RNA基因治疗在肝癌中的应用:现状和临床展望(translate by chatgpt 4.0)
摘要 (translate by chatgpt 4.0)
关键词 (translate by chatgpt 4.0)
INTRODUCTION
Primary liver cancer is one of the most prevalent cancers worldwide. With a total of 905,677 newly registered cases and 830,180 deaths from liver cancer--the third highest number of cancer-related deaths--liver cancer was the sixth most common entity tumor in the world in 2020[1]. Of the various rare forms of primary liver cancer, hepatocellular carcinoma (HCC) accounts for 75%-85% of cases, while intrahepatic cholangiocarcinoma accounts for 10%-15% of cases[2]. Patients with early-stage hepatocellular carcinoma can benefit from radical interventions such as surgery, ablation, and liver transplantation.
The currently approved treatments for HCC include sorafenib, levatinib, regorafenib, and cabozantinib. These medications target tyrosine kinase growth factor receptors and/or serine/threonine kinases associated with angiogenesis and cell proliferation. Patients treated with sorafenib showed a significant improvement in both overall and progression-free survival, according to the study[3]. Median overall survival was 10.7 months in the sorafenib group and 7.9 months in the placebo group (hazard ratio in the sorafenib group, 0.69; 95% confidence interval, 0.55 to 0.87; P < 0.001). There was no significant difference between the two groups in the median time to symptomatic progression (4.1 months vs. 4.9 months, P = 0.77). Nonetheless, a noteworthy segment of patients encountered toxic reactions, leading to the cessation of treatment for
To address the constraints of current treatments, there is a growing interest in RNA-based therapies within the scientific community. In comparison to conventional drugs targeting proteins or DNA, RNA-based therapies show promise owing to their distinctive physicochemical and physiological properties[9-12]. ASOs, siRNAs, and microRNAs are RNA molecules that specifically interact with mRNA and non-coding RNAs (ncRNAs) through Watson-Crick base pairing. This interaction allows them to directly target and regulate the activity of these RNA molecules[12]. Theoretically, RNA can selectively target any specific gene by identifying the appropriate nucleotide sequence on the target RNA. RNA interference (RNAi) serves as a cellular mechanism for the downregulation of gene expression in response to genetic abnormalities and infections[13]. The current consensus regards it as a universally applicable and straightforward biological tool for conducting gene silencing studies. This tool holds promise for developing therapeutic approaches aimed at treating various diseases, including cancer[14,15]. In vitro transcribed (IVT) mRNAs have the potential to be employed in protein replacement therapy or immunization. Upon entering the cytoplasm, they can achieve these therapeutic goals without inducing irreversible genomic alterations or posing genetic risks, in contrast to DNA-based therapies[16]. Furthermore, the clustered regularly interspaced short palindromic repeats (CRISPR)-based genome editing technique enables the precise modification of target RNA sequences for the targeted treatment of specific diseases[17]. RNA aptamers possess the ability to impede protein activity, akin to the functionality exhibited by small molecule inhibitors and antibodies[13]. Consequently, RNA-based treatments are thought to be the most appealing therapeutic targets because they can increase the number of druggable targets[18]. Currently, RNA-based therapies in combination with chemotherapy and immunotherapy have become a hot research topic for developing therapies for different types of cancers. This review provides an overview of the classification and use of RNA-based therapies, as well as the development trends and practical directions of RNA-based therapies for the treatment of HCC. It also covers the advancements made in clinical studies involving RNA as drugs combined with immunotherapy and chemotherapy.
RNA INTERFERENCE
Andrew Fire and Craig C. Mello’s 1998 paper introduced the concept that the suppression of homologous gene expression through positive-sense RNA was initiated by the presence of minute amounts of dsRNA in RNA obtained through in vitro transcription. This phenomenon, termed RNA interference (RNAi), brought about a revolutionary shift in the domain of gene silencing[19]. RNAi-based therapeutic strategies involve the silencing of genes by harnessing the inherent cellular machinery of the targeted cells. The RNA-induced silencing complex (RISC), which binds to single-stranded 20-30 nt RNAs in the miRNA and siRNA pathways, is the minimal effector of RNAi. Through pre- or post-initiation translational repression and mRNA complementary degradation, RISC can be activated to produce gene silencing effects through the catalytic inactivation of Argonaute's or partial complementation with the target genes[13]. After cutting, the newly produced siRNA fragment can be utilized as a primer to create a new dsRNA using mRNA as a template. This dsRNA can then bind with Dicer to form an RISC, which will mediate the subsequent round of homologous mRNA degradation. This allows for the degradation of a large number of homologous mRNAs, which amplifies the cascade amplification effect and amplifies the inhibition effect on gene expression [Figure 1][20]. Post-transcriptional gene silencing by RNAi has a high degree of sequence specificity, closely follows the base complementary pairing principle, attaches to the target mRNA, and only destroys the homologous mRNA, effectively silencing the target gene alone. Moreover, the RNA interference effect can be stably inherited to the next generation[19].
