The versatile applications of human pluripotent stem cell-derived microglia and microglia-containing brain organoids
Abstract
Microglia are the resident immune cells of the central nervous system (CNS) and play pivotal roles in nervous development, homeostasis, and various neurological diseases. Most of the previous understanding of microglia came from rodents or a limited number of postmortem microglia. However, as significant differences between murine and human microglia have been verified, it has become increasingly apparent that rodents cannot accurately recapitulate human genetics and pathology, thus hindering the translation of microglial findings from rodents to humans. In addition, primary human microglia are notoriously difficult to obtain and lack the scalability required for many high-throughput assays. Fortunately, recent advances in microglia generation from human pluripotent stem cells (hPSCs) have enabled exciting new avenues to decipher or revisit microglial biology in the human context. Given the complex interactions between microglia and other CNS cells, hPSC-derived microglia-like cells (MGLs) were further engrafted within hPSC-derived brain organoids (BOs), which largely lack microglia due to their different embryonic origins, to study human microglial functions in either health and disease state closer to brain microglia. This is a rapidly evolving field, especially in the last five years, that has begun to yield novel insights into the genetics of human microglia and their unique role in neurological diseases. In this review, we will summarize the versatile applications of hPSC-derived MGLs and microglia-containing BOs. Specifically, we will discuss their applications in disease modeling, omics and systematic analysis, interaction with other CNS cell types, as well as transplantation-based human-mouse chimerism.
Keywords
INTRODUCTION
Microglial ontogeny and basic functions
Microglia, which reside in the brain, are the major innate immune cells of the central nervous system (CNS), first described and subsequently delineated by Pio del Rio-Hortega et al. more than a century ago. In the last three decades, the understanding of microglia has rapidly expanded due to the development of a series of murine models and the application of new techniques such as genetic manipulation, chemical ablation, fluorescent labeling, lineage tracing and multi-photon microscopy. Specifically, the ontogeny of microglia was elegantly revealed in 2010 by tracing the development of microglia[1]. Microglia originate from yolk sac progenitors generated during the first wave of primitive hematopoiesis and migrate through the bloodstream to the neural tube early in embryonic development[1,2]. Once the blood-brain barrier is formed, the flow of yolk sac progenitor cells into the CNS stops[3] and the early microglia population is homeostatic and maintained throughout adulthood by local self-renewal[4].
After colonization and maturation in the developing CNS[1,5], microglia play key roles in development, homeostasis, and multiple neurological diseases. Microglia in the developing neocortex actively interact with neural precursor cells and regulate their number[4,6,7]. Microglia shape synaptic connections and neuronal circuits through synapse pruning that engulfs and removes axons and dendritic spines and provide nutritional support[5,8,9]. Aberrant pruning has been associated with neurodevelopmental disorders[10], including schizophrenia[11] and autism spectrum disorder (ASD)[12]. Microglia also keep the CNS under constant surveillance by responding to a spectrum of stimuli, including various pathogen-associated molecular patterns (PAMPs), damage-associated molecular patterns (DAMPs), and also neural tissue damages in the form of neurodegeneration-associated molecular patterns (NAMPs)[13,14].
It is now well established that microglia and associated neuroinflammation are the shared hallmark of multiple neurodegenerative diseases, such as Alzheimer’s disease (AD), Parkinson’s disease (PD) and Amyotrophic lateral sclerosis (ALS)[15-18], while the pivotal role of microglia in sculpturing synapses is tightly associated with several neurodevelopmental disorders including ASD and schizophrenia. The field has recently been driven by single-cell RNA sequencing (scRNA-seq) and multi-omics studies that have revealed numerous genes and variants/mutations identified as risk factors for neurodegenerative diseases, with a considerable number of which are preferentially expressed by microglia. Likewise, the phenotype of microglia activation is recognized to be highly heterogeneous, especially in the last five years, accompanied by the development of scRNA-seq[19].
Murine vs. human microglia
So far, the majority of microglial functions and their heterogeneity have been understood through elegant studies on animal models and mouse microglia[20]. Nevertheless, interspecies differences often hinder the translation of microglia-related findings from rodent models to humans, especially in age-related neurodegenerative diseases. Rodent and human microglia differ markedly in proliferation rates, adhesion properties, and preservation of the key gene[21,22]. It is becoming increasingly apparent that rodents do not accurately recapitulate human genetics[23-25]. For example, more than half of the AD risk genes, such as CD33 or CR1, in microglia are below 70% identity between human and mouse[26,27].
Differences in expression profiles between species have also been documented. For instance, several studies of microglial transcriptomes have reported vast expression differences in brain development-related genes, inflammatory factors, complement genes, and risk genes associated with neurodegenerative diseases[22,28,29]. Overall, these species-specific differences may result in mouse models that do not faithfully mimic human diseases, thus hindering the clinical translation of previous findings[30]. These differences, therefore, highlight the need for faithful human models to delineate the cellular and molecular machinery associated with homeostatic, neuroprotective, and neurotoxic microglia.
Human samples
Primary human microglia from postmortem human brain tissue or diseased neurosurgical specimens have long been notoriously difficult to obtain, the limited number of which cannot be applied for many high-throughput applications. However, given that microglia are quite sensitive to environmental changes, the properties of available human microglia may vary considerably due to the different disease states of patients and the multi-step purification procedure, which may dampen the accuracy of in vivo models[28,31,32]. Furthermore, isolated microglia do not warrant studies to determine whether they exhibit phenotypically normal characteristics under disease-affected conditions, or whether they gain aberrant phenotypes due to their environmental origin.
Novel tool: human pluripotent stem cells and organoids
To study homeostatic human microglia, researchers have turned to human pluripotent stem cells (hPSCs), including human embryonic stem cells (hESCs) and human induced pluripotent stem cells (hiPSCs), which can differentiate into all three germ layer cell types [Figure 1]. Note that patient-specific iPSCs are typically reprogrammed from their somatic cells, such as PBMCs and fibroblasts, by introducing reprogramming factors such as the combination of OCT3/4, SOX2, KLF4, and c-MYC[33,34] [Figure 1]. Over the past decade, the advent of iPSC technologies has enabled the massive generation of various human CNS cell types that bear the genetic makeups of their patient donors[35]. Mounting studies based on reprogrammed iPSCs have focused on modeling the pathogenesis of inherited neurodegenerative diseases and screening for potential drugs, which have added new insights into previous findings[36,37].
Figure 1. Microglia and human pluripotent stem cells. Microglia are increasingly emerging as potential drivers of CNS diseases. Using high-throughput sequencing techniques such as GWAS and multi-omics analysis, a series of risk genes/loci associated with neurodegenerative diseases have been detected selectively or preferentially in microglia rather than other cell types in large populations of diseased and healthy patients. It is thus necessary to understand the molecular mechanisms of disease-associated genetic variants and their potential effects in mediating microglial functions. To circumvent the differences between mouse and human microglia, novel methods have been recently developed to differentiate hPSCs, including hESCs and hiPSCs reprogrammed from patients’ somatic cells, as well as isogenic hPSCs edited with CRISPR/Cas9, into MGLs that can provide an unlimited source of human microglia, especially bearing those variants, for disease modeling and studying the mechanisms of microglial activation. CNS: Central nervous system; CRISPR: clustered regularly interspaced short palindromic repeats; ESCs: embryonic stem cells; GWAS: genome-wide association studies; hESCs: human embryonic stem cells; hPSCs: human pluripotent stem cells; iPSCs: induced pluripotent stem cells; MGLs: microglia-like cells; PBMCs: peripheral blood mononuclear cells; WES: whole exome sequencing; WGS: whole genome sequencing; WT: wild type.
Since late 2016, with a clear understanding of microglial ontogeny and advances in stem cell biology, researchers have attempted to derive microglia from hPSCs, providing exciting new approaches to study and decipher human microglia biology and cell type-specific biology[38-43] [Figure 1]. Human PSCs could, in principle, provide an unlimited source of human microglia that can be used for multiple biochemical assays, omics analyses (transcriptomics, proteomics and metabolomics, etc.), secretome analysis, and co-culture with other hPSC-derived cell types (e.g., astrocytes and neurons) in vitro. Notably, given the complex interactions between neurons and glial cells, hPSC-derived microglia can also be incorporated into hPSC-derived organoids, which largely lack microglia due to their different embryonic origins, making them a better in vitro model[44-47]. Furthermore, microglial progenitor cells xenografted in the mouse brain bring a new in vivo model fitted in the brain cell environment, as they manifest phenotypes most resembling primary human microglia[27,48-52].
In this review, we aim to review the versatile applications of hPSC-derived microglia and microglia-containing organoids. Briefly, we will discuss their applications in disease modeling, omics and systematic analysis, interaction with other CNS cell types, as well as transplantation-based human-mouse chimerism.
