI-based bioinformatic algorithms and integrated databases associated with drug resistance, have shown a significant role in improving the rational design, synthesis, and translation efficiency of circRNAs, as well as their application in overcoming tumor drug resistance. AI-based bioinformatics algorithms can assist researchers in identifying novel internal ribosome entry site (IRES) elements and optimize 5’ UTRs, ultimately enhancing circRNA translation efficiency[137]. Several databases have integrated both experimentally validated and computationally predicted ncRNAs associated with drug resistance, aiding the screening and identification of circRNAs linked to cancer drug resistance[138,139]. Expanding the depth of databases on circRNAs, as well as further innovation in AI-based bioinformatics algorithms, are essential to broaden the applicability of circRNAs.
In summary, circRNAs are promising regulators that mediate cancer drug resistance, while further multidisciplinary research is needed to maximize their potential in clinical therapeutic applications. Although circRNAs hold potential in modulating cancer treatment resistance mechanisms, their clinical application necessitates further multidisciplinary research to optimize target selection, delivery systems, and therapeutic validation across diverse tumor microenvironments.
DECLARATIONS
Acknowledgments
Figures were created by Figdraw (ID: AYOSObaaa9; ID: SSOIA45f56).
Authors’ contributions
Wrote the manuscript: Hua J, Zhao P
Responsible for generating the figures: Wang Z, Cheng X, Dai J
All authors read and approved the final manuscript.
Availability of data and materials
Not applicable.
Financial support and sponsorship
This study was supported by the Natural Science Foundation of the Anhui Education Department (2023AH050680) and the Clinical Medicine Training Program of Anhui Medical University (2023-ZQKY-145).
Conflicts of interest
All authors declared that there are no conflicts of interest.
Ethics approval and consent to participate
Not applicable.
Consent for publication
Not applicable.
Copyright
© The Author(s) 2025.
REFERENCES
1. Zhou N, Chen J, Ling Z, et al. Aryl hydrocarbon receptor sulfenylation promotes glycogenolysis and rescues cancer chemoresistance. J Clin Invest. 2023;133:e170753.
2. Qiu H, Cao S, Xu R. Cancer incidence, mortality, and burden in China: a time-trend analysis and comparison with the United States and United Kingdom based on the global epidemiological data released in 2020. Cancer Commun. 2021;41:1037-48.
3. Jensen JL, Bobek O, Chan ICC, et al. Clonal hematopoiesis and clinical outcomes in metastatic castration-resistant prostate cancer patients given androgen receptor pathway inhibitors (Alliance A031201). Clin Cancer Res. 2024;30:4910-9.
4. Qiu C, Tang C, Tang Y, et al. RGS5+ lymphatic endothelial cells facilitate metastasis and acquired drug resistance of breast cancer through oxidative stress-sensing mechanism. Drug Resist Updat. 2024;77:101149.
5. Bordone R, Ivy DM, D’Amico R, et al. MYC upstream region orchestrates resistance to PI3K inhibitors in cancer cells through FOXO3a-mediated autophagic adaptation. Oncogene. 2024;43:3349-65.
6. Zhou Z, Luo W, Zheng C, et al. Mitochondrial metabolism blockade nanoadjuvant reversed immune-resistance microenvironment to sensitize albumin-bound paclitaxel-based chemo-immunotherapy. Acta Pharm Sin B. 2024;14:4087-101.
7. Barnieh FM, Morais GR, Loadman PM, Falconer RA, El-Khamisy SF. Hypoxia-responsive prodrug of ATR inhibitor, AZD6738, selectively eradicates treatment-resistant cancer cells. Adv Sci. 2024;11:e2403831.
8. Kristensen LS, Jakobsen T, Hager H, Kjems J. The emerging roles of circRNAs in cancer and oncology. Nat Rev Clin Oncol. 2022;19:188-206.
9. Wang X, Hua J, Li J, et al. Mechanisms of non-coding RNA-modulated alternative splicing in cancer. RNA Biol. 2022;19:541-7.
10. Chen LL. The expanding regulatory mechanisms and cellular functions of circular RNAs. Nat Rev Mol Cell Biol. 2020;21:475-90.