Figure 1. Types and mechanisms of RNA-based therapies (Created with BioRender.com). (A) RNA interference. Long double-stranded RNA and precursor microRNA are processed by Dicer into siRNAs and mature miRNAs, which are loaded into RISCs for RNA targeting, degradation, or translational repression; (B) mRNA vaccines. mRNAs are delivered to dendritic cells by lipid nanoparticles, released into the cytoplasm, and translated into antigenic proteins by ribosomes. Some antigenic proteins are degraded into small peptides by the proteasome and are presented to the surface of CD8+ T cells by MHC I. CD8+ cytotoxic T cell-mediated immunity kills infected cells by secretion of perforin or granzyme. Other antigenic proteins are degraded in lysosomes and displayed on the surface of CD4+T cells by MHC II. B cell-mediated humoral immunity uses antibodies to neutralize pathogens; (C) Antisense oligonucleotides. ASO can regulate the expression of target genes by two mechanisms: (1) an occupancy-only mechanism and (2) an RNA degradation mechanism.
siRNA is a class of double-stranded non-coding RNA molecules, 20-27 base pairs in length. These molecules are generated when RNase III endonuclease Dicer cleaves long dsRNA precursors into smaller pieces. siRNAs are incorporated into the structure of a complex consisting of RISC and AGO2[20]. The antisense strand of the siRNA binds to complementary sequences on specific target mRNAs, triggering the cleavage pathway, and the AGO2 ribonucleic acid endonuclease cleaves the target mRNAs into small fragments, blocking their translation into functional proteins [Figure 1][20-22].
miRNAs are endogenous small non-coding RNAs with a length of approximately 19-25 nucleotides. Primary miRNAs (pri-miRNAs) have a stem-loop or short hairpin structure of ~33 nucleotides and are processed into precursor miRNAs (pre-miRNAs) of nearly 70 nucleotides[23-26]. Pre-miRNAs translocate to the cytoplasm by Exportin5. Activated by Dicer, it is processed into a mature miRNA that is loaded into the RISC-AGO2 complex [Figure 1]. There, the antisense strand binds complementarily to the target mRNA’s
mRNA AND mRNA VACCINES
In 1978, scientists used lipid-based membrane structures known as liposomes to deliver mRNA into both mouse organisms and human cells, causing the expression of proteins[28]. mRNAs are translated into the cytoplasm instead of the nucleus, which eliminates the possibility of them becoming integrated into the host genome, in contrast to conventional vaccines[16]. Moreover, it is possible to produce RNA vaccines quickly, cheaply, and uniformly[10]. These mRNAs, which are produced outside of the normal cellular environment, encode tumor antigens, cytokines, tumor suppressors, chimeric antigen receptors, or genome editing proteins. They are essential to a number of therapeutic applications, such as cancer vaccines, immunotherapy, tumor suppression, engineered T-cell therapies, and gene therapy. Similar to naturally occurring mRNAs in eukaryotic cells, conventional IVT mRNAs consist of essential elements such as a
Tumor-associated antigens (TAA) and cancer neoantigens are the primary targets of cancer vaccines. TAA refers to atypically expressed proteins in tumors relative to normal tissues, such as overexpression, distinct subcellular localization, and expression that is specific to the tumor (typically isolated at immune-privileged sites or expressed at specific stages of differentiation)[29]. Carrier-delivered mRNA enters dendritic cells through endocytosis, forming endosomes. Within the acidic environment of endosomes, the heads of ionizable lipids are protonated to the cationic state, which generates ion pairs with anionic phospholipids naturally occurring in the endosomal membranes. These ion pairs are smaller than the combined areas of the individual ionizable lipid heads before membrane fusion, thus facilitating membrane fusion and disruption and enabling the mRNA to escape from the endosome and enter the cytoplasm. Once in the cytoplasm, the mRNA is translated by ribosomes into antigenic proteins[41]. After that, the proteasome breaks it down into smaller pieces that are exposed to the cell surface and interact with MHC class I molecules on dendritic cells to activate CD8+T cells and cause cancer cells to undergo apoptosis [Figure 1][42]. As an alternative, mRNA translation produces antigens that are released into the extracellular space, broken down into peptide fragments in the endosome following absorption by
Cytokines play a key role in regulating immune system function, with IFN-α and IL-2 being representative immune agents for tumors. However, there are many problems with previous agent approaches: short
The application of mRNA technology in the design of specific T cell receptor (TCR) or chimeric antigen receptor (CAR) retargeting T cells has become an important direction in the field of tumor immunotherapy in recent years. mRNAs can encode different TCR or CAR molecules, providing a variety of design choices for targeting different antigens. mRNAs can mediate the rapid and controllable expression of genes, avoiding the risks of insertional mutagenesis and genomic instability, and improving therapeutic safety. The rapid and controlled expression of mRNA-mediated genes avoids the risk of insertion mutations and genomic instability, improving the safety of the treatment, and also enables the design of personalized TCR or CAR T cells based on the patient's specific tumor antigens, improving the specificity and efficacy of the treatment. mRNAs can encode for specific TCR alpha and TCR beta chains, and can be introduced into
ANTISENSE OLIGONUCLEOTIDE
Antisense oligonucleotides are single-stranded nucleic acid synthesis polymers. ASOs can be divided into two subcategories according to their mode of action:
One mechanism by which ASOs downregulate gene expression involves the promotion of RNA cleavage and degradation through interactions with endogenous enzymes such as ribonuclease H1 (RNase H1) or argonaute2. The ASO binds to target RNAs at its binding site, facilitating the binding of endogenous enzymes that subsequently cleave the target RNAs. This process leads to the degradation of the target RNAs, and its effectiveness relies on the involvement of specific enzymes in the regulatory pathway[13,53]. RNase H1 represents a highly discerning nucleic acid endonuclease with a specific activity targeting the RNA component within RNA-DNA heteroduplexes[24,54]. RNase H1 is present in the nucleus, mitochondria, and cytoplasm of mammalian cells[54,55]. It participates in DNA repair, resolves R-loops, removes pre-mRNAs connected to chromatin, aids in transcriptional termination, maintains genome integrity, and gets rid of RNA linked to Okazaki fragments, among other diverse roles it plays in the genome[56-60]. The primary benefit of employing RNase H1-based ASO lies in its facilitation of targeting nuclear transcripts, such as pre-mRNAs and long-stranded non-coding RNAs[24,61].