HPSC-DERIVED MICROGLIA
While differentiation protocols of all major neural cell types from hPSCs were rapidly developed and widely studied, microglia production has long been elusive. Between 2016 and 2017, six seminal studies described dedicated differentiation protocols for generating human microglia-like cells (MGLs)[38-43]. Most protocols aim to mimic the in vivo trajectory of embryonic microglia differentiation, by exposing hPSCs to a series of growth factors or small chemicals in a timed, staged fashion. These protocols are also highly inspired by ontogeny studies that trace the microglia lineage from mesodermal primitive yolk sac progenitors to MYB-independent erythroid progenitors (EMPs), yolk sac macrophages, and eventually microglia[1,53,54]. According to this developmental trajectory, these protocols are able to generate cells that transition from hPSCs to microglia through a range of lineage states.
Note that these protocols use different culture conditions, media, and factors driving differentiation to generate MGLs in vitro[55]. Therefore, these protocols differ significantly in the required length of the differentiation period, the sorting needs to enrich intermediate cells, and the characteristics of the obtained MGLs. These protocols were neatly compared and discussed, which has also been nicely reviewed elsewhere[26,56]. Later on, the protocol was recently simplified by introducing a combination of pro-microglial factors, SPI1 and CEBPA (or CEBPB), into hPSCs, which skipped the progenitor cell stage of primitive development and thus shortened the time required for differentiation[57,58]. Each of these studies performed functional tests on their derived MGLs to validate that they could recapitulate a spectrum of traditional microglial cell behaviors.
For example, the ability of microglia to characterize inflammatory cues was tested by stimulating lipopolysaccharide (LPS), interferon-γ (IFN-γ) or interleukin-1β (IL-1β) and confirmed by measuring reactive oxygen species (ROS) or various inflammatory cytokines[39,40,42]. The propensity of MGLs to migrate towards the injury sites was also examined by the addition of ADP, which is usually released from injured neurons, or by employing a 3D culture system with laser-induced injury[57,59]. Basically, MGLs exhibited a rapid response, extending processes towards the perceived site of injury[38,40,41]. Furthermore, the phagocytic capacity was examined by utilizing various substrates, including pHrodo E. coli particles, microbeads coated with Zymosan, or more brain-associated substrates such as synaptosomes and fluro-conjugated fibrillar
CO-CULTURE WITH OTHER CELL TYPES
Mounting studies have shown interactions and regulation between microglia and astrocytes[61,62], while the neuronal regulation of microglial activation has also been well-studied[63,64]. Moreover, the interactions between vascular endothelial cells and microglia have also been implicated in development and diseases during recent years[65-67]. Given the complexity of various cell types inside the brain milieu, it might not be wise to translate the microglial functions from monocultured MGLs directly to the brain. Co-culturing human MGLs with hPSC-derived cells such as neurons, astrocytes, endothelial cells, or other cell types will definitely facilitate mature interactions and thus provide extra important understanding [Figure 2].
Figure 2. The versatile applications of human pluripotent stem cell-derived microglia. Since MGLs and their derivatives were first developed from hPSCs, they have been applied in a variety of disease model systems in a very short time. To date, the most prominent studies have been conducted in the context of modeling multiple neurodegenerative and neurodevelopmental diseases, omics and systematic analysis, interaction with other CNS cell types, as well as transplantation-based human-mouse chimerism. The most widespread application is the use of monocultured MGLs in dissecting the inflammatory endeavors underlying neurodegenerative diseases. Given the complex interactions between microglia and other CNS cells, hPSC-derived MGLs were further co-cultured with hPSC-derived neurons and astrocytes, or engrafted within hPSC-derived BOs, which largely lack microglia due to their different embryonic origins, to study human microglial functions in either health and disease state closer to brain microglia. Other than incorporating into in vitro cultured BOs, human MGLs can also be transplanted in vivo into a fully developed mammalian brain and largely repopulated and functionally integrated into the brain, producing extensive chimerism. The chimeric model is intended to faithfully study the pathophysiology of human microglia within a mature and intact milieu. Moreover, hPSC-derived MGLs also provide a viable platform for conducting functional genomics or drug screening. For example, a promising approach is enabled by CRISPR-based functional genomics in differentiated MGLs. Pooled CRISPRa and CRISPRi screens enable scalable modeling of changes in gene expression and discovery of regulatory mechanisms through gene screens. In addition, integrative omics analyses, including RNA-seq, ATAC-seq, ChIP-seq, as well as proteomics, have been performed based on this platform. BOs: Brain organoids; CNS: central nervous system; CRISPR: clustered regularly interspaced short palindromic repeats; CRISPRa: CRISPR-activation; CRISPRi: CRISPR interference; hPSC: human pluripotent stem cells; MGLs: microglia-like cells; WT: wild type.
Many studies recognized that their MGLs may have transcriptomic defects due to the absence of other brain cell types. Despite the fact that key microglial survival factors, such as CSF1 or IL34, were included in the culture medium for each MGL differentiation protocol, a large number of signaling factors and physical interactions were lacking. Other cytokines such as CX3CL1, CD200 and TGF-β, critical factors for keeping microglia homeostasis[32,53,68], were therefore added into the differentiation paradigm[40]. To further improve it, most protocols attempt to produce their MGLs closer to the microglial fate by the co-culture with astrocytes or neurons, which is able to induce significant changes in both MGL morphologies and transcriptome profiles[38-40,42,43,69].
In a recent study, Guttikonda et al. developed a novel method to generate microglia from hPSCs and established a defined hPSC-derived tri-culture system comprising pure populations of hPSC-derived MGLs, neurons and astrocytes, aiming to explore crosstalks associated with neuroinflammation[70]. Specifically, the tri-culture system was used with AD hPSCs bearing APPSWE+/+ mutations and their isogenic wild-type controls. Probably due to the initiation of reciprocal signaling by microglia and astrocytes in this system, they further uncovered that complement C3, which tightly associates with inflammatory responses and synaptic pruning, was potentiated in tri-culture and further enhanced in the APPSWE+/+ tri-cultures. This study thus successfully clarified, by the co-culture platform, the major cell type that is responsible for the increase in complement C3 in AD.
BRAIN ORGANOIDS AND CHIMERISM
In vitro brain organoids
Developing human in vitro models to study microglia has been a major challenge, with primary microglia or hPSC-derived microglia being viable options. Recent studies have shown that it might not be a wise strategy to study microglia function and associated phenotypes without considering the complex interactions between microglia and other cells in the brain. Elucidating the inner responses of microglia requires the production of MGLs within the brain milieu. To correct the in vivo environment-dependent defects in the MGL transcriptomes, studies have turned to investigating the possible use of hPSC-derived brain organoids (BOs) to study the function of human microglia in health and disease states[71-73].
However, previously described “mini-brains” showed that they lack microglia, which arise in the mesoderm, due to the ectodermal lineage that produces neuroepithelial cells. To further demonstrate microglial functions within the complex interactions of various cell types, in vitro BOs, particularly microglia-containing BOs, as also reviewed in another study[74], may provide an approximate brain environment and are recognized as the best in vitro model [Figure 2]. To address this, Abud et al. documented that MGLs migrate into and transplant within BOs when both are maintained in a co-culture system[40]. The transplanted MGLs, in turn, adopt a more divergent morphology than MGLs alone in vitro, and rapidly turned into an amoeboid morphology responding to the injury[75]. In recent years, MGLs or EMPs derived from hiPSCs have been used to co-culture with cortical and cerebral organoids, respectively, for modeling both brain development and disease pathogenesis[47,50,76-79]. These protocols generally require the time-consuming addition of expensive cytokines and growth factors during the differentiation of immune or progenitor cells, respectively.
Interestingly, a recent study by Ormel et al.[80] modified the original protocol[71,72] by reducing neural ectodermal stimulators and delaying matrigel coating to generate organoid-grown microglia (oMGs) that develop innately within the BOs. This finding was predicated on a scRNA-seq study, which showed that BOs contain a population of cells defined by mesodermal markers[81]. This suggests that the presence or absence of this population may affect the development of microglia within BOs. They validated that the BOs inherently developed cells expressing the myeloid transcription factor PU.1, the conical microglial marker IBA1, as well as the lysosomal marker CD68. The transcriptome profile of oMGs was shown to be similar to that of human microglia in vitro. Notably, the expression levels of homeostatic makers like P2RY12 and TMEM119 are still much lower in the oMGs, suggesting that some in vitro environmental effects may not be fully corrected[31,32].
The chimeric model
The counterpart to in vitro BOs may be a model involving in vivo transplantation of human MGLs into a fully developed mammalian brain. Neonatal transplanted human neural or microglial progenitor cells can be largely repopulated and functionally integrated into the brain or spinal cord of adult host rodents, producing extensive chimerism. This chimeric model is therefore intended to faithfully study the pathophysiology of human microglia within a mature and intact milieu [Figure 2].