11. Peng D, Wei C, Jing B, Yu R, Zhang Z, Han L. A novel protein encoded by circCOPA inhibits the malignant phenotype of glioblastoma cells and increases their sensitivity to temozolomide by disrupting the NONO-SFPQ complex. Cell Death Dis. 2024;15:616.
12. Tang X, Guo M, Zhang Y, Lv J, Gu C, Yang Y. Examining the evidence for mutual modulation between m6A modification and circular RNAs: current knowledge and future prospects. J Exp Clin Cancer Res. 2024;43:216.
13. Zhou Y, Wei C, Xu Y, Wang J, Zhang C, Jin Y. Exosomal circRNAs: a future star in colorectal cancer. Curr Cancer Drug Targets. 2024; doi: 10.2174/0115680096323472240710101854.
14. Xu T, He B, Sun H, et al. Novel insights into the interaction between N6-methyladenosine modification and circular RNA. Mol Ther Nucleic Acids. 2022;27:824-37.
15. Chen L, Huang C, Shan G. Circular RNAs in physiology and non-immunological diseases. Trends Biochem Sci. 2022;47:250-64.
16. Li B, Zhu L, Lu C, et al. circNDUFB2 inhibits non-small cell lung cancer progression via destabilizing IGF2BPs and activating anti-tumor immunity. Nat Commun. 2021;12:295.
17. Wang C, Liu WR, Tan S, et al. Characterization of distinct circular RNA signatures in solid tumors. Mol Cancer. 2022;21:63.
18. Xu C, Jun E, Okugawa Y, et al. A circulating panel of circRNA biomarkers for the noninvasive and early detection of pancreatic ductal adenocarcinoma. Gastroenterology. 2024;166:178-90.e16.
19. Xie F, Liu S, Wang J, et al. deepBase v3.0: expression atlas and interactive analysis of ncRNAs from thousands of deep-sequencing data. Nucleic Acids Res. 2021;49:D877-83.
20. Ding N, You AB, Yang H, et al. A tumor-suppressive molecular axis EP300/circRERE/miR-6837-3p/MAVS activates type I IFN pathway and antitumor immunity to suppress colorectal cancer. Clin Cancer Res. 2023;29:2095-109.
21. Li J, Wang X, Shi L, et al. A mammalian conserved circular RNA circLARP1B regulates hepatocellular carcinoma metastasis and lipid metabolism. Adv Sci. 2024;11:e2305902.
22. Gao X, Xia X, Li F, et al. Circular RNA-encoded oncogenic E-cadherin variant promotes glioblastoma tumorigenicity through activation of EGFR-STAT3 signalling. Nat Cell Biol. 2021;23:278-91.
23. Fu M, Gao Q, Xiao M, et al. Extracellular vesicles containing circMYBL1 induce CD44 in adenoid cystic carcinoma cells and pulmonary endothelial cells to promote lung metastasis. Cancer Res. 2024;84:2484-500.
24. Wong CH, Lou UK, Fung FK, et al. CircRTN4 promotes pancreatic cancer progression through a novel CircRNA-miRNA-lncRNA pathway and stabilizing epithelial-mesenchymal transition protein. Mol Cancer. 2022;21:10.
25. Yang Y, Luo D, Shao Y, et al. circCAPRIN1 interacts with STAT2 to promote tumor progression and lipid synthesis via upregulating ACC1 expression in colorectal cancer. Cancer Commun. 2023;43:100-22.
26. Huang B, Ren J, Ma Q, et al. A novel peptide PDHK1-241aa encoded by circPDHK1 promotes ccRCC progression via interacting with PPP1CA to inhibit AKT dephosphorylation and activate the AKT-mTOR signaling pathway. Mol Cancer. 2024;23:34.
27. Ma Q, Yang F, Huang B, et al. CircARID1A binds to IGF2BP3 in gastric cancer and promotes cancer proliferation by forming a circARID1A-IGF2BP3-SLC7A5 RNA-protein ternary complex. J Exp Clin Cancer Res. 2022;41:251.
28. Du J, Lan T, Liao H, et al. CircNFIB inhibits tumor growth and metastasis through suppressing MEK1/ERK signaling in intrahepatic cholangiocarcinoma. Mol Cancer. 2022;21:18.
