Role of MeCP2 in oligodendrocyte lineage cells in Rett syndrome: review and inference
Abstract
Rett syndrome (RTT) is a neurodevelopmental disorder primarily caused by mutations in the MECP2 gene. Neuronal damage is the main factor contributing to RTT, and the loss of MeCP2 function can result in reduced neuronal somas size, decreased dendritic abundance, and impaired neuronal function. While specific restoration of MeCP2 expression in neurons has been reported to partially rescue the behavioral phenotype and prolong the lifespan of mice, it cannot provide a complete cure. Therefore, other cells may be involved in the development of RTT. Although imaging and autopsy findings have revealed decreased white matter volume and corpus callosum thickness in RTT patients, the mechanisms underlying the development of white matter abnormalities remain unclear. These abnormalities are predominantly caused by damage to mature oligodendrocytes. This review provides an overview of the proliferation, differentiation, and function of oligodendrocyte lineage cells and elucidates the role of MeCP2 in these cells.
Keywords
INTRODUCTION
Rett syndrome (RTT) is a severe and rare neurodevelopmental disorder with no effective treatment for its complex pathogenesis[1]. It has a female preponderance (rarely occurs among men), with the incidence rate ranging from 1/10000 to 1/15000 among female patients[2]. RTT is caused by gene mutations and can be classified as classic and non-classic RTT based on the specific mutation involved. Classic RTT results from a loss-of-function mutation in the X-linked methyl CpG-binding protein 2 (MECP2) gene, which encodes the MeCP2 protein. Clinical studies have reported that 90%-95% of cases are attributed to classic RTT[3]. In contrast, non-classic RTT is primarily caused by mutations in the cyclin-dependent kinase-like 5 (CDKL5) and forkhead box G1(FOXG1) genes[4,5]. It exhibits clinical features similar to those of classic RTT, including early-onset epilepsy and developmental delay[6]. Therefore, MeCP2 has become the focus of research on RTT. Hundreds of MECP2 mutation sites that can cause classic RTT have been identified[7,8]. It is noteworthy that large segment deletions and eight hotspot mutations account for approximately 80% of the reported cases[7]. The mutations of MECP2 have been validated in relevant mouse models.
Research on RTT has been primarily focused on the process and function of neurons. Autopsy reports of patients have shown reduced brain volume, neuronal soma size, dendritic arborization, and dendritic spine abundance[7]. Therefore, neurons have become the core of research into the pathological mechanisms of RTT. The abovementioned pathological features commonly observed in neurons have been validated in different mouse models with various mutations[9,10]. In addition, specific restoration of MeCP2 expression in neurons has been shown to restore relevant behaviors and prolong the lifespan of mice[11,12]. However, some phenotypes cannot be restored through this approach, suggesting that MeCP2 in the central nervous system (CNS) may be involved in RTT development through the regulation of other cells[13,14]. In the CNS, MeCP2 can be detected not only in neurons but also in astrocytes, oligodendrocytes (OLs), and microglia. Relevant studies have demonstrated that restoring the expression of MeCP2 in astrocytes, OLs, and microglia can alleviate some symptoms in knockout mouse models, indicating that glial cells also play an indispensable role in RTT[15]. With a deeper understanding of the function and structure of glial cells, novel mechanisms have been proposed to elucidate the development of RTT.
The application of Magnetic Resonance Imaging (MRI) in biological research has facilitated dynamic observation of abnormal brain development in patients. Brain imaging has revealed significant abnormalities in the total volume of white matter (WM) in RTT patients, in addition to gray matter (GM) abnormalities commonly observed on pathological examination[16,17]. WM is primarily connected through WM fiber bundles across the left and right hemispheres and various brain regions, forming a complex WM signal network[18]. WM fiber bundles are primarily composed of axons of neurons and myelin sheaths wrapped around them.
Given that neuronal bodies are not found in WM and only mature OLs can form myelin sheaths in the CNS, we have proposed that MeCP2 leads to WM abnormalities by regulating the abnormal development of OL lineage cells and myelin formation. The OL lineage cells include oligodendrocyte precursor cells (OPCs) and OLs. OPCs undergo a series of biological regulations and eventually differentiate into functionally specific mature OLs at specific locations[19]. In the CNS of mammals, myelin sheaths are exclusively formed by mature OLs[19]. This review summarizes the mechanisms underlying the development of WM abnormalities resulting from MeCP2 dysfunction and explores the role of MeCP2 in regulating cell proliferation, differentiation, and myelin sheath formation in OLs, thereby providing a rational basis for the study of MeCP2 in OLs.
STRUCTURE AND FUNCTION OF MECP2
MeCP2, a member of the methyl-CpG-binding protein family, has two subtypes, MECP2-E1 and MECP2-E2, each containing four exons that encode four structural domains, including the N-terminal domain (NTD), methyl-CpG-binding domain (MBD), transcription repression domain (TRD), and C-terminal domain (CTD)[7]. Analysis of the expression of the two subtypes revealed that the expression levels of E1 in the human and mouse brains were about 10 times higher than E2, but there was no difference in their expression levels in the liver and testes of mice[20,21]. MeCP2 was initially identified as a nuclear protein that binds to methylated DNA, which led to its characterization as a transcriptional repressor[22] [Figure 1] However, recent studies have demonstrated that MeCP2 is involved in various functions, including activation and inhibition of transcription, RNA splicing, and miRNA regulation within the nucleus[23,24].
Figure 1. Characteristics of MECP2 gene and its protein function. Note: (A) MECP2 gene subtypes, functional domains, and common mutation types (eight hotspot mutations). Two isoforms of the MECP2: MECP2-E1 (encoded by exons 1, 3, 4) and MECP2-E2 (encoded by exons 1, 3, 4). Red arrows indicate the transcription start sites (ATG) of the two isoforms. MeCP2 protein structure is composed of five major domains, N-terminal Domain (NTD), Methyl Binding Domain (MBD), Inter-Domain (ID), Transcription Repression Domain (TRD), and C-terminal Domain (CTD). The AT-Hook domain is located in the CTD, which allows binding to adenine-thymine (AT) rich DNA. Eight Hotspot Mutations: R106W (Arginine to Tryptophan), R133C (Arginine to Cystine), T158M (Threonine to Methionine), R168X (Arginine to termination codon), R255X (Arginine to termination codon), R294X (Arginine to termination codon), R270X (Arginine to termination codon), R306C (Arginine to Cystine); (B) Mechanisms through which MeCP2 regulates transcription, RNA splicing, and mRNA cleavage. The MBD and CTD domains of MeCP2 can activate or inhibit transcriptional regulation by binding to DNA. MeCP2 can regulate the alternative splicing of RNA by combining it with YB-1 and other proteins. MeCP2 can also regulate the expression of miRNAs through the interaction with the Dorsha complex. AT: adenine-thymine; CTD: C-terminal Domain; ID: Inter-Domain; MBD: Methyl Binding Domain; NTD: N-terminal Domain; TRD: Transcription Repression Domain.
Mutations in MeCP2
The majority of RTT cases (over 95%) are due to de novo mutations in the MECP2 gene, mainly occurring on the paternal X chromosome[25]. Because the X chromosome of spermatozoa is hypermethylated, the methylated cytosine residue can undergo spontaneous deamination to form thymine[7,26]. Familial inheritance of RTT is rare and is due to X-chromosomal inheritance from a carrier mother[27]. To date, over 500 MECP2 mutations have been identified, including missense mutations, nonsense mutations, insertion mutations, and small or large deletions[28]. Point mutations are the most common mutation types, with C > T being the most frequently observed[29]. Eight single-point mutations, R106W, R133C, T158M, R168X, R255X, R270X, R294X, and R306C, account for about 70% of the reported cases[6] [Figure 1]. R106W, R133C, and T158M are located in the MBD domain of MeCP2, which can affect the methyl binding capacity of MeCP2[30]. Patients with R106W or T158M mutation exhibit severe pathological phenotypes[31,32]. R306C is located in the TRD domain, preventing MeCP2 from binding to the NCoR/SMRT complex, thus affecting transcriptional activation or inhibition[30,33]. Four nonsense mutations, R168X, R255X, R270X, and R294X, give rise to premature stop codons, resulting in truncated MeCP2. R255X, R270X, and R294X are located in the TRD domain[6]. R168X is located in the intervention outcome domain (ID), which can cause the MeCP2 to lose the entire TRD domain[30]. Patients with T168X exhibited the most severe clinical phenotypes, with the majority losing their walking ability[7,34,35]. In addition, the large segment deletion and C-terminal domain deletion of MeCP2 account for about 13% of RTT cases[25]. Large segment deletion often causes more severe clinical phenotypes, whereas the C-terminal domain deletion is relatively mild[25]. Researchers have established various RTT animal models based on different mutation types, which have greatly assisted in understanding the pathogenesis of RTT.
MeCP2 and transcription
MeCP2 was originally known as a methyl-binding protein that regulates transcription [Figure 1]. The MBD of MeCP2 has a strong affinity for CpG-methylated DNA, and the affinity of MeCP2 for chromatin is significantly reduced in MeCP2-deficient cells. Mellén et al. revealed that MeCP2 could bind to 5-hydroxymethylcytosine (5hmc) with an affinity similar to that for 5-methylcytosine (5mc) in actively transcribed genes[36]. However, the biological significance of the binding between MeCP2 and 5hmc remains unclear. In addition to binding to CpG, MeCP2 can bind to methylation sites such as CpA, CpC, and CpH[37,38]. But the implications of this binding warrant further investigation. Lyst et al. demonstrated that MeCP2 acted as a junction protein between DNA and the NCoR/SMRT complex to repress the transcription of target genes[33]. Continuous investigation of the structure and function of MeCP2 has improved the understanding of mechanisms through which MeCP2 regulates transcription.