Specifically, the hPSC-derived hematopoietic progenitor cells (HPCs) were delivered to neonatal mouse brains to develop hPSC microglial chimeric mouse brain models. Transplanted HPCs contribute to environment-dependent differentiation into microglia, and acquire the signatures of in vitro human microglia and the capability of responding to tissue injury[27]. Specifically, transplanted MGLs respond to peripheral LPS insults by elevating inflammatory genes including MSR1 and CD45. Additionally, they could rapidly migrate towards the injury site upon laser ablation. More importantly, the engrafted MGLs possessed a unique transcriptional profile, greatly different from that of murine microglia, when responding to Aβ plaques and revealed several human-specific responding genes.
Similarly, in another study, a chimeric mouse model was established by transplanting hPSC-derived primitive macrophage progenitor cells (PMPs) into the brains of newborn mice[50]. The scRNA-seq uncovered that the xenografted hPSC-derived MGLs largely retained human microglial properties, in which the expression profile is consistent with physiological human microglia and recapitulated the microglial heterogeneity. Importantly, the engrafted MGLs in the cerebral cortex manifested much more complex morphologies, participated in murine synaptic pruning, and also responded dynamically to cuprizone-induced demyelination[50].
By transcriptome analyses, it is also shown that the expression profile of engrafted MGLs resembles ex vivo human microglia at 2 months of age, and these MGLs have remedied transcriptome impairments observed in monocultured human MGLs[28]. In a scenario where the TREM2R47H mutation may not induce significant defects in MGLs in vitro, in vivo studies of engrafted hPSC-derived MGLs have shown that such mutation results in functional defects. To this end, MITRG-immunodeficient mice were transplanted with homozygous HPCsR47H at the neonatal stage, prompting the development of robust amyloid pathology within the mouse brain. Although the TREM2 expression is normal, the ability of MGLs to migrate to Aβ plaques is significantly deficient[27].
Despite these exciting advantages, however, the chimeric model also has its limitations. The most prominent one is the lack of peripheral adaptive immune system, since this model employs immunodeficient mice. As microglia also actively crosstalk with peripheral immune cells during the development of neurodegenerative diseases[82-84], this shall not be ignored when studying the disease pathology using the chimeric model. The caveat might be addressed by simultaneously transplanted microglia progenitors or BOs and hematopoietic stem progenitor cells (HSPCs), differentiated from the same hPSC line, into newborn immunodeficient mice. This strategy has been applied in a study exploring progressive human immunodeficiency virus type one (HIV-1) infection, in which human neuroglial progenitor cells (mainly astrocytes and oligodendrocytes) and HSPCs were injected into the lateral ventricle and liver, respectively[85]. This method established a new chimeric model with a chimeric brain and humanized peripheral immune system, which might be applied to MGL-related studies.
Collectively, these recent advances demonstrate that the generation of functional MGLs in vitro greatly dwarfs the bottleneck of prior acquisition of human microglia. However, while inducing MGLs in vitro may now be well-established, the environment-dependent nature of microglia and the limited studies comparing MGLs with reference microglia in vivo may fuel the field that may faithfully recapitulate the brain milieu.
VERSATILE APPLICATIONS OF hPSC-DERIVED MICROGLIA
Since MGLs were first induced from hPSCs, they have been applied in a variety of disease models in a very short time. To date, the most prominent studies have been conducted in modeling multiple neurodegenerative diseases and neurodevelopmental disorders, and a range of omics and functional genomic studies [Figure 2].
Disease modeling
Neurodegenerative diseases mainly include AD, PD, and ALS, among others. Of those, AD is the leading cause of about 60%-70% of dementia cases[86], and PD is the second most common neurodegenerative disease, affecting 2%-3% of the population over 65 years old[87,88]. Neurodegenerative disorders are characterized by a progressive decline in cognitive performance and/or motor performance that are caused by progressive neuronal death inside the CNS[89,90]. To be frustrated, very few effective treatments for those diseases are available. Dissecting the pathogenesis of neurodegenerative diseases is urgently needed to develop novel therapeutic strategies.
In the last decade, genetic variation in neurons has been recognized as an important causal factor in neurodegenerative diseases. However, amid the multi-omics era, microglia are increasingly emerging as potential disease drivers rather than passive participants. Particularly in recent years, genome-wide association studies (GWAS)[91-96] and multi-omics analysis[97,98] have uncovered an unexpected dominant role for microglial activation in increasing the risk of neurodegenerative diseases. For example, in a recent study, a GWAS analysis of a large number of AD cases and non-demented elderly people, with the largest sample involving 1,126,563 individuals, has demonstrated that many risk genes related to neurodegenerative diseases or preferentially or selectively expressed in microglia rather than other cell types in the brain[91,92,99,100]. It is thus necessary to understand the molecular underpinnings of disease-associated genetic variants and their potential effects in mediating microglial functions [Figure 1].
Thus, MGLs derived from hPSCs provide viable options for understanding human microglia biology and facilitate functional studies of GWAS risk genes, as MGLs express most CNS disease-related genes[40,101]. Of particular note, risk variants can be corrected by CRISPR/Cas9 genome editing to generate isogenic hPSCs with the same genetic background except for the variants [Figure 1]. This is extremely important, especially considering the genetic heterogeneity of the affected population.
Parkinson’s disease
PD is characterized by the accumulation of α-synuclein (α-Syn) in dopaminergic neurons. Release of oligomeric/fibrillar α-Syn from damaged neurons may potentiate neuronal death partially via microglial activation. One of the first applications of hPSC-derived MGLs in modeling diseases was dissecting the possible effects of several early-onset PD mutations in microglial functions[102]. MGLs were differentiated from iPSC lines reprogrammed from familial PD patients who possessed a triplet of the SNCA gene encoding α-Syn, or the SNCAA53T mutation. Induced MGLs were shown to phagocytose monomeric or fibrillar α-Syn; however, after endogenous overexpression or after the addition of monomeric α-Syn to the medium, this activity was greatly dampened in SNCA triploid mutants[102]. They further revealed that the endocytosis of monomeric α-Syn was actin-independent, whereas the fibrillar form was taken up through an actin-dependent pathway, thus hinting at new potential targets for aggregation clearance[102].
To investigate whether fibrillar α-Syn activates the NLRP3 inflammasome in human microglia. hPSC-derived MGLs were used as an in vitro model for α-Syn activation[103]. MGLs can be activated by the mutant α-SynA53T released by hPSC-derived dopaminergic neurons. Unexpectedly, α-Syn antibody complexes enhanced rather than inhibited inflammasome-mediated IL-1β release, suggesting that α-Syn antibodies are uniquely neuroinflammatory in a human context. Transplantation of MGLs within an α-Syn humanized mouse model led to caspase-1 activation and neural death, which was further aggravated by α-Syn antibodies. The findings may bring a caveat that therapies of depleting protein aggregates by antibodies may produce paradoxical inflammatory effects in human microglia.
Importantly, in a very recent study, Langston et al. found that the influence of PD-associated non-coding variants, as identified by GWAS, on leucine-rich repeat kinase 2 (LRRK2) expression is propagated exclusively through microglia, and not by other cell types in the brain[104]. By using hPSC-derived MGLs, they elucidated microglia-specific regulatory chromatin regions that regulate LRRK2 expression in human frontal cortex and substantia nigra. In addition, they applied a large-scale CRISPR interference (CRISPRi) screen in MGLs and identified a regulatory DNA element containing single nucleotide polymorphism (SNP) rs6581593 that affects LRRK2 expression. This study thus suggests that cell types should be considered when assessing the role of non-coding variants in disease pathogenesis and reveals mechanisms of association of non-coding regions of PD risk genes.
Alzheimer’s disease
As numerous microglia genes have recently been implicated in regulating AD risk[105-110], these studies carefully analyze risk genes to unravel the genetic basis of this disease. To date, most of the work using hPSC-derived MGLs has been devoted to dissecting the pathogenesis of AD. For example, Lin et al. investigated the impact of the APOE ε4 allele on the function of human microglia[76]. An APOE3/3 iPSC line was edited by CRISPR/Cas9 genome editing to produce an isogenic homozygous APOE4/4 line. This genetic change contributed to the dysregulation of more than 1,000 genes, as well as the suppression of the uptake of Aβ oligomers. These MGLs were then co-cultured with BOs, derived from amyloid-beta precursor protein (APP)-overexpressed iPSCs, and manifested diffuse amyloid pathology[111]. APOE3/3 MGLs within the BOs were shown to greatly reduce the number of Aβ, whereas the phagocytosis ability of APOE4/4 MGLs was dampened. Similarly, converting APOE4/4 into isogenic APOE3/3 by CRISPR/Cas9, in turn, recovered the phagocytic deficits.