29. Xie M, Yu T, Jing X, et al. Exosomal circSHKBP1 promotes gastric cancer progression via regulating the miR-582-3p/HUR/VEGF axis and suppressing HSP90 degradation. Mol Cancer. 2020;19:112.
30. Li R, Tian X, Jiang J, Qian H, Shen H, Xu W. CircRNA CDR1as: a novel diagnostic and prognostic biomarker for gastric cancer. Biomarkers. 2023;28:448-57.
31. He J, Lv X, Zeng Z. A potential disease monitoring and prognostic biomarker in cervical cancer patients: the clinical application of circular RNA_0018289. J Clin Lab Anal. 2020;34:e23340.
32. Tian M, Chen R, Li T, Xiao B. Reduced expression of circRNA hsa_circ_0003159 in gastric cancer and its clinical significance. J Clin Lab Anal. 2018;32:e22281.
33. Yao JT, Zhao SH, Liu QP, et al. Over-expression of CircRNA_100876 in non-small cell lung cancer and its prognostic value. Pathol Res Pract. 2017;213:453-6.
34. Zhang X, Wang S, Wang H, et al. Circular RNA circNRIP1 acts as a microRNA-149-5p sponge to promote gastric cancer progression via the AKT1/mTOR pathway. Mol Cancer. 2019;18:20.
35. Chen SW, Zhu SQ, Pei X, et al. Cancer cell-derived exosomal circUSP7 induces CD8+ T cell dysfunction and anti-PD1 resistance by regulating the miR-934/SHP2 axis in NSCLC. Mol Cancer. 2021;20:144.
36. Liu J, Kong L, Bian W, Lin X, Wei F, Chu J. circRNA_0001006 predicts prognosis and regulates cellular processes of triple-negative breast cancer via miR-424-5p. Cell Div. 2023;18:7.
37. Wang X, Zhang Y, Huang L, et al. Decreased expression of hsa_circ_001988 in colorectal cancer and its clinical significances. Int J Clin Exp Pathol. 2015;8:16020-5.
38. Ding M, Dai D, Yang W, Geng C, Cui G. Has_circ_0048764 promotes breast cancer progression by sponging miR-578 and regulating HMGA2 expression. Nucleosides Nucleotides Nucleic Acids. 2023;42:448-63.
39. Dhanyamraju PK. Drug resistance mechanisms in cancers: execution of pro-survival strategies. J Biomed Res. 2024;38:95-121.
40. Xie R, Cheng L, Huang M, et al. NAT10 drives cisplatin chemoresistance by enhancing ac4C-associated DNA repair in bladder cancer. Cancer Res. 2023;83:1666-83.
41. Tang H, Huang X, Wang J, et al. circKIF4A acts as a prognostic factor and mediator to regulate the progression of triple-negative breast cancer. Mol Cancer. 2019;18:23.
42. You X, Guo W, Wang L, et al. Subcellular distribution of RAD23B controls XPC degradation and DNA damage repair in response to chemotherapy drugs. Cell Signal. 2017;36:108-16.
43. Wang X, Zhang J, Cao G, Hua J, Shan G, Lin W. Emerging roles of circular RNAs in gastric cancer metastasis and drug resistance. J Exp Clin Cancer Res. 2022;41:218.
44. Gong J, Han G, Chen Z, et al. CircDCAF8 promotes the progression of hepatocellular carcinoma through miR-217/NAP1L1 axis, and induces angiogenesis and regorafenib resistance via exosome-mediated transfer. J Transl Med. 2024;22:517.
45. Hua J, Wang X, Ma L, et al. CircVAPA promotes small cell lung cancer progression by modulating the miR-377-3p and miR-494-3p/IGF1R/AKT axis. Mol Cancer. 2022;21:123.
46. Zhang P, Sun H, Wen P, Wang Y, Cui Y, Wu J. circRNA circMED27 acts as a prognostic factor and mediator to promote lenvatinib resistance of hepatocellular carcinoma. Mol Ther Nucleic Acids. 2022;27:293-303.
47. Huang W, Yang Y, Wu J, et al. Circular RNA cESRP1 sensitises small cell lung cancer cells to chemotherapy by sponging miR-93-5p to inhibit TGF-β signalling. Cell Death Differ. 2020;27:1709-27.