MeCP2 and RNA splicing
Although MeCP2 can directly regulate gene transcription, recent studies have shown that MeCP2 can regulate gene expression by splicing mRNA [Figure 1]. Young et al. found that truncated MeCP2 can lead to abnormal RNA splicing in Mecp2308/y male mice[39]. They reported that MeCP2 directly interacts with Y box-binding protein 1 (YB-1) mediated by RNA and regulates the splicing of genes such as CD44 molecule
MeCP2 and miRNA
Recent studies have demonstrated that MeCP2 can regulate the expression of miRNAs [Figure 1]. MeCP2 represses the transcription of primary miRNAs (pri-miRNAs), including miR-137[44], miR-15a[45], miR-184[46], and miR-7b[47], by binding to mCG sites in gene promoter regions. Consequently, the loss of MeCP2 enhances the transcription of pri-RNAs, resulting in the expression of mature miRNAs[48]. Furthermore, MeCP2 can alter the differentiation fate of cells by affecting the expression level of miRNAs. For example, MeCP2 can lead to the upregulation of miR-199, which could inhibit the ERK signaling pathway by targeting PAK4, thus hindering early neuron development[49]. In MeCP2 knockout male mice, Mellios et al. observed that the absence of MeCP2 resulted in the upregulation of Let-7f miRNA by suppressing BDNF expression. Subsequently, the elevated Let-7f miRNA levels exerted a direct inhibitory effect on IGF-1 synthesis, consequently leading to perturbed neuronal and astrocytic morphology as well as compromised functional integrity[50]. The deficiency of MeCP2 is associated with the downregulation of miR-146a and miR-146b, leading to the concomitant upregulation of their target gene, IL-1 receptor-associated kinase 1 (Irak1). As a consequence, this dysregulation instigates inflammatory responses, specifically within neurons[51]. Horvath et al. conducted a study on the hippocampus, revealing that MeCP2 exerts regulatory control over synaptic function through its interaction with miR-101a[52]. However, the specific molecular mechanisms underlying this phenomenon have yet to be elucidated. Altogether, the abovementioned studies suggest that RTT-associated mutations can alter multiple miRNA pathways downstream of MeCP2 and lead to impaired brain development and maturation. An in-depth understanding of MeCP2 function may help to elucidate the pathogenesis of RTT and develop effective therapeutic strategies.
CLINICAL CHARACTERISTICS OF RTT
The clinical presentation of RTT is closely related to the age of female patients and can be divided into four stages accordingly[53]. Patients develop normally during the first 6 - 18 months of life, and then experience four sequential stages of onset: stagnation (age 6-18 months), rapid regression (age 1-4 years), pseudo stationary (age 2-potentially life) and late motor deterioration (age 10-life)[7]. Despite their poor physical conditions, patients with RTT can typically survive until adulthood and even up to 70 years of age (70% of patients survive until 45 years of age)[22]. Cardiopulmonary problems are the main cause of death in patients with RTT[54]. Compared with female patients, male patients present with more severe phenotypes that are typically accompanied by severe congenital brain disease. MECP2 mutations have been detected in male fetal tissues and are considered lethal[55]. Owing to its typical sex preference and a sex ratio opposite to that of autism spectrum disorder (ASD) (male: female = 4:1), RTT is no longer classified as ASD in the fifth edition of the Diagnostic and Statistical Manual of Mental Disorders (DSM-V).
RTT AND WM ABNORMALITIES
The pathogenesis of RTT remains largely unclear due to the limited research available, which is primarily based on autopsy reports. However, the rapid development of imaging technology, particularly MRI, and its application in the medical field have improved the understanding of brain developmental abnormalities in RTT patients, providing valuable guidance for further research. MRI scans conducted on patients of varying ages and with different mutation types have revealed that abnormal brain regions in patients with RTT are primarily localized in the frontal lobe, caudate nucleus, putamen, thalamus, parietal lobe, and hippocampus[56]. In addition to the commonly observed GM abnormalities, a decrease in the overall volume of WM and thickness abnormalities in the genu and splenium of the corpus callosum (CC) suggest that functional defects in MeCP2 can lead to WM abnormalities[57]. MRI has been used to image mouse and monkey models to examine the potential association between abnormal brain structure development and behavioral phenotypes in RTT. Grand et al. used MRI and detected overall brain shrinkage and significantly decreased motor cortex and CC thickness in MECP2-knockout mouse models[58]. The presence of CC abnormalities indicates the occurrence of WM abnormalities in mouse models[59]. Similarly, we have observed abnormalities in WM development, particularly in brain regions such as the prefrontal lobe and motor cortex, through brain imaging of monkey models established using Clustered Regularly Interspaced Short Palindromic Repeats (CRISPR/Cas9) technology[55]. Both mouse and monkey models exhibit WM development abnormalities similar to those found in patients. As shown in Table 1, the results of MRI detection of brain dysplasia in patients with RTT (including atypical RTT) and RTT models (mouse and monkey) show that WM abnormalities (especially in the CC) are common in RTT. Owing to the absence of neuronal bodies in WM, it can be inferred that WM abnormalities are closely associated with glial cells. With the rapid development of imaging technology and improved understanding of glial cells, the role of WM in the brain is gradually receiving substantial attention from scholars.
Differences in brain imaging findings between patients with RTT and animal models of RTT
Gene | Species | Sex | Mutation | Cerebral Atrophy | The total volume of WM | Abnormal brain regions in GM | Abnormal brain regions in WM | Ref. |
MECP2 | Human | Female Male | -- | √ | √ | Frontal lobe, parietal Lobe, and cerebellum | Superior longitudinal fasciculus, CC, and middle cerebellar peduncle | Mahmood[57] |
MECP2 | Human | Female | -- | √ | -- | The parietal lobe, prefrontal lobe, and white matter fiber bundles | -- | Carter[65] |
MECP2 | Human | Female | -- | √ | -- | -- | All | Tani[66] |
MECP2 | Human | Female | R190H | √ | -- | -- | All (delayed myelination) | Zhou[67] |
MECP2 | Human | Female | -- | √ | -- | Frontal lobe | CC | Ryo[60] |
MECP2 | Human | Male | -- | √ | -- | -- | -- | Takaji[68] |
CDKL5 | Human | Female | -5bp | √ | -- | Frontal lobe | CC | Saitsu[69] |
FOXG1 | Human | Female | -- | × | × | Frontal lobe | Kumakura[70] | |
Mecp2 | Mouse | Male | 308 | √ | -- | X | CC | De[71] |
Mecp2 | Mouse | Male | KO | √ | √ | All | All | Akaba[59] |
MECP2 | Monkey | Female | KO | √ | √ | occipital gyri, inferior temporal gyrus, and annectant gyrus | cingulate WM CC | Chen[55] |
The clinical symptoms of RTT are similar to those of ASD, and the imaging results of ASD patients also found that the abnormalities of CC are also similar to those of RTT patients[60,61]. Given that the WM abnormalities of RTT and ASD are similar, this finding suggests that WM abnormalities have common features among neurodevelopmental disorders[16,61,62]. The main cell group in WM is OL lineage cells, and OLs are one of the most important components of nerve fiber bundles[63,64]. OPCs differentiate into mature OLs through a series of complex and precise processes[19]. Therefore, we speculate that inhibition of oligodendrocyte precursor cells (OPCs) differentiation is the primary cause of WM abnormalities in patients with RTT. The following sections describe the developmental process of OPCs, which may help to understand the cause of WM abnormalities.
Generation of OPCs
OPCs are widely distributed throughout the CNS and account for approximately 5% of the total cells in the CNS[72]. They can continuously proliferate and differentiate, with their primary function being the differentiation into mature OLs. Additionally, OPCs have the capacity to differentiate into astrocytes and neurons[73]. They play a crucial role in the CNS by participating in immune regulation, neurogenesis regulation, and the formation of synapses with neurons[73]. It is important to note that OPCs are heterogeneous, meaning that their functions vary across different brain regions. Despite originating from the neural tube epithelium, OPCs exhibit functional heterogeneity throughout the CNS, which can be attributed to variations in their time of generation[74,75]. Studies have demonstrated that the developmental process of OPCs and the timing of their development are different between humans and mice. In mice, OPCs are generated in different brain regions at three distinct time points during development. Initially, OPCs emerge in the embryonic cerebellum at E11.5d[74]. OPCs in the cerebral cortex are produced in the medial ganglionic eminence and the medial prefrontal region at E12.5d; these OPCs migrate dorsally and ventrally, eventually reaching the developing cortex at E16.5d[76]. The second phase of OPC proliferation mainly originates from the striatum at E15.5d; these cells migrate to the cortex and eventually become distributed throughout the brain[76]. The third stage of OPC production occurs in the subventricular zone (SVZ) at birth; these cells migrate to the CC and neighboring cortex[76]. Notably, the earliest OPCs that migrate to the cortex are gradually replaced by OPCs produced during the third stage of development, with complete replacement occurring approximately 10 days after birth[77]. At present, the understanding of OPCs in the human brain is not as clear as that in the mouse brain. Studies have suggested that OPCs in the human brain are generated during two concentrated periods. The first peak appears around week 10 of pregnancy. During this stage, OPCs can be observed in the frontal brain, lasting until approximately week 15[77,78]. The second peak occurs during the postnatal period and extends up to 5 years of age, followed by a decrease in OPC proliferation efficiency; however, OPC proliferation can be detected in the adult human brain[79].
OPC differentiation
In the CNS, only OPCs can differentiate into mature OLs through complex and strict regulatory mechanisms. According to different developmental stages, OL lineage cells can be classified as OPCs, immature OLs, and mature OLs. Studies have demonstrated that the maturation of OLs is regulated by factors such as extracellular signaling molecules, epigenetic regulation, and transcription factors. Subsequent chapters will delve into the elucidation of mechanisms influencing OPC differentiation, focusing on miRNA, mitochondrial abnormalities, and immune-related factors.
Transcription factors
Transcriptional regulators play an essential role in maintaining the proliferation and differentiation of OPCs. Common transcriptional regulators include OL transcription factor 2 (Olig2), SRY-box 10 (Sox10), NK2 homeobox 2 (Nkx2.2), and myelin regulatory factor (MYRF)[19]. During the differentiation of NSCs into OPCs, Olig2 is first expressed and can be used as a marker of OPCs[80]. Some researchers have referred to Olig2-positive cells as Pri-OPCs. Olig2 can promote the early differentiation of OPCs, and its expression gradually decreases as differentiation progresses[81]. Wu et al. reported that the combination of proline-rich coiled-coil 2A (Prrc2a) and N6-methyladenosine (m6A) maintained the transcription level of Olig2[82]. Moreover, insufficient myelination was observed in mouse models with a specific knockout of Prrc2a in OPCs, suggesting that Olig2 can promote the differentiation of OPCs[82]. Olig2 can induce the expression of Sox10, which acts as a DNA-binding protein to directly regulate the expression of myelin basic protein (MBP) and promote the differentiation of OPCs[83]. Sox10 can directly regulate the transcription factor MYRF[84]. Together, Sox10 and MYRF can collectively regulate the expression of MBP, myelin oligodendrocyte glycoprotein (MOG), and other genes and promote the differentiation of im-OLs to ma-OLs[84,85]. Nkx2.2 plays an important role in the occurrence and differentiation of OPCs. Related studies have demonstrated that Nkx2.2 can directly bind to the PDGF-α promoter to inhibit its expression, thereby promoting OPC differentiation[86]. However, the underlying mechanisms remain unclear. Studies have demonstrated that the transcription factors inhibitor of DNA binding 2/4 (Id2/4), transcription factor 4 (TCF4), and zinc finger protein 24 (ZFP24) play an indispensable role in OPC differentiation and OL maturation[19]. Altogether, recent novel findings may help to elucidate the development of OL lineage cells.