The creation of isogenic APOE4 MGLs has also been applied in another recent study. By doing this, Victor et al. explored the interaction of microglia with neurons and revealed the refractory properties of
Triggering receptor expressed on myeloid cells-2 (TREM2), expressed on myeloid cells, is another key AD pathogenic gene, which is the most studied gene after the development of hPSC-derived MGLs. The membrane protein TREM2 regulates critical functions of microglia, including phagocytosis and chemotaxis, and plays a pivotal role in the pathogenesis of AD. The TREM2R47H mutation has been shown to possess a risk comparable to that of a single APOE ε4 allele[105,106]. One study has shown that TREM2 was transcribed into mRNA with altered splicing, and was significantly decreased in mice bearing a heterozygous TREM2R47H mutation[113]. However, human MGLs containing the identical mutations did not show TREM2 reduction or altered splicings. To further support this discrepancy, Xiang et al. demonstrated that human MGLs containing either heterozygous or homozygous TREM2 deletions exhibited defective phagocytosis[113]; however, MGLs containing heterozygous R47H variant did not recapitulate this phenotype. It is observed using both E. coli fragments and human Aβ plaques. Thus, those studies re-emphasized the importance of employing human models that faithfully recapitulate disease-associated risk genes/variants to enhance their translatability.
To fully dissect the role of TREM2 in human microglia, McQuade et al. obtained three isogenic TREM2-knockout (KO) iPSC lines using CRISPR/Cas9 and further differentiated these cells into MGLs to study the transcriptional and functional impact of TREM2 deletion[114]. They found increased susceptibility of TREM2-KO MGLs to M-CSF-dependent survival, impaired phagocytosis of disease-associated substrates such as APOE, and defects in CXCR4-mediated chemotaxis. Those collectively lead to an attenuated response to Aβ plaques. Single-cell sequencing of xenografted human microglia further documented the loss of disease-associated microglia (DAM) phenotype in human TREM2-KO microglia. Similarly, in another study, TREM2-KO MGLs exhibited exaggerated Ca2+ signaling in response to purinergic agonists (e.g., ADP) that shape the damage response of microglia[115]. Driven by increased expression of P2Y12 and P2Y13 receptors, this ADP hypersensitivity leads to greater Ca2+ release, which triggers sustained Ca2+ inflow and modulates the motility of TREM2-KO microglia to a greater extent.
Microglia apply oxidative phosphorylation (OXPHOS) to supply energy in a normal surveillance state, but undergo metabolic conversion to glycolysis, facilitating them to execute rapid responses. The role of TREM2 on microglia metabolic function was studied in patient-specific iPSC-derived MGLs carrying TREM2 loss-of-function variants[116]. They showed that MGLs with TREM2 variants (e.g., R47H) manifested profound metabolic defects, including reduced mitochondrial respiratory capacity and inability to undergo glycolytic immunometabolic conversion. Mechanistically, dysregulated PPARγ/p38MAPK signaling was observed to be caused by TREM2 variants, while activating those pathways may ameliorate the metabolic deficits in these cells and consequently rescue critical microglial functions such as Aβ phagocytosis.
Other AD risk genes have also been studied using this human model. For example, CD33, whose polymorphisms are associated with late-onset Alzheimer’s disease (LOAD), is expressed on myeloid immune cells, including microglia. A recent study analyzed CD33-KO hPSC-derived MGLs for cytokine expression, phagocytosis, and its associated oxidative burst[117]. CD33 depletion constitutively activated inflammation-associated pathways with increased phosphorylation of splenic tyrosine kinase (SYK) and extracellular signal-regulated kinases 1 and 2 (ERK1/2), as well as increased levels of IL-1β, IL-8, and IL-10. The phagocytosis and phagocytic oxidative burst of aggregated Aβ and bacterial particles were also increased after CD33 knockout. This study suggests that CD33 deletion is beneficial for phagocytosis of Aβ but potentially harmful for oxidative bursts and inflammation.
SNP rs616338 is another genetic variant recently identified by GWAS that results in a p.S209F amino acid substitution in the ABI3 gene[118]. ABI3 expression is higher in microglia than other CNS cell types, and is a structural component of the WAVE2 complex, which is known to regulate lamellipodia formation, membrane folding, and phagocytosis. This study obtained ABI3-KO iPSC lines and further ABI3-KO MGLs and found that ABI3-KO MGLs showed significantly lower uptake of Zymosan-conjugated pHrodo beads, reduced expression levels of the scavenger receptor MSR1 and increased expression levels of the glucose transporter SLC2A1. ABI3-KO cells were less responsive to ADP in the transwell migration assay despite higher mRNA expression of the P2RY12 receptor in KO cells. The lack of ABI3 did not obviously affect Aβ42 secretion, tau phosphorylation at Ser214 and APP phosphorylation at Thr668 in co-culture with hiPSC-derived neurons carrying the APPSwe mutant. The overall results suggest that ABI3 deficiency in microglia significantly impairs phagocytosis and ADP-induced migration, but produces limited effects on AD-like pathology.
In addition to deleterious risk variants, potentially protective variants in microglia are increasingly being identified. For example, the P522R variant of PLCG2 is associated with a reduced risk of AD[119]. To investigate this, chimeric AD and wild-type mice were established by transplanting PLCG2P522R or isogenic wild-type hPSC-derived MGLs. The PLCG2P522R variant greatly increased microglial human leukocyte antigen expression and induced antigen presentation, chemokine signaling and T cell proliferation pathways by single- and bulk-RNA sequencing as well as histological analysis. This study demonstrates that the PLCG2P522R variant enhances the ability of microglia to recruit T cells and present antigens, and notably primes a transcriptional profile that is shown to be reduced in the brains of AD patients.
Although the role of neurochemical pathways in microglia has been extensively studied, the mechanoreceptors that regulate microglial function remain largely unexplored. A very recent study used hPSC-derived MGLs to study PIEZO1, a mechanotransduction ion channel[120]. They found that PIEZO1 orchestrated the clearance of Aβ by improving phagocytosis, survival, and lysosomal activity. Specifically,
Amyotrophic lateral sclerosis
ALS is a fatal disease characterized pathologically by motor and cortical neurodegeneration and microgliosis. Mutations in the C9orf72 gene are the most common mutations in familial and sporadic ALS and familial fronto-temporal dementia (FTD). The role of C9orf72 mutations has been well-studied in neurons previously, with less in microglia. Recently, studies have generated C9orf72 ALS/FTD patient-derived iPSCs and differentiated them into MGLs[121]. Monocultures of C9orf72 MGLs had essentially similar transcriptome characteristics to control MGLs. However, when treated with Aβ or synaptosomes, the mutant MGLs manifested deficient phagocytic activity and exhibited exacerbated immune responses. They further found that the expression of endosomal markers and lysosome-associated membrane proteins was changed in mutant MGLs, as was seen in patient autopsy tissues.
The FUSP525L mutation is highly penetrant and leads to earlier onset and faster progression of ALS cases. To study its role in microglia, Kerk et al. used iPSC lines bearing FUSP525L mutation and their isogenic control lines to differentiate human MGLs[122]. They found that the mutation led to mislocalization of FUS proteins from nucleus to cytoplasm in MGLs. The mutation also caused changes in the transcriptome profile and revealed differentially expressed genes associated with microglial functions. For example, many chemoreceptor genes were altered and further dampened calcium signaling for chemoreceptor activation. The phagocytic ability and cytokine release were also examined but were not obviously affected in mutant MGLs.
Unlike variants in coding regions, risk loci in non-coding regions have been largely unexplored by genetic association studies; however, these risk loci may play a previously unappreciated but increasingly understood role in the pathogenesis of diseases. Recently, a study performed a region-based rare variant association analysis of 6139 ALS genome-wide untranslated regions (UTRs) and 70403 non-ALS controls[123]. They found that 3′UTR variants of IL-18 receptor accessory protein (IL18RAP) were significantly enriched in the control genomes and were associated with a 5-fold lower risk of ALS development. Mechanistically, the variant reduced mRNA stability and the binding of double-stranded RNA binding proteins. In addition, the variant per se attenuated the neurotoxicity of hiPSC-derived MGLs carrying the C9orf72 mutation by mediating the NF-κB signaling, thereby conferring neuroprotective advantages for motor neurons and rendering it a protective variant for ALS.
Down syndrome
Down syndrome (DS) is caused by the trisomy of human chromosome 21, and is featured by retarded brain development and serves as a common risk factor for AD, partly because the APP gene is located on chromosome 21[124]. Although microglia regulate synaptic plasticity during neurodevelopment, its role in DS and AS in DS (called DSAD) remains elusive. Previously, studying the role of microglia during brain development has been quite challenging due to the lack of an appropriate model; however, the generation of hPSC-derived MGLs provides a novel platform to study the concerted interactions among different cell types and their crosstalk in the human-brain-like environment over a long-term process of in vivo development.