The other is occupation-only mediated regulation, often termed steric block[62]. High-affinity ASOs are used in the occupation-only mediated mechanism to bind to target RNAs without directly degrading them[53,63]. ASOs are designed to prevent the establishment of nucleotide compositions within RNA-DNA duplexes. This strategy aims to minimize the risk of generating substrates for RNaseH1 or Ago2, thereby reducing the likelihood of undesired cleavage of target RNA [Figure 1][62]. Spatially blocking ASOs bind to specific sequences of target nucleotides and act by regulating RNA translation, processing, splicing, RNA-protein interactions, and the target RNA interactome[64]. The most common tactic is to modify RNA splicing[65]. Splicing-switching ASOs have the capability to alter splicing patterns by specifically targeting splicing regulatory cis-elements. Through the recruitment of trans-splicing factors, cis-acting elements are essential in either activating or inhibiting adjacent splice sites. These cis-elements include silencers and enhancers for splicing. Stimulated splicing factors have trouble binding to their corresponding enhancer binding sites when ASOs form base pairs with splicing enhancer sequences. Exon skips are caused by this inhibition of binding, which makes splicing more difficult. On the other hand, ASOs that target the splicing silencing sequence element prevent repressive splicing factors from binding. As a result, exon inclusion56 is eventually caused by the silencing element's negative regulatory effect on splicing activation at the splice site [Figure 1][62].
DELIVERY VEHICLE
Successful RNA therapy depends on the ability to efficiently transport RNA to the cytoplasm through extracellular and intracellular barriers. RNA drugs must evade the large number of RNases present in blood and body fluids[66]. Moreover, RNA medications need to escape from endosomes and enter the cytoplasm in a nontoxic way by crossing the cell membrane and extracellular matrix through receptor-mediated endocytosis[67]. The limitations of RNA drug delivery have been overcome by delivery carriers, and advances in materials science and nanotechnology have also significantly improved drug usage and safety. Carriers can not only encapsulate the drug inside to prevent degradation but also cross the cell membrane to deliver RNA drugs into the cell. In addition, optimization of the carrier itself can play the role of specific targeting, reduce drug off-targeting, and improve the efficacy of RNA drugs while reducing toxic side effects[49,68-70].
Originally designed for the delivery of siRNA, lipid nanoparticles (LNPs) have recently been applied to deliver mRNA and have become the most clinically translatable non-viral delivery vector[29]. Cationic or ionizable lipids have the ability to form complexes with mRNA. Cholesterol enhances stability and facilitates membrane fusion, while polyethylene glycol (PEG)-lipids play a role in controlling size and stability to prevent aggregation. Phospholipids are involved in mediating mRNA encapsulation. By adjusting the ratios of these components, LNPs can be tailored to possess RNA delivery capabilities and target specific organs or cells[44,71-73]. Receptor-mediated endocytosis based on apolipoprotein E (ApoE) or albumin and nonspecific microcellular efflux are the two main mechanisms responsible for the renewal of RNA-loaded LNPs[74,75]. Moreover, LNPs employed for nucleic acid delivery must exhibit metabolic compatibility and swift clearance, thereby mitigating the risk of systemic toxicity attributed to the carrier. This facilitates consistent reproducibility in administration.
Polymeric nanoparticles, serving as non-viral carriers for gene delivery, exhibit promising potential as nucleic acid vectors. Key structural characteristics, such as molecular weight, surface charge, hydrophobicity, and chain compliance, make it easy to determine their fate. The goal of this strategy is to improve gene delivery efficiency[76-79]. Chitosan and natural chitosan nanoparticles, such as exosomes, have been employed as innovative carriers for delivering siRNA. This approach effectively silences crucial target genes in vivo, resulting in substantial antitumor activity[80,81]. The linear or branched polycations in the polyethyleneimine (PEI) polymer family can form nanoscale complexes with siRNAs or miRNAs. RNA molecules are shielded by this interaction, which also makes it easier for them to enter cells[82,83].