Epigenetic regulation
Studies on OL lineage cells have reported that epigenetic regulation, such as chromatin remodeling, histone modification, and RNA regulation, can promote OPC differentiation and myelin formation. Chromosomal condensation and decondensation during OPC differentiation are mainly mediated by the ATPase subunit Brahma-related 1 (BRG1, also known as Smarca4)[87]. Olig2 can recruit BRG1 to the enhancers and promoters of Sox10, MBP, and other OPC differentiation-related genes to promote their transcription[88]. Chromodomain helicase DNA binding protein 7 (CHD7), another chromatin remodeling-related factor, can promote transcription by forming a complex with BRG1 or with the promoters of genes involved in OPC differentiation, such as Sox10 or Nkx2.2[89]. Furthermore, the acetylation and methylation of histones can regulate the differentiation of OPCs. Loss of the core enzyme EZH2 in polycomb repressive complex 2 (PRC2), a histone methyltransferase, can prevent the differentiation of OPCs and reduce the number of OLs[90]. miRNAs can regulate OPC differentiation and myelin formation through post-transcriptional regulation. For example, miR-219 can promote OPC differentiation by specifically recognizing and inhibiting platelet-derived growth factor receptor, alpha polypeptide (PDGF-α), SRY-box transcription factor 6 (SOX6), hes family bHLH transcription factor 5 (HES5), forkhead box J3 (Foxj3), and other RNAs that inhibit genes related to OPC differentiation[91]. In addition, it can repress the expression of ELOVL fatty acid elongase 7 (Elovl7), a fatty acid elongation factor, to avoid excessive accumulation of fat in OLs, thereby maintaining the activity of OLs[92]. Other miRNAs involved in the regulation of the differentiation of OL lineage cells include miR-338, miR-138, miR-219, miR-200, and miR-9[93,94].
Signaling molecules
Extracellular hormones, growth factors, and other related factors promote the proliferation and differentiation of OPCs by activating specific signaling pathways. Some common regulatory factors include Triiodothyronine (T3), platelet-derived growth factor (PDGF), Insulin-like growth factor-1 (IGF-1), and fibroblast growth factor 2 (FGF2). T3 produced and released by the thyroid gland can enter the CNS through the blood–brain barrier to regulate the proliferation and differentiation of OL lineage cells[95]. T3 activates the PI3K/AKT signaling pathway by binding to the thyroid hormone receptor (TR), thereby terminating the cell cycle of OPCs and consequently promoting their differentiation into OLs[96]. In addition, T3 can promote the differentiation of NSCs into OPCs and the morphological and functional maturation of OLs[97]. IGF-1 is primarily synthesized by neurons and glial cells in the CNS. During the development of OPCs, IGF-1 mainly induces the PI3K/AKT and MAPK/ERK signaling cascades by binding to the IGF-1 receptor (IGF-1R), thus inhibiting OPC apoptosis and promoting proliferation[98]. Similar to IGF-1, PDGF is synthesized by neurons and glial cells. PDGF promotes the migration, proliferation, survival, and maturation of OPCs by binding to the PDGF-α receptor on OPCs[99]. In addition, PDGF can maintain the activity of OPCs by activating the JAK/STAT signaling pathway[100]. FGF2 can promote the differentiation of neural progenitor cells into OPCs, and can also induce the differentiation of OPCs into OLs[101]. Other signaling molecules that regulate the development of OPCs include ciliary neurotrophic factor (CNTF), leukemia inhibitory factor (LIF), and epidermal growth factor (EGF)[102-104]. However, the mechanism of action of these molecules warrants further investigation.
POTENTIAL MECHANISM OF ACTION OF MeCP2 IN OL LINEAGE CELLS
In previous studies, developmental abnormalities in WM have been observed on MRI and pathological examination in both RTT patients and animal models of RTT[16,58]. These abnormalities are characterized by a common trend of decreased WM volume and CC thickness. To verify whether the OL lineage cells lead to developmental abnormalities in WM, mouse models with specific knockdown of MeCP2 in OPCs have been used. According to the results of electron microscopy, these knockout mouse models have decreased CC thickness, decreased abundance of OPCs and OLs, and abnormal number and thicknesses of myelin sheaths in the CC[105]. These findings support the hypothesis that WM abnormalities result from the impaired development of OL lineage cells. However, the mechanisms through which MeCP2 regulates the development of OL lineage cells remain unclear. This section briefly summarizes the developmental process and regulatory mechanisms of OL lineage cells and describes the mechanisms through which MeCP2 regulates their proliferation, differentiation, and function. The findings reported in this section may improve the understanding of WM developmental abnormalities in RTT and even ASD.
MeCP2 regulates the differentiation of OPCs
Reduced CC thickness has been observed in both Mecp2-knockout mouse models and in the imaging and autopsy reports of human patients[56,105]. These findings suggest that MeCP2 regulates abnormal myelin formation. However, the underlying mechanisms remain elusive. Studies on primary culture of rat OPCs have demonstrated that Mecp2 expression increases with OPC differentiation and that MeCP2 can bind to the promoter regions of genes encoding myelin proteins such as MBP and PLP to regulate myelin formation in mature OLs[106]. Knockdown of MeCP2 can increase the expression of genes encoding MBP, PLP, myelin-associated oligodendrocyte basic protein (MOBP), MOG, brain-derived neurotrophic factor (BDNF), and transcription factor (YY1) and in the levels of MBP, PLP, and BDNF proteins[106]. Given that these genes are involved in OL differentiation and myelin formation, MeCP2 may play a negative regulatory role in myelin formation. A study using Mecp2-knockout mice revealed 130 differentially expressed genes (DEGs) in the brain. Among those DEGs, 34 genes were also abnormally expressed in the autism mouse model (Ptenm3m4 and Tcf4+/Mut). Enrichment analysis of these 34 genes was significantly enriched in several myelin formation-related gene ontology terms[107]. Approximately 15% of genes were associated with myelin regulation, and their expression was significantly increased, which was consistent with the results of in vitro experiments[107]. However, studies investigating the role of MeCP2 in regulating the proliferation and differentiation of OLs are limited. Therefore, understanding the precise mechanisms through which MeCP2 regulates OLs proliferation, differentiation, and myelin formation may facilitate the study of pathological mechanisms underlying WM abnormalities in RTT.
Function of MeCP2 in oligodendrocyte lineage cells
To date, studies on the pathogenesis of RTT have mainly focused on neurons and elucidated the specific mechanisms through which MeCP2 regulates neurogenesis and neuronal maturation. However, only a few studies have investigated the regulatory mechanisms of MeCP2 in OL progenitor cells. This section summarizes the potential functions of MeCP2 in OL progenitor cells based on the understanding of its functions in neurons and from the perspective of miRNAs, mitochondrial abnormalities, and immunity.
miRNA regulation
MicroRNAs (miRNAs) are small non-coding RNAs that consist of 20-24 nucleotides and typically inhibit gene expression by binding to the 3’-UTR of mRNAs[108]. miRNAs can be categorized into different types and have various biological functions. Recent studies have reported that most miRNAs are located within the introns of protein-coding genes[109]. Transcription of miRNAs results in the production of pri-miRNA transcripts with multiple hairpins in the nucleus. pri-miRNAs are cleaved by the Dorsha enzyme to produce hairpin precursor miRNAs (pre-miRNAs), which are cleaved by the ribonuclease Dicer1 in the cytoplasm to form mature miRNA[108]. miRNAs can regulate various biological functions such as cell proliferation, differentiation, apoptosis, and metabolism at the transcriptional level[110]. In addition, they are involved in regulating developmental processes such as neural development, neuronal maturation, and synaptic plasticity[111]. Abnormal miRNA expression has been observed in patients with RTT, Mecp2-null mouse brains, and in vitro cultured neurons[112]. As shown in Figure 2, MeCP2 can regulate miRNA expression either directly or indirectly. Although no relevant studies have demonstrated that MeCP2 affects OPCs development by regulating miRNA expression, considering that miRNAs have been reported to play a crucial role in the proliferation, differentiation, and myelination of OPCs[113,114], MeCP2 may regulate the proliferation and differentiation of OPCs through miRNAs.
Figure 2. Prediction of mechanisms through which MeCP2 regulates miRNAs in oligodendrocyte lineage cells. MeCP2 may regulate the expression of miRNA through two pathways in the nucleus. The first way is that MeCP2 can directly bind to the mCG site of the miRNA gene promoter to inhibit transcription. The second way is that MeCP2 can affect the number of Dorsha complexes by controlling the binding of the Dorsha and DGCR8. Dorsha complexes can cleave pri-miRNAs to generate pre-miRNAs. MeCP2 regulates the expression of pre-miRNAs in the nucleus and then inhibits the translation of downstream target mRNAs by affecting the expression levels of intracellular miRNAs. Black arrows indicate downregulation, and red arrows indicate upregulation.
Direct regulation of miRNA
The way MeCP2 directly regulates transcription is that it can specifically recognize and bind to the mCG site in the miRNA promoter region, inhibit the transcription of pri-miRNA and reduce the expression of miRNA[112] [Figure 2]. MeCP2 exerts direct inhibitory effects on miR-15a transcription, whereas miR-15a, in turn, modulates neuronal growth through the regulation of brain-derived neurotrophic factor (BDNF) expression[45]. The methylation of the 5’-flanking region of miR-7b plays a crucial role in recruiting MeCP2 and subsequently represses miR-7b expression. Simultaneously, miR-7b exhibits a specific binding affinity towards the MECP2-3’UTR region, thereby exerting inhibitory control over its expression. These reciprocal interactions establish a dynamic equilibrium between miR-7b and MeCP2[47]. Studies on gastric cancer cells have demonstrated that MeCP2 specifically recognizes CpG islands in the miR-338 promoter and inhibits the expression of miR-338-3p and miR-338-5p[115,116]. These two miRNAs can suppress the proliferation of gastric cancer cells by regulating the expression of phosphatidylinositol-3,4,5-trisphosphate dependent Rac exchange factor 2 (P-REX2) and BMI1 proto-oncogene, polycomb ring finger (BMI1), respectively[117]. Additionally, miR-338 inhibits the expression of proteins that promote OPC proliferation, thus promoting their differentiation into myelinating Ols. MiR-338-5p and miR-338-3p can promote the differentiation of OPCs by inhibiting the expression of differentiation inhibitors such as Sox6 and HES5[118]. This indicates that MeCP2 may have the ability to regulate the proliferation and differentiation of OPC through miR-338-5p and miR-338-3p.