Indeed, Jin et al. leveraged microglia-containing BOs and mouse chimeras and demonstrated that DS-MGLs showed defective development and functions[79]. Specifically, DS-MGLs exhibited larger soma size and branching impairment, as well as an enhanced synaptic pruning ability, and further displayed dystrophic and senescent phenotypes responding to pathological tau derived from DSAD human brain tissues. Furthermore, by RNA-sequencing, the type I interferon (IFN-I) signaling is shown to be upregulated in
Autism spectrum disorder
ASD is tightly associated with impaired synaptic pruning during brain development, and thus microglia is shown to be a key cell type that contributes to the disease pathology[10]. A recent study generated a chimeric platform to investigate the neuro-immune activities in ASD with macrocephaly. MGLs in this chimeric model were well survived for several months, and reached full maturation, closely resembling human microglia in situ[47]. The morphology of ASD-MGLs at 12 weeks post transplantation (wpt) is featured by increased soma size and primary process thickness, as well as increased filopodia, which is accompanied by a reactive phenotype. Furthermore, through the generation of ASD and control BOs and their incorporation into unaffected human microglia, it was observed that those microglia only responded to ASD-BOs in terms of morphology changes. This is a quite important notion that the cell-non-autonomous changes of microglial phenotypes were driven by the ASD brain environment, but not the intrinsic microglia genetic predisposition.
Multi-omics and systematic screens
One of the core advantages of hPSC-derived MGLs is that an exponential increase of cells can be harvested compared to traditional methods. In general, more than 100-fold MGLs can be obtained from the starting population of hPSCs[125]. Furthermore, the self-renewal biology of hPSCs could, in principle, provide an unlimited source of human MGLs that could be used for high-throughput experiments, such as omics analysis (transcriptomics, proteomics, and metabolomics) and systematic screening [Figure 2].
A recent study used CRISPR/Cas9 genome editing to generate hPSC lines with several AD variants at the INPP5D, TREM2, SORL1 and CD33 loci and further differentiated them into MGLs, in which AD-like expression profiles were recapitulated[98]. Note that all MGLs thus share the same genetic background, except those variants, which can reduce the genetic bias among individuals. They further combined integrative omics analyses, including RNA-seq, ATAC-seq, ChIP-seq, and proteomics, and found that the upregulation of APOE is a convergent pathogenic node of AD[98].
In addition to this, hPSC-derived MGLs provide a viable platform for conducting functional genomics or drug screening. A promising approach is enabled by CRISPR-based functional genomics in differentiated cells. Pooled CRISPR-activation (CRISPRa) and CRISPRi screens enable scalable modeling of changes in gene expression and discovery of regulatory mechanisms through gene screens. Such a platform was recently established using overexpressed transcription factors to directly convert cell fate[126]. Specifically, they selected six transcription factors (PU.1, MAFB, CEBPA, CEBPB, IRF5, and IRF8) that are highly expressed in human microglia, and engineered them into an iPSC line within two integrated cassettes at the safe harbor loci for doxycycline-induced expression. This allowed the differentiation process of MGLs to take only 8 days.
On this basis, inducible CRISPRa/i machinery was stably integrated into the safe harbor loci of hPSCs, respectively, to enable robust overexpression and knockdown of endogenous genes, as well as large-scale loss-of-function (LOF) and gain-of-function (GOF) gene screens[126]. On this platform, pooled CRISPRa/i screens were performed to discover potential regulators of microglial survival, proliferation, phagocytosis, and inflammatory activation. Screens with scRNA-seq as a readout showed that these microglia adopted a range of states reflecting those observed in the human brain and identified regulators of specific states, which could enable functional characterization and therapeutic targeting of these states. Thus, hPSC-derived MGLs confer a large-scale, multi-modal CRISPRa/i-based genetic screen that can systematically identify regulators of microglia states and their functions.
CONCLUSIONS AND PERSPECTIVES
With advances in technologies such as hPSC cultivation, scRNA-seq, CRISPR/Cas9 systems and lineage tracing, research around microglia has generated substantial growth in our understanding. In particular, with the recent development of robust methods for studying hPSC-derived MGLs and BOs, researchers now have more optional strategies available to verify and expand previous findings in rodents.
Revisiting the protocols
As we described earlier, there are a range of protocols in use, and depending on the study, subtle differences may be introduced that may bring varied relevance to the human context[26,56]. Studies have demonstrated that co-culture with other cell types or adding supplements into the medium further primes MGLs towards a more accurate microglia fate; however, more studies are required to reveal how deficiencies are corrected. In some protocols, hPSCs may have been differentiated into monocytes[127]. These cells are then exposed to extracellular cues that lead to macrophages with microglial features or later trans-differentiate into MGLs. However, the use of monocytes as a model for human microglia may be controversial because of the different ontogenetic origins of microglia and peripheral macrophages. Therefore, no consensus has been reached regarding a unique method for generating hPSC-derived MGLs.
The production of large numbers of MGLs from hPSCs is an important and seemingly initial step in understanding how human microglia behave. The human MGLs hold great promise for dissecting the potential functions of a growing number of risk loci associated with various neurological diseases[93-96]. The platform will thus be important for translational research and drug screening as well as for the development of precision medicines. In addition, human MGLs might also be promising regenerative agents in transplantation therapies, as hPSC-derived neurons are.
Beyond this, it is important to combine the high-throughput in vitro assays and other in situ or in vivo findings within more complicated and/or accurate models such as BOs or human-mouse chimeras. The development and use of this paradigm will likely yield more translational results to humans.
Considering the aging status
As hPSCs were basically reprogrammed from somatic cells, either young or aged donor cells, the aging features were largely rejuvenated[128-130]. Indeed, when cultured alone or co-cultured with neurons and astrocytes cultured in two-dimensional monolayer or three-dimensional organoids, hPSC-derived MGLs are most similar to fetal or early postnatal human microglia. The expression of key microglial markers such as TREM2, P2RY12, and TMEM119 in hPSC-derived MGLs was significantly lower compared to microglia isolated from adult brain tissue[131]. As such, MGLs and their aging status might be scrutinized in future studies, especially when modeling the pathogenesis of neurodegenerative diseases.
Several avenues, however, might be employed to render cellular senescence, for instance, by introducing progerin, a truncated Lamin A protein that causes the pathology of Hutchinson-Gilford progeria syndrome (HGPS)[132], or by telomerase manipulation[133]. The treatment with chemicals, such as hydroxyurea, triggers senescence featured by increased β-Gal staining and ROS production, decreased proliferation, and DNA damage profiles[134]. However, given the sensitivity of MGLs to stimuli, the use of chemical induction methods may warrant caution.
Alternatively, maintaining the cellular aging status has been achieved by direct reprogramming of adult cells in recent years[34,135,137]. Typical reprogramming events were performed on adult fibroblasts and astrocytes that can be converted to neurons[137-139]. However, reprogramming microglia to neurons has been controversial, or furthermore, proved unsuccessful under a stringent lineage-tracing condition[140,141]. The extra challenge with the microglia reprogramming events might come from the cross-lineage barrier if looking at the epigenetic landscape of neural development. As such, direct reprogramming of adult cells into microglia would be, in a similar fashion, much trickier. To address this, continuous endeavors will have to be devoted.
DECLARATIONS
Author’s contributionConceived this study: Tang Y
Prepared the draft and figures: Wu J, Tang Y
All authors reviewed and approved the final manuscript.
Availability of data and materialsNot applicable.
Financial support and sponsorshipThis study was funded by National Key R&D Program of China (No. 2022ZD0213700), National Natural Sciences Foundation of China (No. 82271280 to Tang Y and No. 82301433 to Wu J), Hunan Provincial Natural Science Foundation of China (No. 2022JJ40824 to Wu J), Hunan Provincial Health Commission (No. B202303070054 to Tang Y), Talents Startup Fund (No. 2209090550 to Tang Y), and Youth Science Fund (No. 2021Q04 to Wu J) of Xiangya Hospital, Central South University, Changsha, China.
Conflicts of interestAll authors declared that there are no conflicts of interest.
Ethical approval and consent to participateNot applicable.
Consent for publicationNot applicable.
Copyright© The Author(s) 2023.
REFERENCES
1. Ginhoux F, Greter M, Leboeuf M, et al. Fate mapping analysis reveals that adult microglia derive from primitive macrophages. Science 2010;330:841-5.
2. Gomez Perdiguero E, Klapproth K, Schulz C, et al. Tissue-resident macrophages originate from yolk-sac-derived erythro-myeloid progenitors. Nature 2015;518:547-51.
3. Salter MW, Stevens B. Microglia emerge as central players in brain disease. Nat Med 2017;23:1018-27.
4. Barger N, Keiter J, Kreutz A, et al. Microglia: an intrinsic component of the proliferative zones in the fetal rhesus monkey (Macaca mulatta) cerebral cortex. Cereb Cortex 2019;29:2782-96.