48. Lin J, Lyu Z, Feng H, et al. CircPDIA3/miR-449a/XBP1 feedback loop curbs pyroptosis by inhibiting palmitoylation of the GSDME-C domain to induce chemoresistance of colorectal cancer. Drug Resist Updat. 2024;76:101097.
49. Pan Z, Zheng J, Zhang J, et al. A novel protein encoded by exosomal circATG4B induces oxaliplatin resistance in colorectal cancer by promoting autophagy. Adv Sci. 2022;9:e2204513.
50. Wang X, Cao Q, Shi Y, et al. Identification of low-dose radiation-induced exosomal circ-METRN and miR-4709-3p/GRB14/PDGFRα pathway as a key regulatory mechanism in Glioblastoma progression and radioresistance: functional validation and clinical theranostic significance. Int J Biol Sci. 2021;17:1061-78.
51. Qu H, Wang Y, Yan Q, et al. CircCDYL2 bolsters radiotherapy resistance in nasopharyngeal carcinoma by promoting RAD51 translation initiation for enhanced homologous recombination repair. J Exp Clin Cancer Res. 2024;43:122.
52. Lin L, Hu P, Luo M, et al. CircNOP14 increases the radiosensitivity of hepatocellular carcinoma via inhibition of Ku70-dependent DNA damage repair. Int J Biol Macromol. 2024;264:130541.
53. Zhang Y, Liu Z, Zhong Z, et al. A tumor suppressor protein encoded by circKEAP1 inhibits osteosarcoma cell stemness and metastasis by promoting vimentin proteasome degradation and activating anti-tumor immunity. J Exp Clin Cancer Res. 2024;43:52.
54. Chen DL, Chen N, Sheng H, Zhang DS. Circular RNA circNCOA3 promotes tumor progression and anti-PD-1 resistance in colorectal cancer. Cancer Drug Resist. 2024;7:9.
55. Hu Z, Chen G, Zhao Y, et al. Exosome-derived circCCAR1 promotes CD8+ T-cell dysfunction and anti-PD1 resistance in hepatocellular carcinoma. Mol Cancer. 2023;22:55.
56. Zheng F, Xu R. CircPVT1 contributes to chemotherapy resistance of lung adenocarcinoma through miR-145-5p/ABCC1 axis. Biomed Pharmacother. 2020;124:109828.
57. Huang M, Li T, Wang Q, et al. Silencing circPVT1 enhances radiosensitivity in non-small cell lung cancer by sponging microRNA-1208. Cancer Biomark. 2021;31:263-79.
58. Tang YF, Liu ZH, Zhang LY, et al. circ_PPAPDC1A promotes Osimertinib resistance by sponging the miR-30a-3p/ IGF1R pathway in non-small cell lung cancer (NSCLC). Mol Cancer. 2024;23:91.
59. Li H, Luo F, Jiang X, et al. CircITGB6 promotes ovarian cancer cisplatin resistance by resetting tumor-associated macrophage polarization toward the M2 phenotype. J Immunother Cancer. 2022;10:e004029.
60. Marino M, Cannarella R, Condorelli RA, Crafa A, La Vignera S, Calogero AE. New insights of target therapy: effects of tyrosine kinase inhibitors on male gonadal function: a systematic review. Clin Genitourin Cancer. 2024;22:102131.
61. Bricker-Anthony R, Koeberl DD, Lipshutz GS, Perna F. Staying on target in gene and cell therapy. Mol Ther. 2024;32:2801-2.
62. Yang WC, Koto M, Ikawa H, et al. Clinical target volume design and dose in carbon-ion radiation therapy for sinonasal mucosal melanoma. Radiother Oncol. 2024;200:110511.
63. Bian Y, Shan G, Bi G, et al. Targeting ALDH1A1 to enhance the efficacy of KRAS-targeted therapy through ferroptosis. Redox Biol. 2024;77:103361.
64. Varshney P, Sharma V, Yadav D, et al. The impacts and changes related to the cancer drug resistance mechanism. Curr Drug Metab. 2023;24:787-802.
65. Adenis A, Ghiringhelli F, Gauthier L, et al. Regorafenib plus FOLFIRINOX as first-line treatment for patients with RAS-mutant metastatic colorectal cancer (FOLFIRINOX-R trial): a dose-escalation study. Cancer Chemother Pharmacol. 2024;94:443-52.