IMPLICATIONS OF RNA-BASED THERAPIES IN HCC
Through inhibition of the Notch3/Hes1/p27 cascade reaction, transcription factor C/EBP-α (CCAAT/enhancer-binding protein alpha) is a major regulator of liver and medullary function, as well as several oncogenic processes. It also suppresses tumor growth in vitro and in vivo and its expression is suppressed in several liver diseases, including HCC[84]. MTL-CEBPA, a liposomal nanoparticle-formulated small activating RNA (CEBPA-51) that upregulates hepatic C/EBP-α expression[85], is the first saRNA drug to enter clinical trials. It was tested in a phase I clinical trial in patients with advanced hepatocellular carcinoma in combination with sorafenib, and it demonstrated an acceptable safety profile in hepatocellular carcinoma as well as potential synergism with TKIs[86] [Table 1] [https://clinicaltrials.gov/study/NCT02716012]. A phase II study is recruiting patients with advanced HCC caused by hepatitis B or hepatitis C infection [Table 1] [https://clinicaltrials.gov/study/NCT04710641]. Additionally, a Phase I clinical trial combining MTL-CEBPA with bevacizumab and atilizumab, the standard of care, is being conducted to treat advanced hepatocellular carcinoma [Table 1] [https://clinicaltrials.gov/study/NCT05097911].
RNA-based therapeutics in clinical trials in liver cancer
RNA class | Target gene | Drug | delivery method | Administration route | Sponsor | Phases | References |
siRNA | MYC | DCR-MYC | LNP | I.V. | Dicerna Pharmaceuticals, Inc., a Novo Nordisk company | Ib/II | [https://clinicaltrials.gov/study/NCT02314052] |
siRNA | PLK1 | TKM-080301 | LNP | HAI | National Cancer Institute | I | [https://clinicaltrials.gov/study/NCT014337007] |
siRNA | KSP, VEGF | ALN-VSP02 | SNALP | I.V. | Alnylam Pharmaceuticals | I | [https://clinicaltrials.gov/study/NCT00882180] |
saRNA | CEBPA | MTL-CEBPA+Sorafenib | LNP | I.V. | Mina Alpha Limited | I | [https://clinicaltrials.gov/study/NCT02716012] |
saRNA | CEBPA | MTL-CEBPA+Sorafenib | LNP | I.V. | Mina Alpha Limited | II | [https://clinicaltrials.gov/study/NCT04710641] |
saRNA | CEBPA | MTL-CEBPA +Atezolizumab+Bevacizumab | LNP | I.V. | National University Hospital, Singapore | I | [https://clinicaltrials.gov/study/NCT05097911] |
microRNA | MRX34 | MRX34 | Liposomal | I.V. | Mirna Therapeutics, Inc. | I | [https://clinicaltrials.gov/study/NCT01829971] |
mRNA | CEA | CEA RNA-pulsed DC cancer vaccine | DC vaccine | I.V. | Duke University | I | [https://clinicaltrials.gov/study/NCT00004604] |
mRNA | Neoantigen | Neoantigen mRNA vaccine with or without PD-1/L1 | _ | _ | Jianming Xu | I | [https://clinicaltrials.gov/study/NCT05192460] |
mRNA | Neoantigen | Neoantigen mRNA vaccine + Stintilimab I | _ | _ | Shanghai Zhongshan Hospital | I | [https://clinicaltrials.gov/study/NCT05761717] |
mRNA | HBV | HBV mRNA Vaccine | _ | I.M | West China Hospital | I | [https://clinicaltrials.gov/study/NCT05738447] |
mRNA | Neoantigen | ABOR2014/IPM511 | _ | I.M | Peking Union Medical | I | [https://clinicaltrials.gov/study/NCT05981066] |
mRNA | MYC | OTX-2002+Tyrosine | LNP | I.V. | College Hospital Omega Therapeutics | I/II | [https://clinicaltrials.gov/study/NCT05497453] |
mRNA | HBV | kinase inhibitor+Checkpoint | _ | _ | Lion TCR Pte. Ltd. | I | [https://clinicaltrials.gov/study/NCT03634683] |
mRNA | HBV | Inhibitor LioCyx | _ | _ | Lion TCR Pte. Ltd. | I | [https://clinicaltrials.gov/study/NCT05195294] |
mRNA | HBV | LioCyx-M+ Lenvatinib | _ | _ | Lion TCR Pte. Ltd | I | [https://clinicaltrials.gov/study/NCT04745403] |
mRNA | HBV | mRNA HBV/TCR T-cells | _ | _ | Beijing 302 Hospital | I | [https://clinicaltrials.gov/study/NCT03899415] |
ASO | XIAP | TCR redirected T cells AEG35156+ Sorafenib | _ | I.V. | Aegera Therapeutics | I | [https://clinicaltrials.gov/study/NCT00882869] |
siRNA drugs efficiently silence the expression of target genes in a sequence-specific manner, providing an excellent opportunity to use this strategy in targeted cancer therapies. The MYC pathway is activated in 70% of hepatocellular carcinoma[87], which is involved in a variety of cellular processes that promote tumorigenesis, including cell cycle progression, apoptosis inhibition, cellular metabolism, and immune evasion[88]. MYC is therefore a very desirable therapeutic target. DCR-MYC is a lipid particle created by Dicerna Pharmaceuticals that binds to synthetic double-stranded RNA to target and silence the MYC oncogene, thereby preventing the spread of cancer. Patients with hepatocellular carcinoma who are either sorafenib-intolerant or sorafenib-refractory were evaluated for DCR-MYC in a Phase Ib/II clinical trial. Nevertheless, preliminary findings fell short of projections, leading to the termination of all DCR-MYC clinical trials, necessitating additional research [Table 1] [https://clinicaltrials.gov/study/NCT02314052]. The most well-known member of the serine-threonine kinase family, Plk1, is a Polo-like kinase (Plks) that controls cell mitosis and is overexpressed in many cancers[89]. miRNA targeting Plk1 is used to make
● Mis-expression or mutation of certain factors in the miRNA machinery can lead to altered processing, stability, or targeting of miRNAs, which can lead to serious diseases including cancer[27]. The miR-34 family suppresses tumorigenesis and slows the progression of tumors by taking part in the epithelial-mesenchymal transition (EMT) through the EMT-transcription factor p53 and various key signaling pathways[93]. The phase I clinical trial of MRX34, the first tumor-targeting miRNA drug based on a miR-34a mimic, ended prematurely due to five immune-related adverse events; however, the proof-of-concept for miRNA-based cancer therapy was provided by the dose-dependent regulation of the associated target genes[94] [Table 1] [https://clinicaltrials.gov/study/NCT01437007].