5. Ransohoff RM, Cardona AE. The myeloid cells of the central nervous system parenchyma. Nature 2010;468:253-62.
6. Noctor SC, Penna E, Shepherd H, et al. Periventricular microglial cells interact with dividing precursor cells in the nonhuman primate and rodent prenatal cerebral cortex. J Comp Neurol 2019;527:1598-609.
7. Cunningham CL, Martínez-Cerdeño V, Noctor SC. Microglia regulate the number of neural precursor cells in the developing cerebral cortex. J Neurosci 2013;33:4216-33.
8. Paolicelli RC, Bolasco G, Pagani F, et al. Synaptic pruning by microglia is necessary for normal brain development. Science 2011;333:1456-8.
9. Schafer DP, Lehrman EK, Kautzman AG, et al. Microglia sculpt postnatal neural circuits in an activity and complement-dependent manner. Neuron 2012;74:691-705.
10. Lukens JR, Eyo UB. Microglia and neurodevelopmental disorders. Annu Rev Neurosci 2022;45:425-45.
11. Sekar A, Bialas AR, de Rivera H, et al. Schizophrenia risk from complex variation of complement component 4. Nature 2016;530:177-83.
12. Neniskyte U, Gross CT. Errant gardeners: glial-cell-dependent synaptic pruning and neurodevelopmental disorders. Nat Rev Neurosci 2017;18:658-70.
13. Koizumi S, Shigemoto-Mogami Y, Nasu-Tada K, et al. UDP acting at P2Y6 receptors is a mediator of microglial phagocytosis. Nature 2007;446:1091-5.
14. Nimmerjahn A, Kirchhoff F, Helmchen F. Resting microglial cells are highly dynamic surveillants of brain parenchyma in vivo. Science 2005;308:1314-8.
15. Perry VH, Nicoll JAR, Holmes C. Microglia in neurodegenerative disease. Nat Rev Neurol 2010;6:193-201.
17. Tang Y, Le W. Differential roles of M1 and M2 microglia in neurodegenerative diseases. Mol Neurobiol 2016;53:1181-94.
18. Le W, Wu J, Tang Y. Protective microglia and their regulation in Parkinson’s disease. Front Mol Neurosci 2016;9:89.
19. Li C, Ren J, Zhang M, et al. The heterogeneity of microglial activation and its epigenetic and non-coding RNA regulations in the immunopathogenesis of neurodegenerative diseases. Cell Mol Life Sci 2022;79:511.
20. Wolf SA, Boddeke HWGM, Kettenmann H. Microglia in physiology and disease. Annu Rev Physiol 2017;79:619-43.
21. Masuda T, Sankowski R, Staszewski O, et al. Spatial and temporal heterogeneity of mouse and human microglia at single-cell resolution. Nature 2019;566:388-92.
23. Dawson TM, Golde TE, Lagier-Tourenne C. Animal models of neurodegenerative diseases. Nat Neurosci 2018;21:1370-9.
24. Friedman BA, Srinivasan K, Ayalon G, et al. Diverse brain myeloid expression profiles reveal distinct microglial activation states and aspects of Alzheimer’s disease not evident in mouse models. Cell Rep 2018;22:832-47.
25. Ueda Y, Gullipalli D, Song WC. Modeling complement-driven diseases in transgenic mice: values and limitations. Immunobiology 2016;221:1080-90.
26. Hasselmann J, Blurton-Jones M. Human iPSC-derived microglia:a growing toolset to study the brain’s innate immune cells. Glia 2020;68:721-39.
27. Hasselmann J, Coburn MA, England W, et al. Development of a chimeric model to study and manipulate human microglia in vivo. Neuron 2019;103:1016-33.e10.
28. Gosselin D, Skola D, Coufal NG, et al. An environment-dependent transcriptional network specifies human microglia identity. Science 2017;356:eaal3222.
29. Galatro TF, Holtman IR, Lerario AM, et al. Transcriptomic analysis of purified human cortical microglia reveals age-associated changes. Nat Neurosci 2017;20:1162-71.
30. Burns TC, Li MD, Mehta S, Awad AJ, Morgan AA. Mouse models rarely mimic the transcriptome of human neurodegenerative diseases: a systematic bioinformatics-based critique of preclinical models. Eur J Pharmacol 2015;759:101-17.
31. Bohlen CJ, Bennett FC, Tucker AF, Collins HY, Mulinyawe SB, Barres BA. Diverse requirements for microglial survival, specification, and function revealed by defined-medium cultures. Neuron 2017;94:759-73.e8.
32. Butovsky O, Jedrychowski MP, Moore CS, et al. Identification of a unique TGF-β-dependent molecular and functional signature in microglia. Nat Neurosci 2014;17:131-43.
33. Takahashi K, Tanabe K, Ohnuki M, et al. Induction of pluripotent stem cells from adult human fibroblasts by defined factors. Cell 2007;131:861-72.
34. Tang Y, Liu ML, Zang T, Zhang CL. Direct reprogramming rather than iPSC-based reprogramming maintains aging hallmarks in human motor neurons. Front Mol Neurosci 2017;10:359.
35. Shi Y, Inoue H, Wu JC, Yamanaka S. Induced pluripotent stem cell technology: a decade of progress. Nat Rev Drug Discov 2017;16:115-30.
36. Lee G, Studer L. Induced pluripotent stem cell technology for the study of human disease. Nat Methods 2010;7:25-7.
37. Fernando MB, Ahfeldt T, Brennand KJ. Modeling the complex genetic architectures of brain disease. Nat Genet 2020;52:363-9.
38. Muffat J, Li Y, Yuan B, et al. Efficient derivation of microglia-like cells from human pluripotent stem cells. Nat Med 2016;22:1358-67.
39. Pandya H, Shen MJ, Ichikawa DM, et al. Differentiation of human and murine induced pluripotent stem cells to microglia-like cells. Nat Neurosci 2017;20:753-9.
40. Abud EM, Ramirez RN, Martinez ES, et al. iPSC-derived human microglia-like cells to study neurological diseases. Neuron 2017;94:278-93.e9.
41. Douvaras P, Sun B, Wang M, et al. Directed differentiation of human pluripotent stem cells to microglia. Stem Cell Reports 2017;8:1516-24.
42. Haenseler W, Sansom SN, Buchrieser J, et al. A highly efficient human pluripotent stem cell microglia model displays a neuronal-co-culture-specific expression profile and inflammatory response. Stem Cell Reports 2017;8:1727-42.
43. Takata K, Kozaki T, Lee CZW, et al. Induced-pluripotent-stem-cell-derived primitive macrophages provide a platform for modeling tissue-resident macrophage differentiation and function. Immunity 2017;47:183-98.e6.
44. Park J, Wetzel I, Marriott I, et al. A 3D human triculture system modeling neurodegeneration and neuroinflammation in Alzheimer’s disease. Nat Neurosci 2018;21:941-51.
45. Brownjohn PW, Smith J, Solanki R, et al. Functional studies of missense TREM2 mutations in human stem cell-derived microglia. Stem Cell Reports 2018;10:1294-307.
46. Xu R, Boreland AJ, Li X, et al. Developing human pluripotent stem cell-based cerebral organoids with a controllable microglia ratio for modeling brain development and pathology. Stem Cell Reports 2021;16:1923-37.
47. Schafer ST, Mansour AA, Schlachetzki JCM, et al. An in vivo neuroimmune organoid model to study human microglia phenotypes. Cell 2023;186:2111-26.e20.
48. Mancuso R, Van Den Daele J, Fattorelli N, et al. Stem-cell-derived human microglia transplanted in mouse brain to study human disease. Nat Neurosci 2019;22:2111-6.
49. Svoboda DS, Barrasa MI, Shu J, et al. Human iPSC-derived microglia assume a primary microglia-like state after transplantation into the neonatal mouse brain. Proc Natl Acad Sci U S A 2019;116:25293-303.
50. Xu R, Li X, Boreland AJ, et al. Human iPSC-derived mature microglia retain their identity and functionally integrate in the chimeric mouse brain. Nat Commun 2020;11:1577.
51. Fattorelli N, Martinez-Muriana A, Wolfs L, Geric I, De Strooper B, Mancuso R. Stem-cell-derived human microglia transplanted into mouse brain to study human disease. Nat Protoc 2021;16:1013-33.
52. Jiang P, Turkalj L, Xu R. High-fidelity modeling of human microglia with pluripotent stem cells. Cell Stem Cell 2020;26:629-31.
53. Kierdorf K, Erny D, Goldmann T, et al. Microglia emerge from erythromyeloid precursors via Pu.1- and Irf8-dependent pathways. Nat Neurosci 2013;16:273-80.