66. Kim HD, Jung S, Lim HY, et al. Regorafenib plus nivolumab in unresectable hepatocellular carcinoma: the phase 2 RENOBATE trial. Nat Med. 2024;30:699-707.
67. Zheng Y, Ye S, Huang S, et al. Lefamulin overcomes acquired drug resistance via regulating mitochondrial homeostasis by targeting ILF3 in hepatocellular carcinoma. Adv Sci. 2024;11:e2401789.
68. Chang MH, Lee J, Han J, et al. Prognostic role of insulin-like growth factor receptor-1 expression in small cell lung cancer. APMIS. 2009;117:861-9.
69. Tognon CE, Sorensen PH. Targeting the insulin-like growth factor 1 receptor (IGF1R) signaling pathway for cancer therapy. Expert Opin Ther Targets. 2012;16:33-48.
70. Yang Z, Wang X, Fu Y, et al. YTHDF2 in peritumoral hepatocytes mediates chemotherapy-induced antitumor immune responses through CX3CL1-mediated CD8+ T cell recruitment. Mol Cancer. 2024;23:186.
71. Low K, Foulkes P, Hills F, Roberts HC, Stordal B. The efficacy of gemcitabine and docetaxel chemotherapy for the treatment of relapsed and refractory osteosarcoma: a systematic review and pre-clinical study. Cancer Med. 2024;13:e70248.
72. Liang T, Dong Y, Cheng I, et al. In situ formation of biomolecular condensates as intracellular drug reservoirs for augmenting chemotherapy. Nat Biomed Eng. 2024;8:1469-82.
73. Han X, Guo J, Li L, et al. Sintilimab combined with anlotinib and chemotherapy as second-line or later therapy in extensive-stage small cell lung cancer: a phase II clinical trial. Signal Transduct Target Ther. 2024;9:241.
74. Borek WE, Nobre L, Pedicona SF, et al. Phosphoproteomics predict response to midostaurin plus chemotherapy in independent cohorts of FLT3-mutated acute myeloid leukaemia. EBioMedicine. 2024;108:105316.
75. Dugo M, Huang CS, Egle D, et al. The immune-related 27-gene signature DetermaIO predicts response to neoadjuvant atezolizumab plus chemotherapy in triple-negative breast cancer. Clin Cancer Res. 2024;30:4900-9.
76. Akkus E, Arslan Ç, Ürün Y. Advancements in platinum chemotherapy for metastatic castration-resistant prostate cancer: insights and perspectives. Cancer Treat Rev. 2024;130:102818.
77. Ying Q, Fan R, Shen Y, et al. Small cell lung cancer-an update on chemotherapy resistance. Curr Treat Options Oncol. 2024;25:1112-23.
78. Lim JKM, Samiei A, Delaidelli A, et al. The eEF2 kinase coordinates the DNA damage response to cisplatin by supporting p53 activation. Cell Death Dis. 2024;15:501.
79. Mann J, Niedermayer K, Krautstrunk J, et al. Combined inhibition of RAD51 and CHK1 causes synergistic toxicity in cisplatin resistant cancer cells by triggering replication fork collapse. Int J Cancer. 2025;156:389-402.
80. Wang P, Ye Y, Chen Z, Li R, Hou G, Liu Z. PFKL promotes cell viability and glycolysis and inhibits cisplatin chemosensitivity of laryngeal squamous cell carcinoma. Biochem Biophys Res Commun. 2024;730:150366.
81. Lu H, Tong W, Jiang M, et al. Mitochondria-targeted multifunctional nanoprodrugs by inhibiting metabolic reprogramming for combating cisplatin-resistant lung cancer. ACS Nano. 2024;18:21156-70.
82. Xiao L, Zhu Y, Xu H, Lin L, Li M, Zhou Y. Circ_0000395 promotes cell growth, metastasis and oxaliplatin resistance by regulating miR-153-5p/MYO6 in colorectal cancer. Pathol Res Pract. 2024;260:155476.
83. Liu D, Wu L, Xu X, et al. Regimens combining radiation and immunotherapy for cancer: latest updates from 2024 ASCO Annual Meeting. J Hematol Oncol. 2024;17:84.