Indirect regulation of miRNA
About indirect regulation in Figure 2, dephosphorylation of MeCP2 at pS80 leads to its dissociation from the chelated DiGeorge syndrome critical region 8 (DGCR8) and allows DGCR8 to recruit Dorsha complexes to cleave pri-miRNAs[48,108]. It is noteworthy that abnormal MeCP2 phosphorylation in different cell types may be the primary reason for abnormal miRNA expression. Mellios et al. found that MeCP2 delayed the early differentiation of neurons by upregulating miR-199 and miR-214 expression. MiR-214 can target and inhibit the expression of PTEN, thereby activating the AKT signaling pathway to inhibit neuronal differentiation[49,119]. Nakashine et al. demonstrated that MeCP2 upregulates the expression of miR-199a in NSCs by binding to the Dorsha receptor, while miR-199a promotes NSC differentiation into neurons by decreasing the expression of Smad1[120]. In addition, studies on OPCs derived from human ES cells have reported that miR-199a and miR-214 can inhibit OPC differentiation by suppressing the expression of MOBP and MYRF[121,122]. Therefore, MeCP2 may regulate OPC differentiation by regulating miR-199 and miR-214. In non-classic RTT, the FOXG1 protein regulates miRNA biogenesis by recruiting Dorsha, indirectly indicating the importance of miRNAs in the development of RTT[123].
Based on a literature search and analysis of miRNAs that are commonly regulated by MeCP2 in both neural cells and OPCs, it has been hypothesized that MeCP2 regulates OPC differentiation through miR-26[124,125], miR-30[126,127], miR-146[51,128], and miR-203[52,129], and regulates OLs maturation and myelination through miR-9[130,131], miR-21[132,133], miR-23[130], miR-184[46,134]. Figure 2 shows the potential signaling pathways that are affected by MeCP2 through the regulation of miR-199 and miR-214, and miR-338 in OPCs. This information may guide future studies investigating the regulatory effects of MeCP2 on miRNAs during the proliferation, differentiation, and myelination of OL lineage cells.
Mitochondrial abnormalities
RTT is considered a mitochondrial disease because of the shared clinical features between RTT syndrome and mitochondrial diseases, such as oxidative damage, abnormalities in electron transport chain (ETC) complexes, and elevated levels of lactate and pyruvate in the peripheral blood and cerebrospinal fluid[135-137]. Loss of MeCP2 can lead to abnormalities in the structure and function of mitochondria in RTT. Investigation of mitochondria in the muscle, skin, and different brain regions of patients with RTT has revealed pathological features such as abnormally enlarged mitochondria and dumbbell-shaped vacuoles[138-140]. A study demonstrated that loss of Mecp2 in 3-week-old mice resulted in an increase in the volume of mitochondria and the abundance of mitochondrial cristae without forming bubble-like structures[135]. Although no differences in mitochondrial morphology were observed in 12-week-old mice, significant abnormalities were observed in their mitochondrial function[135]. The primary function of mitochondria is to provide energy to cells through ATP synthesis. In addition, they play a role in regulating biological processes such as oxidative stress, lipid metabolism, and cell apoptosis.
To date, no studies have reported mitochondrial abnormalities in OLs in RTT. F2-isoprostanes are biomarkers for OS damage and have been detected in WM from patients with RTT[141], indicating that MeCP2 may induce OS and eventually lead to WM abnormalities by affecting mitochondrial function. OLs synthesize lipids required for myelin sheath formation and provide substances for neurons, such as lactate and pyruvate. Therefore, they require more mitochondria to maintain their energy expenditure[142,143]. To investigate the impact of MeCP2 on the structure and function of mitochondria, Aldosary et al. performed an integrated analysis of gene expression data from the brains and blood of patients with RTT and identified 77 common differentially expressed genes[137]. And most of them are involved in mitochondrial dysfunction and oxidative stress (OS) responses, which exacerbate the development of RTT[137].
OS abnormalities
OS is primarily caused by abnormal accumulation of ROS, which is mainly produced by mitochondria within cells. Therefore, MeCP2 may induce OS by regulating mitochondrial function. Elevated OS levels have been detected in Mecp2-null mice[144,145]. In a study, MeCP2 expression was restored in Mecp2-null mice to verify the causal relationship between Mecp2 and OS. The results revealed that reactivating MeCP2 in the MeCP2-deficient mouse brain restored the levels of OS markers such as F4 NeuroPs, F2 IsoPs, and NPBI[144]. Additionally, a decrease in superoxide dismutase (SOD) and glutathione (GSH) levels have been observed in symptomatic mouse models of RTT[146,147]. Downregulation of SOD and GSH may lead to excessive accumulation of ROS and induce OS responses. In addition, abnormal expression of genes associated with mitochondria or OS, such as uncoupling protein 3 (UCP3), cardiolipin synthase (CRLS1), NADH: ubiquinone oxidoreductase subunit A5 (NDUFA5), mitogen-activated protein kinase 9 (MAPK9), ATP synthase peripheral stalk subunit OSCP (ATP5PO), MAF bZIP transcription factor (MAF), Fos proto-oncogene (FOS), and stress-activated protein kinase (SAPK), has been observed in the brain tissues of mice with RTT[137]. In neurodegenerative diseases such as MS, an abnormal increase in mitochondrial activity in OLs can exacerbate oxidative damage, which may be the cause of demyelination[148]. This phenomenon indirectly verifies our hypothesis. In studies on OLs, abnormal accumulation of ROS has been demonstrated to cause damage to DNA and mtDNA, which not only leads to a decrease in the levels of mitochondrial respiratory chain-related proteins but also reduces the ability of OLs to form myelin[149]. In addition to providing cellular energy, mitochondria play a necessary role in lipid biosynthesis and myelin formation in OLs. Elevated ROS levels can lead to lipid peroxidation (LAHP) and inhibit myelin formation[150]. When ROS levels are extremely high, LAHP is oxidized to MDA, and the abnormal accumulation of MDA can lead to cell apoptosis.
MeCP2 and electron transport chain abnormalities
Because intracellular ROS are generated by the mitochondrial respiratory chain complex II, the detection of decreased ATP synthesis in Mecp2-null cells suggests that MeCP2 can affect OS levels by regulating mitochondria[151]. Studies on abnormal mitochondrial function in hippocampal neurons have demonstrated that MeCP2 deficiency significantly reduces the enzymatic activity of respiratory chain complex I, II, and IV and also results in an abnormal increase in ROS levels[152]. MeCP2 can directly upregulate the promoter of the uncoupling CQR-1 complex III protein gene (Uqccr1 complex III) with ubiquinone cytochrome c reductase core protein I[153]. However, Mecp2 knockout can increase the respiratory rate related to complex III in mitochondria in the brains of mice and accelerate the generation of ROS by increasing the electron chain non-coupling rate[138,153]. However, the energy demand of OPCs is higher than that of OLs, indicating that OPC differentiation is a high-energy-consuming process[154]. High-energy-demanding cells lead to an increase in ROS levels, which promotes OPC differentiation and myelin formation[148,155]. MeCP2 deficiency can induce mitochondrial abnormalities in neurons and astrocytes. If this phenomenon occurs in OLs, high energy demand and mitochondrial dysfunction can accelerate the abnormal accumulation of ROS, possibly leading to the release of cytochrome C (Cyt C) in the cytoplasm and inducing OPCs or OLs apoptosis. In addition, calcium ion disorders have been found in MeCP2-deficient astrocytes, and calcium ion accumulation owing to mitochondrial abnormalities can lead to Cyt C release in the cytoplasm and induce cell apoptosis[156].
As shown in Figure 3, we associate the mechanisms underlying MeCP2-induced mitochondrial abnormalities with research on mitochondria in neurodegenerative diseases and infer the role and function of MeCP2 in OLs.
Figure 3. Abnormal MeCP2 function may lead to changes in mitochondrial structure and function. MeCP2 may regulate the function of mitochondria by affecting electron transport chain complexes. The loss of MeCP2 function can lead to the release of cytochrome C into the cytoplasm and induce apoptosis. MeCP2 mutations can also lead to abnormal accumulation of reactive oxygen species, resulting in DNA damage and even apoptosis. However, an appropriate increase in ROS can make OPC exit the cell cycle and promote its differentiation. The abnormal function of MeCP2 can also reduce the coenzyme of dehydrogenase (NAD+) and the generation of ATP, thereby reducing the synthesis of lipids by affecting the reaction of tricarboxylic acid. OPC:oligodendrocyte precursor cell; ROS: reactive oxygen species.
Immunoregulation
Loss of MeCP2 function could cause immune dysfunction and chronic inflammation, exacerbating the deterioration of clinical characteristics[157]. Therefore, exploring the regulatory effects of MeCP2 on immune cell function and pro-inflammatory signaling could promote our understanding of the pathogenesis of RTT. As immune cells, microglia produce cytokines, such as interleukin (IL) -1β, tumor necrosis factor (TNF), and IL-6, in the CNS to regulate the developmental process[158,159]. Although specific knockout Mecp2 in microglia could not induce RTT pathological phenotypes in mice, specific restoration of Mecp2 in microglia can rescue the pathological phenotype in RTT mice and extend their lifespan, indicating that the microglia play an important role in the pathogenesis of RTT[160]. A recent study showed that the expression of TNF was upregulated in the microglia of an RTT mouse model, which can promote the occurrence of inflammatory reactions[161]. The upregulation of TNF can inhibit the mTOR signaling pathway by activating AMP-activated protein kinase (AMPK), preventing differentiation, and even inducing apoptosis of OPCs[162]. In addition, Feldhaus et al. found that TNF can also reduce the expression of MBP during OPC differentiation, which may be the main reason why MeCP2 inhibits OPC differentiation[163]. IL-9, IL-4, IL-1β, and IL-17 were upregulated in the plasma of RTT patients[164,165]. IL-9 can inhibit OPC differentiation, but the mechanism is unclear[166]. IL-4 can stimulate the microglia to secrete IGF-1, inhibiting the apoptosis of OPCs. IL-1β and IL-17 can directly inhibit the apoptosis of OPCs and promote their proliferation[167,168]. The mechanism of MeCP2 in regulating proliferation, differentiation, and apoptosis of OL lineage cells through affecting the expression of immune factors is not yet clear, and further studies are needed.