54. Schulz C, Gomez Perdiguero E, Chorro L, et al. A lineage of myeloid cells independent of Myb and hematopoietic stem cells. Science 2012;336:86-90.
55. Timmerman R, Burm SM, Bajramovic JJ. An overview of in vitro methods to study microglia. Front Cell Neurosci 2018;12:242.
56. Speicher AM, Wiendl H, Meuth SG, Pawlowski M. Generating microglia from human pluripotent stem cells: novel in vitro models for the study of neurodegeneration. Mol Neurodegener 2019;14:46.
57. Chen SW, Hung YS, Fuh JL, et al. Efficient conversion of human induced pluripotent stem cells into microglia by defined transcription factors. Stem Cell Reports 2021;16:1363-80.
58. Speicher AM, Korn L, Csatári J, et al. Deterministic programming of human pluripotent stem cells into microglia facilitates studying their role in health and disease. Proc Natl Acad Sci U S A 2022;119:e2123476119.
59. Davalos D, Grutzendler J, Yang G, et al. ATP mediates rapid microglial response to local brain injury in vivo. Nat Neurosci 2005;8:752-8.
60. Dolan MJ, Therrien M, Jereb S, et al. Exposure of iPSC-derived human microglia to brain substrates enables the generation and manipulation of diverse transcriptional states in vitro. Nat Immunol 2023;24:1382-90.
61. Liddelow SA, Guttenplan KA, Clarke LE, et al. Neurotoxic reactive astrocytes are induced by activated microglia. Nature 2017;541:481-7.
62. Rothhammer V, Borucki DM, Tjon EC, et al. Microglial control of astrocytes in response to microbial metabolites. Nature 2018;557:724-8.
63. Marinelli S, Basilico B, Marrone MC, Ragozzino D. Microglia-neuron crosstalk: signaling mechanism and control of synaptic transmission. Semin Cell Dev Biol 2019;94:138-51.
64. Sheridan GK, Murphy KJ. Neuron-glia crosstalk in health and disease: fractalkine and CX3CR1 take centre stage. Open Biol 2013;3:130181.
65. Stankovic ND, Teodorczyk M, Ploen R, Zipp F, Schmidt MHH. Microglia-blood vessel interactions: a double-edged sword in brain pathologies. Acta Neuropathol 2016;131:347-63.
66. Yousef H, Czupalla CJ, Lee D, et al. Aged blood impairs hippocampal neural precursor activity and activates microglia via brain endothelial cell VCAM1. Nat Med 2019;25:988-1000.
67. Merlini M, Rafalski VA, Rios Coronado PE, et al. Fibrinogen induces microglia-mediated spine elimination and cognitive impairment in an Alzheimer’s disease model. Neuron 2019;101:1099-108.e6.
68. Cardona AE, Pioro EP, Sasse ME, et al. Control of microglial neurotoxicity by the fractalkine receptor. Nat Neurosci 2006;9:917-24.
69. Muffat J, Li Y, Omer A, et al. Human induced pluripotent stem cell-derived glial cells and neural progenitors display divergent responses to Zika and dengue infections. Proc Natl Acad Sci U S A 2018;115:7117-22.
70. Guttikonda SR, Sikkema L, Tchieu J, et al. Fully defined human pluripotent stem cell-derived microglia and tri-culture system model C3 production in Alzheimer’s disease. Nat Neurosci 2021;24:343-54.
71. Lancaster MA, Renner M, Martin CA, et al. Cerebral organoids model human brain development and microcephaly. Nature 2013;501:373-9.
72. Lancaster MA, Knoblich JA. Generation of cerebral organoids from human pluripotent stem cells. Nat Protoc 2014;9:2329-40.
73. Mansour AA, Gonçalves JT, Bloyd CW, et al. An in vivo model of functional and vascularized human brain organoids. Nat Biotechnol 2018;36:432-41.
74. Zhang W, Jiang J, Xu Z, et al. Microglia-containing human brain organoids for the study of brain development and pathology. Mol Psychiatry 2023;28:96-107.
75. Karperien A, Ahammer H, Jelinek HF. Quantitating the subtleties of microglial morphology with fractal analysis. Front Cell Neurosci 2013;7:3.
76. Lin YT, Seo J, Gao F, et al. APOE4 causes widespread molecular and cellular alterations associated with Alzheimer’s disease phenotypes in human iPSC-derived brain cell types. Neuron 2018;98:1141-54.e7.
77. Fagerlund I, Dougalis A, Shakirzyanova A, et al. Microglia-like cells promote neuronal functions in cerebral organoids. Cells 2021;11:124.
78. Song L, Yuan X, Jones Z, et al. Functionalization of brain region-specific spheroids with isogenic microglia-like cells. Sci Rep 2019;9:11055.
79. Jin M, Xu R, Wang L, et al. Type-I-interferon signaling drives microglial dysfunction and senescence in human iPSC models of Down syndrome and Alzheimer’s disease. Cell Stem Cell 2022;29:1135-53.e8.
80. Ormel PR, de Sá RV, van Bodegraven EJ, et al. Microglia innately develop within cerebral organoids. Nat Commun 2018;9:4167.
81. Quadrato G, Nguyen T, Macosko EZ, et al. Cell diversity and network dynamics in photosensitive human brain organoids. Nature 2017;545:48-53.
82. Unger MS, Schernthaner P, Marschallinger J, Mrowetz H, Aigner L. Microglia prevent peripheral immune cell invasion and promote an anti-inflammatory environment in the brain of APP-PS1 transgenic mice. J Neuroinflammation 2018;15:274.
83. DeMaio A, Mehrotra S, Sambamurti K, Husain S. The role of the adaptive immune system and T cell dysfunction in neurodegenerative diseases. J Neuroinflammation 2022;19:251.
84. Marsh SE, Abud EM, Lakatos A, et al. The adaptive immune system restrains Alzheimer’s disease pathogenesis by modulating microglial function. Proc Natl Acad Sci U S A 2016;113:E1316-25.
85. Li W, Gorantla S, Gendelman HE, Poluektova LY. Systemic HIV-1 infection produces a unique glial footprint in humanized mouse brains. Dis Model Mech 2017;10:1489-502.
87. Ascherio A, Schwarzschild MA. The epidemiology of Parkinson’s disease: risk factors and prevention. Lancet Neurol 2016;15:1257-72.
90. Zaletel I, Schwirtlich M, Perović M, et al. Early impairments of hippocampal neurogenesis in 5xFAD mouse model of Alzheimer’s disease are associated with altered expression of SOXB transcription factors. J Alzheimers Dis 2018;65:963-76.
91. Seshadri S, Fitzpatrick AL, Ikram MA, et al. Genome-wide analysis of genetic loci associated with Alzheimer disease. JAMA 2010;303:1832-40.
92. Wightman DP, Jansen IE, Savage JE, et al. Largest GWAS (N=1,126,563) of Alzheimer’s disease implicates microglia and immune cells. medRxiv. [Preprint.] November 23, 2020 [accessed 2023 September 6]. Available from: https://www.medrxiv.org/content/10.1101/2020.11.20.20235275v1.
93. Kosoy R, Fullard JF, Zeng B, et al. Genetics of the human microglia regulome refines Alzheimer’s disease risk loci. Nat Genet 2022;54:1145-54.
94. de Paiva Lope K, Snijders GJL, Humphrey J, et al. Genetic analysis of the human microglial transcriptome across brain regions, aging and disease pathologies. Nat Genet 2022;54:4-17.
95. Young AMH, Kumasaka N, Calvert F, et al. A map of transcriptional heterogeneity and regulatory variation in human microglia. Nat Genet 2021;53:861-8.
96. Tansey KE, Cameron D, Hill MJ. Genetic risk for Alzheimer’s disease is concentrated in specific macrophage and microglial transcriptional networks. Genome Med 2018;10:14.
97. Morabito S, Miyoshi E, Michael N, et al. Single-nucleus chromatin accessibility and transcriptomic characterization of Alzheimer’s disease. Nat Genet 2021;53:1143-55.
98. Liu T, Zhu B, Liu Y, et al. Multi-omic comparison of Alzheimer’s variants in human ESC-derived microglia reveals convergence at APOE. J Exp Med 2020;217:e20200474.
99. Bertram L, Lange C, Mullin K, et al. Genome-wide association analysis reveals putative Alzheimer’s disease susceptibility loci in addition to APOE. Am J Hum Genet 2008;83:623-32.
100. Yu JT, Jiang T, Wang YL, et al. Triggering receptor expressed on myeloid cells 2 variant is rare in late-onset Alzheimer’s disease in Han Chinese individuals. Neurobiol Aging 2014;35:937.e1-3.
101. Cakir B, Tanaka Y, Kiral FR, et al. Expression of the transcription factor PU.1 induces the generation of microglia-like cells in human cortical organoids. Nat Commun 2022;13:430.