84. Hircock C, Lee SF, Raman S, et al. Bridging the care gap: radiation therapy in elderly and frail cancer patients. Curr Opin Support Palliat Care. 2024;18:276-81.
85. Feng D, Yan Z, Fu B, et al. Phase II study of pegaspargase, etoposide, gemcitabine (PEG) followed by involved-field radiation therapy in early-stage extranodal natural killer/T-cell lymphoma. Hematology. 2024;29:2402102.
86. Sharma D, Khosla D, Meena BL, Yadav HP, Kapoor R. Exploring the evolving landscape of stereotactic body radiation therapy in hepatocellular carcinoma. J Clin Exp Hepatol. 2025;15:102386.
87. Liu K, Hoover AR, Wang L, et al. Localized ablative immunotherapy enhances antitumor immunity by modulating the transcriptome of tumor-infiltrating Gamma delta T cells. Cancer Lett. 2024;604:217267.
88. Li C, Yu X, Han X, et al. Innate immune cells in tumor microenvironment: a new frontier in cancer immunotherapy. iScience. 2024;27:110750.
89. Chen S, Yao C, Tian N, et al. The interplay between persistent pathogen infections with tumor microenvironment and immunotherapy in cancer. Cancer Med. 2024;13:e70154.
90. Liu F, Zhuang W, Song B, et al. MAVS-loaded unanchored Lys63-linked polyubiquitin chains activate the RIG-I-MAVS signaling cascade. Cell Mol Immunol. 2023;20:1186-202.
91. Zhao H, Zhang L, Du D, et al. The RIG-I-like receptor signaling pathway triggered by Staphylococcus aureus promotes breast cancer metastasis. Int Immunopharmacol. 2024;142:113195.
92. Li J, Feng H, Zhu J, et al. Gastric cancer derived exosomal THBS1 enhanced Vγ9Vδ2 T-cell function through activating RIG-I-like receptor signaling pathway in a N6-methyladenosine methylation dependent manner. Cancer Lett. 2023;576:216410.
93. He QQ, Huang Y, Nie L, et al. MAVS integrates glucose metabolism and RIG-I-like receptor signaling. Nat Commun. 2023;14:5343.
94. Bai M, Wang WX, Deng T, Duan JJ, Ba Y. Feasibility and safety of PD-1 blockades among elderly patients with metastatic esophageal squamous cell carcinoma: a real-world study. Drug Des Devel Ther. 2024;18:4135-51.
95. Yan M, Chen X, Li X, et al. Transferrin receptor-targeted immunostimulant for photodynamic immunotherapy against metastatic tumors through β-catenin/CREB interruption. Acta Pharm Sin B. 2024;14:4118-33.
96. Singh A, Mommers-Elshof ETAM, Vijver SV, et al. Leukocyte-associated immunoglobulin-like receptor-1 blockade in combination with programmed death-ligand 1 targeting therapy mediates increased tumour control in mice. Cancer Immunol Immunother. 2024;73:16.
97. Zou Y, Yang A, Chen B, et al. crVDAC3 alleviates ferroptosis by impeding HSPB1 ubiquitination and confers trastuzumab deruxtecan resistance in HER2-low breast cancer. Drug Resist Updat. 2024;77:101126.
98. Yang R, Cui J. Advances and applications of RNA vaccines in tumor treatment. Mol Cancer. 2024;23:226.
99. Li J, Zhou W, Wang H, Huang M, Deng H. Exosomal circular RNAs in tumor microenvironment: an emphasis on signaling pathways and clinical opportunities. MedComm. 2024;5:e70019.
100. Huang X, Song C, Zhang J, Zhu L, Tang H. Circular RNAs in breast cancer diagnosis, treatment and prognosis. Oncol Res. 2023;32:241-9.
101. Cao X, Zhou X, Hou F, et al. ncRNADrug: a database for validated and predicted ncRNAs associated with drug resistance and targeted by drugs. Nucleic Acids Res. 2024;52:D1393-9.
102. Xie J, Ye F, Deng X, et al. Circular RNA: a promising new star of vaccine. J Transl Int Med. 2023;11:372-81.
103. Loan Young T, Chang Wang K, James Varley A, Li B. Clinical delivery of circular RNA: lessons learned from RNA drug development. Adv Drug Deliv Rev. 2023;197:114826.