OPCs and immunoregulation
OLs are well-known as myelin-forming cells in the CNS. However, with the in-depth investigation of OL lineage cells, it is reported that OPCs also have immune functions, including secreting inflammatory factors, expressing cytokine receptors, and responding to inflammation[169]. Moyon et al. found that OPCs can release immune regulatory factors, such as IL-1β and CCL2, in a demyelination mouse model during the process of proliferation and migration to damaged areas[170]. Zeis et al. also confirmed that OPCs can express various immune regulatory factors, such as chemokines, cytokines, and complement[169]. Zveik et al. used cerebrospinal fluid from MS patients to treat OPCs in vitro and found that the treated OPCs showed inhibited proliferation and differentiation ability as well as reduced expression of histocompatibility complexes (MHC)-II and reduced secretion of TNF-α and NF-κB, which also provide evidence that OPCs has the immune function[171]. In addition, OPCs can also express immune-related proteins such as interleukin-17A (IL-17A), C-X-C motif chemokine ligand 10 (CXCL10), CD55, CD59, CD200, and CD47, further confirming that OL lineage cells can actively participate in the regulation of CNS inflammation[169,172]. The mechanism of OPCs in secreting immune factors remains unclear. Kishi et al. showed that MeCP2 can regulate cytokine expression through the NF-κB signaling pathway. Using RTT mouse cortex, they found that MeCP2 deficiency results in the loss of its transcriptional inhibitory effect on Irak1, which can activate the NF-κB signaling pathway to promote the entry of p65/RelA complex into the nucleus, thereby promoting the transcription of immune factors[173]. This may explain the immune dysfunction in RTT patients. In addition, Falcão et al. found that OPCs, like activated microglia, can phagocytose myelin granules[174]. Under inflammatory stimuli, immune-competent OPCs exit the cell cycle, exhibit the immune phenotype, and maintain immune function for a long time[73,175]. It remains unaddressed whether OPCs with an immunophenotype have the potential to differentiate.
Other regulatory effects
MeCP2 is involved in regulating the aging phenotype of OPCs and OLs. Studies have shown that MeCP2 is upregulated in primary cultured OPCs from the spinal cords of aged rats; however, the underlying mechanisms require further investigation[106]. Aging is often associated with OS, and MeCP2 may be involved in regulating the aging phenotype by regulating OS[176]. Because OPCs can form synapses with neurons, and this ability gradually disappears as they differentiate, MeCP2 can regulate the formation of OPC-neuron synapses like how it regulates synaptic plasticity in neurons[177-179]. Additionally, OPCs can secrete neuroregulatory factors to participate in cellular interactions, and MeCP2 may be involved in regulating the expression of neurotrophic factors[180,181]. Altogether, aging and OPC-neuron synapses may represent novel perspectives for investigating the regulatory mechanisms of MeCP2 in OL lineage cells.
CONCLUSION
Although classic RTT occurs owing to the functional loss of MeCP2, the complex types of mutations in the MECP2 gene and the diversity of MeCP2 protein functions have increased the difficulty of studying the pathogenesis of RTT. The mechanisms through which MeCP2 affects neuronal morphology and function are relatively well-understood, and the corresponding treatment methods or interventions have been shown to restore RTT-like phenotypes and prolong lifespan in mouse models. However, these approaches do not result in successful treatment. Glial cells play an essential role in the development of RTT. However, the underlying mechanisms warrant further investigation. In addition to aberrant neuronal morphology and function, abnormalities in total WM volume and CC thickness are observed in RTT. Abnormal OL function plays an integral role in causing WM abnormalities. Recent advances in epigenomics and transcriptomics, as well as their application in research on CNS, have improved the understanding of the regulation and function of OL lineage cells.
In conclusion, this review summarizes the function of MeCP2 in neurons. MeCP2 regulates various functions in OLs through transcription activation and inhibition, OS, miRNA expression, immunoregulation, and aging-related phenotypes. These findings may provide a rational basis for further investigating the role of MeCP2 in OL lineage cells and expediting research on WM abnormalities.
DECLARATIONS
Authors’ contributionsContributed the most to the conception and writing of the manuscript: Zhen Z
Construction of figures: Peng L
Conceived the project and revised the manuscript: Chen YC
Availability of data and materialsNot applicable.
Financial support and sponsorshipThis work was supported by the National Natural Science Foundation of China (grant numbers: 82125008 and 81930121), the National Science and Technology Innovation 2030 Major Program (grant number: 2021ZD0200900), the National Key Research and Development Program of China (grant numbers: 2018YFA0801403 and 2018YFA0107902), and the Natural Science Foundation of Yunnan Province (grant numbers: 202001BC070001 and 202102AA100053).
Conflicts of interestAll authors have no conflicts of interest to declare.
Ethical approval and consent to participateNot applicable.
Consent for publicationNot applicable.
Copyright© The Author(s) 2023.
REFERENCES
1. Williamson SL, Christodoulou J. Rett syndrome: new clinical and molecular insights. Eur J Hum Genet 2006;14:896-903.
2. Burd L, Randall T, Martsolf JT, Kerbeshian J. Rett syndrome symptomatology of institutionalized adults with mental retardation: comparison of males and females. Am J Ment Retard 1991;95:596-601.
3. Amir RE, Van den Veyver IB, Wan M, Tran CQ, Francke U, Zoghbi HY. Rett syndrome is caused by mutations in X-linked MECP2, encoding methyl-CpG-binding protein 2. Nat Genet 1999;23:185-8.
4. Evans JC, Archer HL, Colley JP, et al. Early onset seizures and Rett-like features associated with mutations in CDKL5. Eur J Hum Genet 2005;13:1113-20.
5. Philippe C, Amsallem D, Francannet C, et al. Phenotypic variability in Rett syndrome associated with FOXG1 mutations in females. J Med Genet 2010;47:59-65.
6. Pejhan S, Rastegar M. Role of DNA methyl-CpG-binding protein MeCP2 in Rett syndrome pathobiology and mechanism of disease. Biomolecules 2021;11:75.
7. Kyle SM, Vashi N, Justice MJ. Rett syndrome: a neurological disorder with metabolic components. Open Biol 2018:8.
9. Chen RZ, Akbarian S, Tudor M, Jaenisch R. Deficiency of methyl-CpG binding protein-2 in CNS neurons results in a Rett-like phenotype in mice. Nat Genet 2001;27:327-31.
10. Baker SA, Chen L, Wilkins AD, Yu P, Lichtarge O, Zoghbi HY. An AT-hook domain in MeCP2 determines the clinical course of Rett syndrome and related disorders. Cell 2013;152:984-96.
11. Guy J, Gan J, Selfridge J, Cobb S, Bird A. Reversal of neurological defects in a mouse model of Rett syndrome. Science 2007;315:1143-7.
12. Zhou H, Wu W, Zhang Y, et al. Selective preservation of cholinergic MeCP2 rescues specific Rett-syndrome-like phenotypes in MeCP2(stop) mice. Behav Brain Res 2017;322:51-9.
13. Maezawa I, Swanberg S, Harvey D, LaSalle JM, Jin LW. Rett syndrome astrocytes are abnormal and spread MeCP2 deficiency through gap junctions. J Neurosci 2009;29:5051-61.
14. Alvarez-Saavedra M, Sáez MA, Kang D, Zoghbi HY, Young JI. Cell-specific expression of wild-type MeCP2 in mouse models of Rett syndrome yields insight about pathogenesis. Hum Mol Genet 2007;16:2315-25.
15. Lioy DT, Garg SK, Monaghan CE, et al. A role for glia in the progression of Rett’s syndrome. Nature 2011;475:497-500.
16. Kong Y, Li QB, Yuan ZH, et al. Multimodal neuroimaging in Rett syndrome with MECP2 mutation. Front Neurol 2022;13:838206.
17. Shiohama T, Tsujimura K. Quantitative structural brain magnetic resonance imaging analyses: methodological overview and application to Rett syndrome. Front Neurosci 2022;16:835964.
18. Rivera AD, Chacon-De-La-Rocha I, Pieropan F, Papanikolau M, Azim K, Butt AM. Keeping the ageing brain wired: a role for purine signalling in regulating cellular metabolism in oligodendrocyte progenitors. Pflugers Arch 2021;473:775-83.
19. Elbaz B, Popko B. Molecular control of oligodendrocyte development. Trends Neurosci 2019;42:263-77.
20. Kriaucionis S, Bird A. The major form of MeCP2 has a novel N-terminus generated by alternative splicing. Nucleic Acids Res 2004;32:1818-23.
21. Olson CO, Zachariah RM, Ezeonwuka CD, Liyanage VR, Rastegar M. Brain region-specific expression of MeCP2 isoforms correlates with DNA methylation within Mecp2 regulatory elements. PLoS One 2014;9:e90645.
22. Sandweiss AJ, Brandt VL, Zoghbi HY. Advances in understanding of Rett syndrome and MECP2 duplication syndrome: prospects for future therapies. Lancet Neurol 2020;19:689-98.
23. Rodrigues DC, Mufteev M, Ellis J. Regulation, diversity and function of MECP2 exon and 3’UTR isoforms. Hum Mol Genet 2020;29:R89-99.
25. Neul JL, Fang P, Barrish J, et al. Specific mutations in methyl-CpG-binding protein 2 confer different severity in Rett syndrome. Neurology 2008;70:1313-21.
26. Goto T, Monk M. Regulation of X-chromosome inactivation in development in mice and humans. Microbiol Mol Biol Rev 1998;62:362-78.
27. Trappe R, Laccone F, Cobilanschi J, et al. MECP2 mutations in sporadic cases of Rett syndrome are almost exclusively of paternal origin. Am J Hum Genet 2001;68:1093-101.
28. Stenson PD, Ball EV, Mort M, et al. Human gene mutation database (HGMD): 2003 update. Hum Mutat 2003;21:577-81.
29. Lee SS, Wan M, Francke U. Spectrum of MECP2 mutations in Rett syndrome. Brain Dev 2001;23 Suppl 1:S138-43.
30. Krishnaraj R, Ho G, Christodoulou J. RettBASE: Rett syndrome database update. Hum Mutat 2017;38:922-31.
31. Brown K, Selfridge J, Lagger S, et al. The molecular basis of variable phenotypic severity among common missense mutations causing Rett syndrome. Hum Mol Genet 2016;25:558-70.
32. Nikitina T, Ghosh RP, Horowitz-Scherer RA, Hansen JC, Grigoryev SA, Woodcock CL. MeCP2-chromatin interactions include the formation of chromatosome-like structures and are altered in mutations causing Rett syndrome. J Biol Chem 2007;282:28237-45.