There are currently very few studies specifically for HCC, and most clinical trials for cancer vaccines are in phase I or phase II. The field of cancer vaccines is still in its developmental stage. Developing or bolstering anti-cancer immunity is the main goal of cancer vaccines. Tumor antigens elicit an immune response against cancer, and developing new tumor antigens presents several difficult obstacles. Globally, around 350 million individuals are chronically infected with HBV, and chronic HBV infection is a major contributor, constituting at least 50% of hepatocellular carcinoma cases worldwide[1]. Anti-HBV represents a promising focus in addressing hepatocellular carcinoma. A phase I clinical study is currently in progress, evaluating an mRNA vaccine for treating individuals with HBV-positive advanced hepatocellular carcinoma. This study aims to enroll patients who have experienced failure with second-line standard therapy or are ineligible for standard therapy. The trial involves a dose-escalation phase to determine an effective dosage for subsequent fixed-dose trials. The investigation focuses on assessing the safety, tolerability, and efficacy of applying mRNA immunotherapy technology in the clinical treatment of advanced hepatocellular carcinoma
Notably, mRNA-4157/V940 was created and produced based on a distinct mutational profile of the patient’s tumor DNA sequence. This mRNA encodes a single mRNA that can contain up to 34 neoantigens. In this Phase IIb clinical trial, postoperative patients with intermediate to advanced melanoma who were at high risk of recurrence received an adjuvant treatment consisting of the mRNA-4157/V940 vaccine plus pembrolizumab [Table 1] [https://clinicaltrials.gov/study/NCT03897881]. According to data, patients who received both the mRNA vaccine and pembrolizumab had a 44% lower risk of dying or having their disease return than those who only received pembrolizumab[96]. In February 2023, the mRNA vaccine, in combination with a PD-1 inhibitor, was cleared by the FDA as a new adjuvant therapy for the treatment of melanoma with a high risk of recurrence. This certification marks the first-ever mRNA vaccine against cancer worldwide, and it represents a significant advancement for mRNA vaccines in the anti-tumor arena. It is anticipated that this vaccine's phase III clinical trials will begin in accordance with other cancer types.
OTX-2002 is an mRNA therapy given to patients via LNP. It downregulates MYC expression pre-transcriptionally through epigenetic regulation while overcoming MYC auto-regulation. OTX-2002 is currently being evaluated as a single agent and in combination with a TKI or PD-(L)1 inhibitor in Phase 1/2 trials[97]. The primary endpoints of this trial for relapsed or refractory HCC and other solid tumor types known to be associated with the MYC oncogene are to determine the maximum tolerated dose of therapy, dose-limiting toxicity, incidence of therapeutic-emergent adverse events, overall efficacy rate, and duration of response [Table 1] [https://clinicaltrials.gov/study/NCT05497453].
The mRNA of the antigen-specific TCR-expressing antigen is introduced into T cells by electrotransformation and transiently expressed on T cells. Such a protocol is able to strike a good balance between safety and efficacy, ensuring that the infused specific TCR-T cells reach the target site through the blood circulation, triggering a controlled local inflammatory response that effectively kills tumor cells. Targeting HBV antigens expressed on HBV-HCC cells by transfecting autologous T cells (HBV-TCR T cells) with mRNA encoding HBV antigen-specific TCRs is currently the most widely used strategy [https://clinicaltrials.gov/study/NCT05738447][51,52,97], and several studies have entered clinical trials [Table 1].