104. Deng J, Liao S, Chen C, et al. Specific intracellular retention of circSKA3 promotes colorectal cancer metastasis by attenuating ubiquitination and degradation of SLUG. Cell Death Dis. 2023;14:750.
105. Xu J, Ji L, Liang Y, et al. CircRNA-SORE mediates sorafenib resistance in hepatocellular carcinoma by stabilizing YBX1. Signal Transduct Target Ther. 2020;5:298.
106. Lee KH, Kim S, Song J, Han SR, Kim JH, Lee SW. Efficient circular RNA engineering by end-to-end self-targeting and splicing reaction using Tetrahymena group I intron ribozyme. Mol Ther Nucleic Acids. 2023;33:587-98.
107. Feng Z, Zhang X, Zhou J, et al. An in vitro-transcribed circular RNA targets the mitochondrial inner membrane cardiolipin to ablate EIF4G2+/PTBP1+ pan-adenocarcinoma. Nat Cancer. 2024;5:30-46.
108. Zhang Z, Fu Y, Ju X, Zhang F, Zhang P, He M. Advances in engineering circular RNA vaccines. Pathogens. 2024;13:692.
109. Chen R, Wang SK, Belk JA, et al. Engineering circular RNA for enhanced protein production. Nat Biotechnol. 2023;41:262-72.
110. Cheng M, Yu X, Qi S, et al. Development of organ targeting lipid nanoparticles with low immunogenicity and their application in the treatment of pulmonary fibrosis. Angew Chem Int Ed Engl. 2024;63:e202407398.
111. Ferraresso F, Badior K, Seadler M, et al. Protein is expressed in all major organs after intravenous infusion of mRNA-lipid nanoparticles in swine. Mol Ther Methods Clin Dev. 2024;32:101314.
112. Rouatbi N, Walters AA, Costa PM, et al. RNA lipid nanoparticles as efficient in vivo CRISPR-Cas9 gene editing tool for therapeutic target validation in glioblastoma cancer stem cells. J Control Release. 2024;375:776-87.
113. Palanki R, Riley JS, Bose SK, et al. In utero delivery of targeted ionizable lipid nanoparticles facilitates in vivo gene editing of hematopoietic stem cells. Proc Natl Acad Sci U S A. 2024;121:e2400783121.
114. Yang H, Lv D, Qu S, et al. A ROS-responsive lipid nanoparticles release multifunctional hydrogel based on microenvironment regulation promotes infected diabetic wound healing. Adv Sci. 2024;11:e2403219.
115. Varshney K, Mazumder R, Rani A, Pandey P, Ravindra Babu M. Liquid crystalline lipid nanoparticles: emerging trends and applications in skin cancer. Pharm Nanotechnol. 2024; doi: 10.2174/0122117385312450240816055942.
116. Rong J, Liu T, Yin X, et al. Co-delivery of camptothecin and MiR-145 by lipid nanoparticles for MRI-visible targeted therapy of hepatocellular carcinoma. J Exp Clin Cancer Res. 2024;43:247.
117. Wang D, Zhang H, Liao X, et al. Oral administration of Robinia pseudoacacia L. flower exosome-like nanoparticles attenuates gastric and small intestinal mucosal ferroptosis caused by hypoxia through inhibiting HIF-1α- and HIF-2α-mediated lipid peroxidation. J Nanobiotechnology. 2024;22:479.
118. Kassaee SN, Richard D, Ayoko GA, Islam N. Lipid polymer hybrid nanoparticles against lung cancer and their application as inhalable formulation. Nanomedicine. 2024;19:2113-33.
119. Wu W, Zhang J, Cao X, Cai Z, Zhao F. Exploring the cellular landscape of circular RNAs using full-length single-cell RNA sequencing. Nat Commun. 2022;13:3242.
120. Xu C, Zhang L, Wang W, et al. Improving the circularization efficiency, stability and translatability of circular RNA by circDesign. bioRxiv 2023. Available from: https://www.biorxiv.org/content/10.1101/2023.07.09.548293v1. [accessed 26 Mar 2025]
121. Wesselhoeft RA, Kowalski PS, Anderson DG. Engineering circular RNA for potent and stable translation in eukaryotic cells. Nat Commun. 2018;9:2629.