33. Lyst MJ, Ekiert R, Ebert DH, et al. Rett syndrome mutations abolish the interaction of MeCP2 with the NCoR/SMRT co-repressor. Nat Neurosci 2013;16:898-902.
34. Pidcock FS, Salorio C, Bibat G, et al. Functional outcomes in Rett syndrome. Brain Dev 2016;38:76-81.
35. Cuddapah VA, Pillai RB, Shekar KV, et al. Methyl-CpG-binding protein 2 (MECP2) mutation type is associated with disease severity in Rett syndrome. J Med Genet 2014;51:152-8.
36. Mellén M, Ayata P, Heintz N. 5-hydroxymethylcytosine accumulation in postmitotic neurons results in functional demethylation of expressed genes. Proc Natl Acad Sci U S A 2017;114:E7812-21.
37. Sperlazza MJ, Bilinovich SM, Sinanan LM, Javier FR, Williams DC Jr. Structural basis of MeCP2 distribution on Non-CpG methylated and hydroxymethylated DNA. J Mol Biol 2017;429:1581-94.
38. Liu K, Xu C, Lei M, et al. Structural basis for the ability of MBD domains to bind methyl-CG and TG sites in DNA. J Biol Chem 2018;293:7344-54.
39. Young JI, Hong EP, Castle JC, et al. Regulation of RNA splicing by the methylation-dependent transcriptional repressor methyl-CpG binding protein 2. Proc Natl Acad Sci U S A 2005;102:17551-8.
40. Brito DVC, Gulmez Karaca K, Kupke J, Frank L, Oliveira AMM. MeCP2 gates spatial learning-induced alternative splicing events in the mouse hippocampus. Mol Brain 2020;13:156.
41. Gonzales ML, Adams S, Dunaway KW, LaSalle JM. Phosphorylation of distinct sites in MeCP2 modifies cofactor associations and the dynamics of transcriptional regulation. Mol Cell Biol 2012;32:2894-903.
42. Kleene R, Loers G, Schachner M. The KDET Motif in the intracellular domain of the cell adhesion molecule L1 interacts with several nuclear, cytoplasmic, and mitochondrial proteins essential for neuronal functions. Int J Mol Sci 2023;24:932.
43. Wong JJ, Gao D, Nguyen TV, et al. Intron retention is regulated by altered MeCP2-mediated splicing factor recruitment. Nat Commun 2017;8:15134.
44. Chen T, Cai SL, Li J, et al. Mecp2-mediated epigenetic silencing of miR-137 contributes to colorectal adenoma-carcinoma sequence and tumor progression via relieving the suppression of c-Met. Sci Rep 2017;7:44543.
45. Gao Y, Su J, Guo W, et al. Inhibition of miR-15a promotes BDNF expression and rescues dendritic maturation deficits in MeCP2-deficient neurons. Stem Cells 2015;33:1618-29.
46. Nomura T, Kimura M, Horii T, et al. MeCP2-dependent repression of an imprinted miR-184 released by depolarization. Hum Mol Genet 2008;17:1192-9.
47. Chen Y, Shin BC, Thamotharan S, Devaskar SU. Differential methylation of the micro-RNA 7b gene targets postnatal maturation of murine neuronal Mecp2 gene expression. Dev Neurobiol 2014;74:407-25.
48. Cheng TL, Wang Z, Liao Q, et al. MeCP2 suppresses nuclear microRNA processing and dendritic growth by regulating the DGCR8/Drosha complex. Dev Cell 2014;28:547-60.
49. Mellios N, Feldman DA, Sheridan SD, et al. MeCP2-regulated miRNAs control early human neurogenesis through differential effects on ERK and AKT signaling. Mol Psychiatry 2018;23:1051-65.
50. Mellios N, Woodson J, Garcia RI, et al. β2-Adrenergic receptor agonist ameliorates phenotypes and corrects microRNA-mediated IGF1 deficits in a mouse model of Rett syndrome. Proc Natl Acad Sci U S A 2014;111:9947-52.
51. Urdinguio RG, Fernandez AF, Lopez-Nieva P, et al. Disrupted microRNA expression caused by Mecp2 loss in a mouse model of Rett syndrome. Epigenetics 2010;5:656-63.
52. Horvath PM, Piazza MK, Kavalali ET, Monteggia LM. MeCP2 loss-of-function dysregulates microRNAs regionally and disrupts excitatory/inhibitory synaptic transmission balance. Hippocampus 2022;32:610-23.
53. Vashi N, Justice MJ. Treating Rett syndrome: from mouse models to human therapies. Mamm Genome 2019;30:90-110.
54. Tarquinio DC, Hou W, Neul JL, et al. The changing face of survival in Rett syndrome and MECP2-related disorders. Pediatr Neurol 2015;53:402-11.
55. Chen Y, Yu J, Niu Y, et al. Modeling rett syndrome using TALEN-Edited MECP2 mutant cynomolgus monkeys. Cell 2017;169:945-55.e10.
56. Grattan-Smith JD, Chow J, Kurugol S, Jones RA. Quantitative renal magnetic resonance imaging: magnetic resonance urography. Pediatr Radiol 2022;52:228-48.
57. Mahmood A, Bibat G, Zhan AL, et al. White matter impairment in Rett syndrome: diffusion tensor imaging study with clinical correlations. AJNR Am J Neuroradiol 2010;31:295-9.
58. Allemang-Grand R, Ellegood J, Spencer Noakes L, et al. Neuroanatomy in mouse models of Rett syndrome is related to the severity of Mecp2 mutation and behavioral phenotypes. Mol Autism 2017;8:32.
59. Akaba Y, Shiohama T, Komaki Y, et al. Comprehensive volumetric analysis of Mecp2-null mouse model for Rett syndrome by T2-weighted 3D magnetic resonance imaging. Front Neurosci 2022;16:885335.
60. Takeguchi R, Kuroda M, Tanaka R, et al. Structural and functional changes in the brains of patients with Rett syndrome: a multimodal MRI study. J Neurol Sci 2022;441:120381.
61. Zhao Y, Yang L, Gong G, Cao Q, Liu J. Identify aberrant white matter microstructure in ASD, ADHD and other neurodevelopmental disorders: a meta-analysis of diffusion tensor imaging studies. Prog Neuropsychopharmacol Biol Psychiatry 2022;113:110477.
62. Wang J, Wang Z, Zhang H, et al. White matter structural and network topological changes underlying the behavioral phenotype of MECP2 mutant monkeys. Cereb Cortex 2021;31:5396-410.
63. Concha L. A macroscopic view of microstructure: using diffusion-weighted images to infer damage, repair, and plasticity of white matter. Neuroscience 2014;276:14-28.
64. Graciarena M, Seiffe A, Nait-Oumesmar B, Depino AM. Hypomyelination and oligodendroglial alterations in a mouse model of autism spectrum disorder. Front Cell Neurosci 2018;12:517.
65. Carter JC, Lanham DC, Pham D, Bibat G, Naidu S, Kaufmann WE. Selective cerebral volume reduction in Rett syndrome: a multiple-approach MR imaging study. AJNR Am J Neuroradiol 2008;29:436-41.
66. Tani H, Ishikawa N, Kobayashi Y, et al. Anti-MOG antibody encephalitis mimicking neurological deterioration in a case of Rett syndrome with MECP2 mutation. Brain Dev 2018;40:943-6.
67. Zhou X, Liao Y, Xu M, et al. A novel mutation R190H in the AT-hook 1 domain of MeCP2 identified in an atypical Rett syndrome. Oncotarget 2017;8:82156-64.
68. Tokaji N, Ito H, Kohmoto T, et al. A rare male patient with classic Rett syndrome caused by MeCP2_e1 mutation. Am J Med Genet A 2018;176:699-702.
69. Saitsu H, Osaka H, Nishiyama K, et al. A girl with early-onset epileptic encephalopathy associated with microdeletion involving CDKL5. Brain Dev 2012;34:364-7.
70. Kumakura A, Takahashi S, Okajima K, Hata D. A haploinsufficiency of FOXG1 identified in a boy with congenital variant of Rett syndrome. Brain Dev 2014;36:725-9.
71. De Filippis B, Fabbri A, Simone D, et al. Modulation of RhoGTPases improves the behavioral phenotype and reverses astrocytic deficits in a mouse model of Rett syndrome. Neuropsychopharmacology 2012;37:1152-63.
72. Juarez A, He D, Richard Lu Q. Oligodendrocyte progenitor programming and reprogramming: toward myelin regeneration. Brain Res 2016;1638:209-20.
73. Akay LA, Effenberger AH, Tsai LH. Cell of all trades: oligodendrocyte precursor cells in synaptic, vascular, and immune function. Genes Dev 2021;35:180-98.
74. Weng Q, Wang J, Wang J, et al. Single-cell transcriptomics uncovers glial progenitor diversity and cell fate determinants during development and gliomagenesis. Cell Stem Cell 2019;24:707-723.e8.
75. Huang W, Bhaduri A, Velmeshev D, et al. Origins and proliferative states of human oligodendrocyte precursor cells. Cell 2020;182:594-608.e11.
76. Kessaris N, Fogarty M, Iannarelli P, Grist M, Wegner M, Richardson WD. Competing waves of oligodendrocytes in the forebrain and postnatal elimination of an embryonic lineage. Nat Neurosci 2006;9:173-9.
77. Jakovcevski I, Filipovic R, Mo Z, Rakic S, Zecevic N. Oligodendrocyte development and the onset of myelination in the human fetal brain. Front Neuroanat 2009;3:5.
78. Cui QL, D'Abate L, Fang J, et al. Human fetal oligodendrocyte progenitor cells from different gestational stages exhibit substantially different potential to myelinate. Stem Cells Dev 2012;21:1831-7.
79. Kuhn S, Gritti L, Crooks D, Dombrowski Y. Oligodendrocytes in development, myelin generation and beyond. Cells 2019;8:1424.
80. Dawson MR, Polito A, Levine JM, Reynolds R. NG2-expressing glial progenitor cells: an abundant and widespread population of cycling cells in the adult rat CNS. Mol Cell Neurosci 2003;24:476-88.
81. Xu J, Zhao J, Wang R, et al. Shh and Olig2 sequentially regulate oligodendrocyte differentiation from hiPSCs for the treatment of ischemic stroke. Theranostics 2022;12:3131-49.
82. Wu R, Li A, Sun B, et al. A novel m6A reader Prrc2a controls oligodendroglial specification and myelination. Cell Res 2019;29:23-41.
83. Liu Z, Hu X, Cai J, et al. Induction of oligodendrocyte differentiation by Olig2 and Sox10: evidence for reciprocal interactions and dosage-dependent mechanisms. Dev Biol 2007;302:683-93.