102. Haenseler W, Zambon F, Lee H, et al. Excess α-synuclein compromises phagocytosis in iPSC-derived macrophages. Sci Rep 2017;7:9003.
103. Trudler D, Nazor KL, Eisele YS, et al. Soluble α-synuclein-antibody complexes activate the NLRP3 inflammasome in hiPSC-derived microglia. Proc Natl Acad Sci U S A 2021;118:e2025847118.
104. Langston RG, Beilina A, Reed X, et al. Association of a common genetic variant with Parkinson’s disease is mediated by microglia. Sci Transl Med 2022;14:eabp8869.
105. Guerreiro R, Wojtas A, Bras J, et al. TREM2 variants in Alzheimer’s disease. N Engl J Med 2013;368:117-27.
106. Jonsson T, Stefansson H, Steinberg S, et al. Variant of TREM2 associated with the risk of Alzheimer’s disease. N Engl J Med 2013;368:107-16.
107. Lambert JC, Ibrahim-Verbaas CA, Harold D, et al. Meta-analysis of 74,046 individuals identifies 11 new susceptibility loci for Alzheimer’s disease. Nat Genet 2013;45:1452-8.
108. Efthymiou AG, Goate AM. Late onset Alzheimer’s disease genetics implicates microglial pathways in disease risk. Mol Neurodegener 2017;12:43.
109. Jansen IE, Savage JE, Watanabe K, et al. Genome-wide meta-analysis identifies new loci and functional pathways influencing Alzheimer’s disease risk. Nat Genet 2019;51:404-13.
110. Kunkle BW, Grenier-Boley B, Sims R, et al. Genetic meta-analysis of diagnosed Alzheimer’s disease identifies new risk loci and implicates Aβ, tau, immunity and lipid processing. Nat Genet 2019;51:414-30.
111. Raja WK, Mungenast AE, Lin YT, et al. Self-organizing 3D human neural tissue derived from induced pluripotent stem cells recapitulate Alzheimer’s disease phenotypes. PLoS One 2016;11:e0161969.
112. Victor MB, Leary N, Luna X, et al. Lipid accumulation induced by APOE4 impairs microglial surveillance of neuronal-network activity. Cell Stem Cell 2022;29:1197-212.e8.
113. Xiang X, Piers TM, Wefers B, et al. The Trem2 R47H Alzheimer’s risk variant impairs splicing and reduces Trem2 mRNA and protein in mice but not in humans. Mol Neurodegener 2018;13:49.
114. McQuade A, Kang YJ, Hasselmann J, et al. Gene expression and functional deficits underlie TREM2-knockout microglia responses in human models of Alzheimer’s disease. Nat Commun 2020;11:5370.
115. Jairaman A, McQuade A, Granzotto A, et al. TREM2 regulates purinergic receptor-mediated calcium signaling and motility in human iPSC-derived microglia. Elife 2022;11:e73021.
116. Piers TM, Cosker K, Mallach A, et al. A locked immunometabolic switch underlies TREM2 R47H loss of function in human iPSC-derived microglia. FASEB J 2020;34:2436-50.
117. Wißfeld J, Nozaki I, Mathews M, et al. Deletion of Alzheimer’s disease-associated CD33 results in an inflammatory human microglia phenotype. Glia 2021;69:1393-412.
118. Rolova T, Zhang F, Koskuvi M, Koistinaho J. The role of ABI3 in human induced pluripotent stem cell-derived microglia. Alzheimers Dement 2020;16:e047270.
119. Claes C, England WE, Danhash EP, et al. The P522R protective variant of PLCG2 promotes the expression of antigen presentation genes by human microglia in an Alzheimer’s disease mouse model. Alzheimers Dement 2022;18:1765-78.
120. Jäntti H, Sitnikova V, Ishchenko Y, et al. Microglial amyloid beta clearance is driven by PIEZO1 channels. J Neuroinflammation 2022;19:147.
121. Lorenzini I, Alsop E, Levy J, et al. Cellular and molecular phenotypes of C9orf72ALS/FTD patient derived iPSC-microglia mono-cultures. bioRxiv. [Preprint.] July 18, 2022 [accessed 2023 September 6]. Available from: https://www.biorxiv.org/content/10.1101/2020.09.03.277459v2.full.
122. Kerk SY, Bai Y, Smith J, et al. Homozygous ALS-linked FUS P525L mutations cell- autonomously perturb transcriptome profile and chemoreceptor signaling in human iPSC microglia. Stem Cell Rep 2022;17:678-92.
123. Eitan C, Siany A, Barkan E, et al. Whole-genome sequencing reveals that variants in the Interleukin 18 Receptor Accessory Protein 3'UTR protect against ALS. Nat Neurosci 2022;25:433-45.
124. Lott IT, Head E. Dementia in Down syndrome: unique insights for Alzheimer disease research. Nat Rev Neurol 2019;15:135-47.
125. McQuade A, Coburn M, Tu CH, Hasselmann J, Davtyan H, Blurton-Jones M. Development and validation of a simplified method to generate human microglia from pluripotent stem cells. Mol Neurodegener 2018;13:67.
126. Dräger NM, Sattler SM, Huang CTL, et al. A CRISPRi/a platform in human iPSC-derived microglia uncovers regulators of disease states. Nat Neurosci 2022;25:1149-62.
127. Capotondo A, Milazzo R, Garcia-Manteiga JM, et al. Intracerebroventricular delivery of hematopoietic progenitors results in rapid and robust engraftment of microglia-like cells. Sci Adv 2017;3:e1701211.
128. Marion RM, Strati K, Li H, et al. Telomeres acquire embryonic stem cell characteristics in induced pluripotent stem cells. Cell Stem Cell 2009;4:141-54.
129. Studer L, Vera E, Cornacchia D. Programming and reprogramming cellular age in the era of induced pluripotency. Cell Stem Cell 2015;16:591-600.
130. Mahmoudi S, Brunet A. Aging and reprogramming: a two-way street. Curr Opin Cell Biol 2012;24:744-56.
131. Olah M, Patrick E, Villani AC, et al. A transcriptomic atlas of aged human microglia. Nat Commun 2018;9:539.
132. Miller JD, Ganat YM, Kishinevsky S, et al. Human iPSC-based modeling of late-onset disease via progerin-induced aging. Cell Stem Cell 2013;13:691-705.
133. Vera E, Bosco N, Studer L. Generating late-onset human iPSC-based disease models by inducing neuronal age-related phenotypes through telomerase manipulation. Cell Rep 2016;17:1184-92.
134. Dong CM, Wang XL, Wang GM, et al. A stress-induced cellular aging model with postnatal neural stem cells. Cell Death Dis 2014;5:e1116.
135. Mertens J, Paquola ACM, Ku M, et al. Directly reprogrammed human neurons retain aging-associated transcriptomic signatures and reveal age-related nucleocytoplasmic defects. Cell Stem Cell 2015;17:705-18.
136. Victor MB, Richner M, Olsen HE, et al. Striatal neurons directly converted from Huntington’s disease patient fibroblasts recapitulate age-associated disease phenotypes. Nat Neurosci 2018;21:341-52.
137. Gascón S, Masserdotti G, Russo GL, Götz M. Direct neuronal reprogramming: achievements, hurdles, and new roads to success. Cell Stem Cell 2017;21:18-34.
138. Wang H, Yang Y, Liu J, Qian L. Direct cell reprogramming: approaches, mechanisms and progress. Nat Rev Mol Cell Biol 2021;22:410-24.
139. Yang SG, Wang XW, Qian C, Zhou FQ. Reprogramming neurons for regeneration: the fountain of youth. Prog Neurobiol 2022;214:102284.
140. Matsuda T, Irie T, Katsurabayashi S, et al. Pioneer factor NeuroD1 rearranges transcriptional and epigenetic profiles to execute microglia-neuron conversion. Neuron 2019;101:472-85.e7.
Cite This Article
How to Cite
Download Citation
Export Citation File:
Type of Import
Tips on Downloading Citation
Citation Manager File Format
Type of Import
Direct Import: When the Direct Import option is selected (the default state), a dialogue box will give you the option to Save or Open the downloaded citation data. Choosing Open will either launch your citation manager or give you a choice of applications with which to use the metadata. The Save option saves the file locally for later use.
Indirect Import: When the Indirect Import option is selected, the metadata is displayed and may be copied and pasted as needed.
About This Article
Copyright
Data & Comments
Data
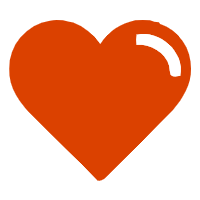
Comments
Comments must be written in English. Spam, offensive content, impersonation, and private information will not be permitted. If any comment is reported and identified as inappropriate content by OAE staff, the comment will be removed without notice. If you have any queries or need any help, please contact us at [email protected].