122. Qu L, Yi Z, Shen Y, et al. Circular RNA vaccines against SARS-CoV-2 and emerging variants. Cell. 2022;185:1728-44.e16.
123. Kim M, Jeong M, Hur S, et al. Engineered ionizable lipid nanoparticles for targeted delivery of RNA therapeutics into different types of cells in the liver. Sci Adv. 2021;7:eabf4398.
124. Jung HN, Lee SY, Lee S, Youn H, Im HJ. Lipid nanoparticles for delivery of RNA therapeutics: current status and the role of in vivo imaging. Theranostics. 2022;12:7509-31.
125. Saber N, Senti ME, Schiffelers RM. Lipid nanoparticles for nucleic acid delivery beyond the liver. Hum Gene Ther. 2024;35:617-27.
126. Xu S, Xu Y, Solek NC, et al. Tumor-tailored ionizable lipid nanoparticles facilitate IL-12 circular RNA delivery for enhanced lung cancer immunotherapy. Adv Mater. 2024;36:e2400307.
127. Zhao Q, Liu J, Deng H, et al. Targeting mitochondria-located circRNA SCAR alleviates NASH via reducing mROS output. Cell. 2020;183:76-93.e22.
128. Huang G, Zheng W, Zhou Y, Wan M, Hu T. Recent advances to address challenges in extracellular vesicle-based applications for lung cancer. Acta Pharm Sin B. 2024;14:3855-75.
129. Rehman FU, Liu Y, Zheng M, Shi B. Exosomes based strategies for brain drug delivery. Biomaterials. 2023;293:121949.
130. de Voogt WS, Tanenbaum ME, Vader P. Illuminating RNA trafficking and functional delivery by extracellular vesicles. Adv Drug Deliv Rev. 2021;174:250-64.
131. Kronstadt SM, Jay SM, Jeyaram A. Extracellular vesicle loading via pH-gradient modification. In: Federico M, Ridolfi B, editors. Extracellular vesicles in diagnosis and therapy. New York: Springer US; 2022. pp. 231-9.
132. Chao F, Zhang Y, Lv L, et al. Extracellular vesicles derived circSH3PXD2A inhibits chemoresistance of small cell lung cancer by miR-375-3p/YAP1. Int J Nanomedicine. 2023;18:2989-3006.
133. Yu X, Bai Y, Han B, et al. Extracellular vesicle-mediated delivery of circDYM alleviates CUS-induced depressive-like behaviours. J Extracell Vesicles. 2022;11:e12185.
134. Yang L, Han B, Zhang Z, et al. Extracellular vesicle-mediated delivery of circular RNA SCMH1 promotes functional recovery in rodent and nonhuman primate ischemic stroke models. Circulation. 2020;142:556-74.
135. Zhu Z, Huang J, Li X, et al. Gut microbiota regulate tumor metastasis via circRNA/miRNA networks. Gut Microbes. 2020;12:1788891.
136. Liu CX, Chen LL. Circular RNAs: characterization, cellular roles, and applications. Cell. 2022;185:2016-34.
138. Zhang H, Zhang L, Lin A, et al. Algorithm for optimized mRNA design improves stability and immunogenicity. Nature. 2023;621:396-403.
Cite This Article
How to Cite
Download Citation
Export Citation File:
Type of Import
Tips on Downloading Citation
Citation Manager File Format
Type of Import
Direct Import: When the Direct Import option is selected (the default state), a dialogue box will give you the option to Save or Open the downloaded citation data. Choosing Open will either launch your citation manager or give you a choice of applications with which to use the metadata. The Save option saves the file locally for later use.
Indirect Import: When the Indirect Import option is selected, the metadata is displayed and may be copied and pasted as needed.
About This Article
Copyright
Data & Comments
Data
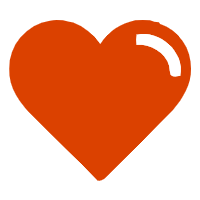
Comments
Comments must be written in English. Spam, offensive content, impersonation, and private information will not be permitted. If any comment is reported and identified as inappropriate content by OAE staff, the comment will be removed without notice. If you have any queries or need any help, please contact us at [email protected].