84. Hornig J, Fröb F, Vogl MR, Hermans-Borgmeyer I, Tamm ER, Wegner M. The transcription factors Sox10 and Myrf define an essential regulatory network module in differentiating oligodendrocytes. PLoS Genet 2013;9:e1003907.
85. Emery B, Agalliu D, Cahoy JD, et al. Myelin gene regulatory factor is a critical transcriptional regulator required for CNS myelination. Cell 2009;138:172-85.
86. Zhu Q, Zhao X, Zheng K, et al. Genetic evidence that Nkx2.2 and Pdgfra are major determinants of the timing of oligodendrocyte differentiation in the developing CNS. Development 2014;141:548-55.
87. Sanchez JC, Zhang L, Evoli S, et al. The molecular basis of selective DNA binding by the BRG1 AT-hook and bromodomain. Biochim Biophys Acta Gene Regul Mech 2020;1863:194566.
88. Matsumoto S, Banine F, Feistel K, et al. Brg1 directly regulates Olig2 transcription and is required for oligodendrocyte progenitor cell specification. Dev Biol 2016;413:173-87.
89. He D, Marie C, Zhao C, et al. Chd7 cooperates with Sox10 and regulates the onset of CNS myelination and remyelination. Nat Neurosci 2016;19:678-89.
90. Wang W, Cho H, Kim D, et al. PRC2 acts as a critical timer that drives oligodendrocyte fate over astrocyte identity by repressing the notch pathway. Cell Rep 2020;32:108147.
91. Dugas JC, Cuellar TL, Scholze A, et al. Dicer1 and miR-219 Are required for normal oligodendrocyte differentiation and myelination. Neuron 2010;65:597-611.
92. Shin D, Shin JY, McManus MT, Ptácek LJ, Fu YH. Dicer ablation in oligodendrocytes provokes neuronal impairment in mice. Ann Neurol 2009;66:843-57.
93. Wang H, Moyano AL, Ma Z, et al. miR-219 Cooperates with miR-338 in myelination and promotes myelin repair in the CNS. Dev Cell 2017;40:566-582.e5.
94. Buller B, Chopp M, Ueno Y, et al. Regulation of serum response factor by miRNA-200 and miRNA-9 modulates oligodendrocyte progenitor cell differentiation. Glia 2012;60:1906-14.
95. Gonzalez Cardona J, Smith MD, Wang J, et al. Quetiapine has an additive effect to triiodothyronine in inducing differentiation of oligodendrocyte precursor cells through induction of cholesterol biosynthesis. PLoS One 2019;14:e0221747.
96. Huang JY, Wang YX, Gu WL, et al. Expression and function of myelin-associated proteins and their common receptor NgR on oligodendrocyte progenitor cells. Brain Res 2012;1437:1-15.
97. Sharma KD, Alghazali KM, Hamzah RN, et al. Gold nanorod substrate for rat fetal neural stem cell differentiation into oligodendrocytes. Nanomaterials (Basel) 2022;12:929.
98. Pang Y, Zheng B, Fan LW, Rhodes PG, Cai Z. IGF-1 protects oligodendrocyte progenitors against TNFalpha-induced damage by activation of PI3K/Akt and interruption of the mitochondrial apoptotic pathway. Glia 2007;55:1099-107.
99. McKinnon RD, Waldron S, Kiel ME. PDGF alpha-receptor signal strength controls an RTK rheostat that integrates phosphoinositol 3’-kinase and phospholipase Cgamma pathways during oligodendrocyte maturation. J Neurosci 2005;25:3499-508.
100. Dell’albani P, Kahn M, Cole R, Condorelli D, Giuffrida-stella A, de Vellis J. Oligodendroglial survival factors, PDGF-AA and CNTF, activate similar JAK/STAT signaling pathways. J Neurosci Res 1998;54:191-205.
101. Azim K, Raineteau O, Butt AM. Intraventricular injection of FGF-2 promotes generation of oligodendrocyte-lineage cells in the postnatal and adult forebrain. Glia 2012;60:1977-90.
102. Deverman BE, Patterson PH. Exogenous leukemia inhibitory factor stimulates oligodendrocyte progenitor cell proliferation and enhances hippocampal remyelination. J Neurosci 2012;32:2100-9.
103. Vinukonda G, Hu F, Mehdizadeh R, et al. Epidermal growth factor preserves myelin and promotes astrogliosis after intraventricular hemorrhage. Glia 2016;64:1987-2004.
104. Talbott JF, Cao Q, Bertram J, et al. CNTF promotes the survival and differentiation of adult spinal cord-derived oligodendrocyte precursor cells in vitro but fails to promote remyelination in vivo. Exp Neurol 2007;204:485-9.
105. Nguyen MV, Felice CA, Du F, et al. Oligodendrocyte lineage cells contribute unique features to Rett syndrome neuropathology. J Neurosci 2013;33:18764-74.
106. Zhou J, Wu YC, Xiao BJ, Guo XD, Zheng QX, Wu B. Age-related changes in the global DNA methylation profile of oligodendrocyte progenitor cells derived from rat spinal cords. Curr Med Sci 2019;39:67-74.
107. Phan BN, Bohlen JF, Davis BA, et al. A myelin-related transcriptomic profile is shared by Pitt-Hopkins syndrome models and human autism spectrum disorder. Nat Neurosci 2020;23:375-85.
109. Rajman M, Schratt G. MicroRNAs in neural development: from master regulators to fine-tuners. Development 2017;144:2310-22.
110. Good KV, Vincent JB, Ausió J. MeCP2: The genetic driver of rett syndrome epigenetics. Front Genet 2021;12:620859.
111. Sarkar D, Leung EY, Baguley BC, Finlay GJ, Askarian-Amiri ME. Epigenetic regulation in human melanoma: past and future. Epigenetics 2015;10:103-21.
112. Ip JPK, Mellios N, Sur M. Rett syndrome: insights into genetic, molecular and circuit mechanisms. Nat Rev Neurosci 2018;19:368-82.
113. Galloway DA, Moore CS. miRNAs as emerging regulators of oligodendrocyte development and differentiation. Front Cell Dev Biol 2016;4:59.
114. Tiane A, Schepers M, Rombaut B, et al. From OPC to oligodendrocyte: an epigenetic journey. Cells 2019;8:1236.
115. AmeliMojarad M, AmeliMojarad M, Pourmahdian A. Circular RNA circ_0051620 sponges miR-338-3p and regulates ADAM17 to promote the gastric cancer progression. Pathol Res Pract 2022;233:153887.
116. Wang G, Zhang Z, Xia C. Long non-coding RNA LINC00240 promotes gastric cancer progression via modulating miR-338-5p/METTL3 axis. Bioengineered 2021;12:9678-91.
117. Guo B, Liu L, Yao J, et al. miR-338-3p suppresses gastric cancer progression through a PTEN-AKT axis by targeting P-REX2a. Mol Cancer Res 2014;12:313-21.
118. de Faria O Jr, Cui QL, Bin JM, et al. Regulation of miRNA 219 and miRNA clusters 338 and 17-92 in oligodendrocytes. Front Genet 2012;3:46.
119. Tsujimura K, Irie K, Nakashima H, et al. miR-199a Links MeCP2 with mTOR signaling and its dysregulation leads to rett syndrome phenotypes. Cell Rep 2015;12:1887-901.
120. Nakashima H, Tsujimura K, Irie K, et al. MeCP2 controls neural stem cell fate specification through miR-199a-mediated inhibition of BMP-Smad signaling. Cell Rep 2021;35:109124.
121. Bronstein JM, Tiwari-woodruff S, Buznikov AG, Stevens DB. Involvement of OSP/claudin-11 in oligodendrocyte membrane interactions: Role in biology and disease. J Neurosci Res 2000;59:706-11.
122. Letzen BS, Liu C, Thakor NV, Gearhart JD, All AH, Kerr CL. MicroRNA expression profiling of oligodendrocyte differentiation from human embryonic stem cells. PLoS One 2010;5:e10480.
123. Weise SC, Arumugam G, Villarreal A, et al. FOXG1 Regulates PRKAR2B transcriptionally and posttranscriptionally via miR200 in the adult hippocampus. Mol Neurobiol 2019;56:5188-201.
124. Shyamasundar S, Ramya S, Kandilya D, et al. Maternal diabetes deregulates the expression of Mecp2 via miR-26b-5p in mouse embryonic neural stem cells. Cells 2023;12:1516.
125. Lau P, Verrier JD, Nielsen JA, Johnson KR, Notterpek L, Hudson LD. Identification of dynamically regulated microRNA and mRNA networks in developing oligodendrocytes. J Neurosci 2008;28:11720-30.
126. Fang X, Sun D, Wang Z, et al. MiR-30a positively regulates the inflammatory response of microglia in experimental autoimmune encephalomyelitis. Neurosci Bull 2017;33:603-15.
127. Volkmann I, Kumarswamy R, Pfaff N, et al. MicroRNA-mediated epigenetic silencing of sirtuin1 contributes to impaired angiogenic responses. Circ Res 2013;113:997-1003.
128. Liu XS, Chopp M, Pan WL, et al. MicroRNA-146a promotes oligodendrogenesis in stroke. Mol Neurobiol 2017;54:227-37.
129. Ma Q, Matsunaga A, Ho B, Oksenberg JR, Didonna A. Oligodendrocyte-specific argonaute profiling identifies microRNAs associated with experimental autoimmune encephalomyelitis. J Neuroinflammation 2020;17:297.
130. Li JS, Yao ZX. MicroRNAs: novel regulators of oligodendrocyte differentiation and potential therapeutic targets in demyelination-related diseases. Mol Neurobiol 2012;45:200-12.
131. Hinojosa-Godinez A, Jave-Suarez LF, Flores-Soto M, et al. Melatonin modifies SOX2+ cell proliferation in dentate gyrus and modulates SIRT1 and MECP2 in long-term sleep deprivation. Neural Regen Res 2019;14:1787-95.
132. Miguel-Hidalgo JJ, Hall KO, Bonner H, et al. MicroRNA-21: expression in oligodendrocytes and correlation with low myelin mRNAs in depression and alcoholism. Prog Neuropsychopharmacol Biol Psychiatry 2017;79:503-14.
133. Koelsch KA, Webb R, Jeffries M, et al. Functional characterization of the MECP2/IRAK1 lupus risk haplotype in human T cells and a human MECP2 transgenic mouse. J Autoimmun 2013;41:168-74.
134. Afrang N, Tavakoli R, Tasharrofi N, et al. A critical role for miR-184 in the fate determination of oligodendrocytes. Stem Cell Res Ther 2019;10:112.