DISCUSSION
Hepatocellular carcinoma, a highly aggressive form of cancer, has spurred ongoing exploration for novel therapeutic approaches due to the limitations of conventional treatments. RNA therapies have emerged as promising and innovative advancements in the field of hepatocellular carcinoma immunotherapy. The COVID-19 pandemic marked a significant milestone with the approval of two mRNA vaccines, namely mRNA-1273-P301 and BNT162b1. These vaccines were instrumental in initiating an immune response against SARS-CoV-2 and in spurring the mainstream drug development landscape to embrace RNA interference (RNAi) and mRNA-based therapies[98,99]. The results of RNA-based treatments have opened up new directions for the study of RNA components in the creation of cancer treatments. A more focused approach to therapy is provided by the development of personalized therapeutic strategies. By analyzing an individual's genomic profile, a customized mRNA vaccine is formulated to effectively stimulate the immune system for attacking hepatocellular carcinoma. The execution of clinical trials highlights the substantial potential of this approach in enhancing patient survival and reducing recurrence rates. The prospect of personalized mRNA vaccine therapy emerges as a crucial direction for future research. Given that
Furthermore, highly customized delivery platforms are made possible by optimizing targeting functionality and physical properties of nanoparticles. Increasing the effectiveness of RNA delivery will be largely dependent on the creation of innovative nanomaterials. By utilizing targeting molecules with specific affinity, RNA will be precisely directed to the surface of hepatocellular carcinoma cells to improve the precision of delivery. In addition, optimization of the physicochemical properties of the delivery system is also an important direction to ensure that RNA can be released stably and controllably in vivo.
RNA therapy also has some possible toxicity and safety issues. mRNA and siRNA, which may be recognized by the body's immune system as foreign substances, activate innate and adaptive immune responses, which may lead to inflammatory responses and cytokine storms, resulting in serious side effects[102,103]. Additionally, RNA molecular delivery systems may pose additional safety concerns. Lipid nanoparticles (LNPs) may be inherently toxic, possibly triggering an immune response or organ toxicity[72,73]. To reduce risk, researchers are developing safer delivery systems, optimizing RNA sequence design, and conducting exhaustive toxicology studies to ensure the safety and efficacy of RNA therapies in clinical applications.
RNA therapy has experienced notable advancements in the realm of cancer treatment, particularly due to its capacity to target diverse genetic materials within the body and the swift progress in drug development. It is plausible to anticipate an increased emphasis on research endeavors dedicated to the evolution of
DECLARATIONS
Acknowledgments
The figures are created with BioRender.com and quoted with permission from BioRender.
Authors’ contributions
Initiated the study and finalized the manuscript: Liu M
Reviewed the literature and wrote the manuscript: Xing L, Wang ZK, Li DM, Li J
Reviewed the literature and drew the pictures: Xing L
Review and revised the manuscript: Liu M
Read and approved the final manuscript: Xing L, Wang ZK, L DM, Li J, Liu M
Availability of data and materials
Not applicable.
Financial support and sponsorship
This research was supported by the National Natural Science Foundation of China (82122048).
Conflicts of interest
All authors declared that there are no conflicts of interest.
Ethical approval and consent to participate
Not applicable.
Consent for publication
Not applicable.
Copyright
© The Author(s) 2024.
REFERENCES
1. Sung H, Ferlay J, Siegel RL, et al. Global cancer statistics 2020: GLOBOCAN estimates of incidence and mortality worldwide for 36 cancers in 185 countries. CA Cancer J Clin 2021;71:209-49.
2. Llovet JM, Kelley RK, Villanueva A, et al. Hepatocellular carcinoma. Nat Rev Dis Primers 2021;7:6.
3. Kudo M, Finn RS, Qin S, et al. Lenvatinib versus sorafenib in first-line treatment of patients with unresectable hepatocellular carcinoma: a randomised phase 3 non-inferiority trial. Lancet 2018;391:1163-73.
4. Greten TF, Abou-Alfa GK, Cheng AL, et al. Society for immunotherapy of cancer (SITC) clinical practice guideline on immunotherapy for the treatment of hepatocellular carcinoma. J Immunother Cancer 2021;9:e002794.
5. Gallage S, García-Beccaria M, Szydlowska M, et al. The therapeutic landscape of hepatocellular carcinoma. Med 2021;2:505-52.
6. Lee MS, Ryoo BY, Hsu CH, et al. GO30140 investigators. Atezolizumab with or without bevacizumab in unresectable hepatocellular carcinoma (GO30140): an open-label, multicentre, phase 1b study. Lancet Oncol 2020;21:808-20.
7. Finn RS, Ryoo BY, Merle P, et al. KEYNOTE-240 investigators. Pembrolizumab As second-line therapy in patients with advanced hepatocellular carcinoma in KEYNOTE-240: a randomized, double-blind, phase III trial. J Clin Oncol 2020;38:193-202.
8. Finn RS, Qin S, Ikeda M, et al. IMbrave150 Investigators. Atezolizumab plus bevacizumab in unresectable hepatocellular carcinoma. N Engl J Med 2020;382:1894-905.
10. Kim YK. RNA therapy: rich history, various applications and unlimited future prospects. Exp Mol Med 2022;54:455-65.
12. Crooke ST, Witztum JL, Bennett CF, Baker BF. RNA-targeted therapeutics. Cell Metab 2018;27:714-39.
13. Wilson RC, Doudna JA. Molecular mechanisms of RNA interference. Annu Rev Biophys 2013;42:217-39.
14. Han X, Wang L, Li T, et al. Beyond blocking: engineering RNAi-mediated targeted immune checkpoint nanoblocker enables T-cell-independent cancer treatment. ACS Nano 2020;14:17524-34.
15. Zhang C, Zhao Y, Yang Y, et al. RNAi mediated silencing of Nanog expression suppresses the growth of human colorectal cancer stem cells. Biochem Biophys Res Commun 2021;534:254-60.
16. Pardi N, Hogan MJ, Porter FW, Weissman D. mRNA vaccines - a new era in vaccinology. Nat Rev Drug Discov 2018;17:261-79.
17. Rosenblum D, Gutkin A, Kedmi R, et al. CRISPR-Cas9 genome editing using targeted lipid nanoparticles for cancer therapy. Sci Adv 2020:6.
18. Adachi T, Nakamura Y. Aptamers: a review of their chemical properties and modifications for therapeutic application. Molecules 2019;24:4229.
19. Fire A, Xu S, Montgomery MK, Kostas SA, Driver SE, Mello CC. Potent and specific genetic interference by double-stranded RNA in Caenorhabditis elegans. Nature 1998;391:806-11.
20. Martinez J, Patkaniowska A, Urlaub H, Lührmann R, Tuschl T. Single-stranded antisense siRNAs guide target RNA cleavage in RNAi. Cell 2002;110:563-74.
21. Bernstein E, Caudy AA, Hammond SM, Hannon GJ. Role for a bidentate ribonuclease in the initiation step of RNA interference. Nature 2001;409:363-6.
22. Meister G, Tuschl T. Mechanisms of gene silencing by double-stranded RNA. Nature 2004;431:343-9.
23. Robb T, Reid G, Blenkiron C. Exploiting microRNAs as cancer therapeutics. Target Oncol 2017;12:163-78.
24. Roberts TC, Langer R, Wood MJA. Advances in oligonucleotide drug delivery. Nat Rev Drug Discov 2020;19:673-94.
25. Oura K, Morishita A, Masaki T. Molecular and functional roles of microRNAs in the progression of hepatocellular carcinoma-A review. Int J Mol Sci 2020;21:8362.
26. To KKW, Fong W, Tong CWS, Wu M, Yan W, Cho WCS. Advances in the discovery of microRNA-based anticancer therapeutics: latest tools and developments. Expert Opin Drug Discov 2020;15:63-83.
27. Barata P, Sood AK, Hong DS. RNA-targeted therapeutics in cancer clinical trials: Current status and future directions. Cancer Treat Rev 2016;50:35-47.
30. Mockey M, Gonçalves C, Dupuy FP, Lemoine FM, Pichon C, Midoux P. mRNA transfection of dendritic cells: synergistic effect of ARCA mRNA capping with poly(A) chains in cis and in trans for a high protein expression level. Biochem Biophys Res Commun 2006;340:1062-8.
31. Muttach F, Muthmann N, Rentmeister A. Synthetic mRNA capping. Beilstein J Org Chem 2017;13:2819-32.
32. Shanmugasundaram M, Senthilvelan A, Kore AR. Recent advances in modified cap analogs: synthesis, biochemical properties, and mRNA based vaccines. Chem Rec 2022;22:e202200005.
33. Orlandini von Niessen AG, Poleganov MA, Rechner C, et al. Improving mRNA-based therapeutic gene delivery by expression-augmenting 3' UTRs identified by cellular library screening. Mol Ther 2019;27:824-36.
34. Jia L, Mao Y, Ji Q, Dersh D, Yewdell JW, Qian SB. Decoding mRNA translatability and stability from the 5' UTR. Nat Struct Mol Biol 2020;27:814-21.
35. Alexaki A, Hettiarachchi GK, Athey JC, et al. Effects of codon optimization on coagulation factor IX translation and structure: Implications for protein and gene therapies. Sci Rep 2019;9:15449.
36. Karikó K, Weissman D. Naturally occurring nucleoside modifications suppress the immunostimulatory activity of RNA: implication for therapeutic RNA development. Curr Opin Drug Discov Devel 2007;10:523-32.
37. Holtkamp S, Kreiter S, Selmi A, et al. Modification of antigen-encoding RNA increases stability, translational efficacy, and T-cell stimulatory capacity of dendritic cells. Blood 2006;108:4009-17.
38. Willis E, Pardi N, Parkhouse K, et al. Nucleoside-modified mRNA vaccination partially overcomes maternal antibody inhibition of de novo immune responses in mice. Sci Transl Med 2020:12.
39. Sharifnia Z, Bandehpour M, Kazemi B, Zarghami N. Design and development of modified mRNA encoding core antigen of hepatitis C virus: a possible application in vaccine production. Iran Biomed J 2019;23:57-67.
40. Karikó K, Muramatsu H, Welsh FA, et al. Incorporation of pseudouridine into mRNA yields superior nonimmunogenic vector with increased translational capacity and biological stability. Mol Ther 2008;16:1833-40.
41. Oberli MA, Reichmuth AM, Dorkin JR, et al. Lipid nanoparticle assisted mRNA delivery for potent cancer immunotherapy. Nano Lett 2017;17:1326-35.