135. Belichenko PV, Wright EE, Belichenko NP, et al. Widespread changes in dendritic and axonal morphology in Mecp2-mutant mouse models of Rett syndrome: evidence for disruption of neuronal networks. J Comp Neurol 2009;514:240-58.
136. Zlatic SA, Duong D, Gadalla KKE, et al. Convergent cerebrospinal fluid proteomes and metabolic ontologies in humans and animal models of Rett syndrome. iScience 2022;25:104966.
137. Aldosary M, Al-Bakheet A, Al-Dhalaan H, et al. Rett syndrome, a neurodevelopmental disorder, whole-transcriptome, and mitochondrial genome multiomics analyses identify novel variations and disease pathways. OMICS 2020;24:160-71.
138. Dave A, Pillai PP. Docosahexaenoic acid increased MeCP2 mediated mitochondrial respiratory complexes II and III enzyme activities in cortical astrocytes. J Biochem Mol Toxicol 2022;36:e23002.
139. Zlatic SA, Werner E, Surapaneni V, et al. Systemic metabolic and mitochondrial defects in rett syndrome models. bioRxiv ;2023:2023.
140. Gold WA, Williamson SL, Kaur S, et al. Mitochondrial dysfunction in the skeletal muscle of a mouse model of Rett syndrome (RTT): implications for the disease phenotype. Mitochondrion 2014;15:10-7.
141. Durand T, De Felice C, Signorini C, et al. F(2)-Dihomo-isoprostanes and brain white matter damage in stage 1 Rett syndrome. Biochimie 2013;95:86-90.
142. Poitelon Y, Kopec AM, Belin S. Myelin Fat Facts: An overview of lipids and fatty acid metabolism. Cells 2020;9:812.
143. Marangon D, Boccazzi M, Lecca D, Fumagalli M. Regulation of oligodendrocyte functions: targeting lipid metabolism and extracellular matrix for myelin repair. J Clin Med 2020;9:470.
144. De Felice C, Della Ragione F, Signorini C, et al. Oxidative brain damage in Mecp2-mutant murine models of Rett syndrome. Neurobiol Dis 2014;68:66-77.
145. Filosa S, Pecorelli A, D'Esposito M, Valacchi G, Hajek J. Exploring the possible link between MeCP2 and oxidative stress in Rett syndrome. Free Radic Biol Med 2015;88:81-90.
146. Nance E, Kambhampati SP, Smith ES, et al. Dendrimer-mediated delivery of N-acetyl cysteine to microglia in a mouse model of Rett syndrome. J Neuroinflammation 2017;14:252.
147. Li N, Zhang T, He M, Mu Y. MeCP2 attenuates cardiomyocyte hypoxia/reperfusion-induced injury via regulation of the SFRP4/Wnt/β-catenin axis. Biomarkers 2021;26:363-70.
148. Benjamins JA, Nedelkoska L, Lisak RP. Melanocortin receptor subtypes are expressed on cells in the oligodendroglial lineage and signal ACTH protection. J Neurosci Res 2018;96:427-35.
149. Nissanka N, Moraes CT. Mitochondrial DNA damage and reactive oxygen species in neurodegenerative disease. FEBS Lett 2018;592:728-42.
150. Adibhatla RM, Hatcher JF. Lipid oxidation and peroxidation in CNS health and disease: from molecular mechanisms to therapeutic opportunities. Antioxid Redox Signal 2010;12:125-69.
151. Shulyakova N, Andreazza AC, Mills LR, Eubanks JH. Mitochondrial dysfunction in the pathogenesis of Rett syndrome: implications for mitochondria-targeted therapies. Front Cell Neurosci 2017;11:58.
152. Can K, Menzfeld C, Rinne L, et al. Neuronal redox-imbalance in Rett syndrome affects mitochondria as well as cytosol, and is accompanied by intensified mitochondrial O (2) consumption and ROS release. Front Physiol 2019;10:479.
153. Kriaucionis S, Paterson A, Curtis J, Guy J, Macleod N, Bird A. Gene expression analysis exposes mitochondrial abnormalities in a mouse model of Rett syndrome. Mol Cell Biol 2006;26:5033-42.
154. Rone MB, Cui QL, Fang J, et al. Oligodendrogliopathy in multiple sclerosis: low glycolytic metabolic rate promotes oligodendrocyte survival. J Neurosci 2016;36:4698-707.
155. Lichvarova L, Blum W, Schwaller B, Szabolcsi V. Parvalbumin expression in oligodendrocyte-like CG4 cells causes a reduction in mitochondrial volume, attenuation in reactive oxygen species production and a decrease in cell processes’ length and branching. Sci Rep 2019;9:10603.
156. Dong Q, Liu Q, Li R, et al. Mechanism and consequence of abnormal calcium homeostasis in Rett syndrome astrocytes. Elife 2018:7.
157. Pecorelli A, Cervellati C, Cordone V, Hayek J, Valacchi G. Compromised immune/inflammatory responses in Rett syndrome. Free Radic Biol Med 2020;152:100-6.
158. Mehler MF, Kessler JA. Hematolymphopoietic and inflammatory cytokines in neural development. Trends Neurosci 1997;20:357-65.
159. Derecki NC, Cronk JC, Kipnis J. The role of microglia in brain maintenance: implications for Rett syndrome. Trends Immunol 2013;34:144-50.
160. Derecki NC, Cronk JC, Lu Z, et al. Wild-type microglia arrest pathology in a mouse model of Rett syndrome. Nature 2012;484:105-9.
161. Cronk JC, Derecki NC, Ji E, et al. Methyl-CpG binding protein 2 regulates microglia and macrophage gene expression in response to inflammatory stimuli. Immunity 2015;42:679-91.
162. Bonora M, De Marchi E, Patergnani S, et al. Tumor necrosis factor-α impairs oligodendroglial differentiation through a mitochondria-dependent process. Cell Death Differ 2014;21:1198-208.
163. Feldhaus B, Dietzel ID, Heumann R, Berger B. [Corticoids protect oligodentrocyte precursor cells against cytokine-induced damage]. Zentralbl Gynakol 2004;126:282-5.
164. Pecorelli A, Cervellati F, Belmonte G, et al. Cytokines profile and peripheral blood mononuclear cells morphology in Rett and autistic patients. Cytokine 2016;77:180-8.
165. Leoncini S, De Felice C, Signorini C, et al. Cytokine dysregulation in MECP2- and CDKL5-related Rett syndrome: relationships with aberrant redox homeostasis, inflammation, and ω-3 PUFAs. Oxid Med Cell Longev 2015;2015:421624.
166. Ding X, Cao F, Cui L, Ciric B, Zhang GX, Rostami A. IL-9 signaling affects central nervous system resident cells during inflammatory stimuli. Exp Mol Pathol 2015;99:570-4.
167. Liu H, Yang X, Yang J, et al. IL-17 Inhibits oligodendrocyte progenitor cell proliferation and differentiation by increasing K+ channel Kv1.3. Front Cell Neurosci 2021;15:679413.
168. Li Y, Liu L, Ding X, Liu Y, Yang Q, Ren B. Interleukin-1β attenuates the proliferation and differentiation of oligodendrocyte precursor cells through regulation of the microRNA-202-3p/β-catenin/Gli1 axis. Int J Mol Med 2020;46:1217-24.
169. Zeis T, Enz L, Schaeren-Wiemers N. The immunomodulatory oligodendrocyte. Brain Res 2016;1641:139-48.
170. Moyon S, Dubessy AL, Aigrot MS, et al. Demyelination causes adult CNS progenitors to revert to an immature state and express immune cues that support their migration. J Neurosci 2015;35:4-20.
171. Zveik O, Fainstein N, Rechtman A, et al. Cerebrospinal fluid of progressive multiple sclerosis patients reduces differentiation and immune functions of oligodendrocyte progenitor cells. Glia 2022;70:1191-209.
172. Zhou B, Zhu Z, Ransom BR, Tong X. Oligodendrocyte lineage cells and depression. Mol Psychiatry 2021;26:103-17.
173. Kishi N, MacDonald JL, Ye J, Molyneaux BJ, Azim E, Macklis JD. Reduction of aberrant NF-κB signalling ameliorates Rett syndrome phenotypes in Mecp2-null mice. Nat Commun 2016;7:10520.
174. Falcão AM, van Bruggen D, Marques S, et al. Disease-specific oligodendrocyte lineage cells arise in multiple sclerosis. Nat Med 2018;24:1837-44.
175. Antel JP, Lin YH, Cui QL, et al. Immunology of oligodendrocyte precursor cells in vivo and in vitro. J Neuroimmunol 2019;331:28-35.
176. Kilanczyk E, Saraswat Ohri S, Whittemore SR, Hetman M. Antioxidant protection of NADPH-Depleted oligodendrocyte precursor cells is dependent on supply of reduced glutathione. ASN Neuro 2016;8:175909141666040.
177. Orduz D, Maldonado PP, Balia M, et al. Interneurons and oligodendrocyte progenitors form a structured synaptic network in the developing neocortex. Elife 2015:4.
178. Orduz D, Benamer N, Ortolani D, et al. Developmental cell death regulates lineage-related interneuron-oligodendroglia functional clusters and oligodendrocyte homeostasis. Nat Commun 2019;10:4249.
179. Mount CW, Yalçın B, Cunliffe-Koehler K, Sundaresh S, Monje M. Monosynaptic tracing maps brain-wide afferent oligodendrocyte precursor cell connectivity. Elife 2019:8.
180. Birey F, Kloc M, Chavali M, et al. Genetic and Stress-induced loss of NG2 glia triggers emergence of depressive-like behaviors through reduced secretion of FGF2. Neuron 2015;88:941-56.
Cite This Article

How to Cite
Download Citation
Export Citation File:
Type of Import
Tips on Downloading Citation
Citation Manager File Format
Type of Import
Direct Import: When the Direct Import option is selected (the default state), a dialogue box will give you the option to Save or Open the downloaded citation data. Choosing Open will either launch your citation manager or give you a choice of applications with which to use the metadata. The Save option saves the file locally for later use.
Indirect Import: When the Indirect Import option is selected, the metadata is displayed and may be copied and pasted as needed.
About This Article
Copyright
Data & Comments
Data
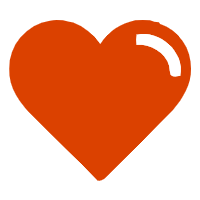
Comments
Comments must be written in English. Spam, offensive content, impersonation, and private information will not be permitted. If any comment is reported and identified as inappropriate content by OAE staff, the comment will be removed without notice. If you have any queries or need any help, please contact us at [email protected].