42. Chen J, Ye Z, Huang C, et al. Lipid nanoparticle-mediated lymph node-targeting delivery of mRNA cancer vaccine elicits robust CD8+ T cell response. Proc Natl Acad Sci U S A 2022;119:e2207841119.
43. Kreiter S, Vormehr M, van de Roemer N, et al. Mutant MHC class II epitopes drive therapeutic immune responses to cancer. Nature 2015;520:692-6.
44. Kranz LM, Diken M, Haas H, et al. Systemic RNA delivery to dendritic cells exploits antiviral defence for cancer immunotherapy. Nature 2016;534:396-401.
45. Linares-Fernández S, Lacroix C, Exposito JY, Verrier B. Tailoring mRNA vaccine to balance innate/adaptive immune response. Trends Mol Med 2020;26:311-23.
46. Esprit A, de Mey W, Bahadur Shahi R, Thielemans K, Franceschini L, Breckpot K. Neo-antigen mRNA vaccines. Vaccines 2020;8:776.
47. Peng M, Mo Y, Wang Y, et al. Neoantigen vaccine: an emerging tumor immunotherapy. Mol Cancer 2019;18:128.
48. Keskin DB, Anandappa AJ, Sun J, et al. Neoantigen vaccine generates intratumoral T cell responses in phase Ib glioblastoma trial. Nature 2019;565:234-9.
49. Lang F, Schrörs B, Löwer M, Türeci Ö, Sahin U. Identification of neoantigens for individualized therapeutic cancer vaccines. Nat Rev Drug Discov 2022;21:261-82.
50. Liu JQ, Zhang C, Zhang X, et al. Intratumoral delivery of IL-12 and IL-27 mRNA using lipid nanoparticles for cancer immunotherapy. J Control Release 2022;345:306-13.
51. Tan AT, Meng F, Jin J, et al. Immunological alterations after immunotherapy with short lived HBV-TCR T cells associates with long-term treatment response in HBV-HCC. Hepatol Commun 2022;6:841-54.
52. Rajan T, Gugliandolo A, Bramanti P, Mazzon E. In vitro-transcribed mRNA chimeric antigen receptor T cell (IVT mRNA CAR T) therapy in hematologic and solid tumor management: a preclinical update. Int J Mol Sci 2020;21:6514.
54. Wu H, Lima WF, Zhang H, Fan A, Sun H, Crooke ST. Determination of the role of the human RNase H1 in the pharmacology of DNA-like antisense drugs. J Biol Chem 2004;279:17181-9.
55. Liang XH, Sun H, Nichols JG, Crooke ST. RNase H1-dependent antisense oligonucleotides are robustly active in directing rna cleavage in both the cytoplasm and the nucleus. Mol Ther 2017;25:2075-92.
56. Ruhanen H, Ushakov K, Yasukawa T. Involvement of DNA ligase III and ribonuclease H1 in mitochondrial DNA replication in cultured human cells. Biochim Biophys Acta 2011;1813:2000-7.
57. Lima WF, Murray HM, Damle SS, et al. Viable RNaseH1 knockout mice show RNaseH1 is essential for R loop processing, mitochondrial and liver function. Nucleic Acids Res 2016;44:5299-312.
59. Cerritelli SM, Crouch RJ. RNases H: multiple roles in maintaining genome integrity. DNA Repair 2019;84:102742.
60. Lai F, Damle SS, Ling KK, Rigo F. Directed RNase H cleavage of nascent transcripts causes transcription termination. Mol Cell 2020;77:1032-1043.e4.
61. Khan P, Siddiqui JA, Lakshmanan I, et al. RNA-based therapies: a cog in the wheel of lung cancer defense. Mol Cancer 2021;20:54.
62. Crooke ST, Baker BF, Crooke RM, Liang XH. Antisense technology: an overview and prospectus. Nat Rev Drug Discov 2021;20:427-53.
63. Ramasamy T, Ruttala HB, Munusamy S, Chakraborty N, Kim JO. Nano drug delivery systems for antisense oligonucleotides (ASO) therapeutics. J Control Release 2022;352:861-78.
64. Desterro J, Bak-Gordon P, Carmo-Fonseca M. Targeting mRNA processing as an anticancer strategy. Nat Rev Drug Discov 2020;19:112-29.
65. Li D, Mastaglia FL, Fletcher S, Wilton SD. Precision medicine through antisense oligonucleotide-mediated exon skipping. trends Pharmacol Sci 2018;39:982-94.
68. Chen X, Mangala LS, Rodriguez-Aguayo C, Kong X, Lopez-Berestein G, Sood AK. RNA interference-based therapy and its delivery systems. Cancer Metastasis Rev 2018;37:107-24.
69. Meng C, Chen Z, Li G, Welte T, Shen H. Nanoplatforms for mRNA therapeutics. Adv Ther 2021;4:2000099.
70. Byun MJ, Lim J, Kim SN, et al. Advances in nanoparticles for effective delivery of RNA therapeutics. Biochip J 2022;16:128-45.
71. Li Y, Fang H, Zhang T, et al. Lipid-mRNA nanoparticles landscape for cancer therapy. Front Bioeng Biotechnol 2022;10:1053197.
72. Witzigmann D, Kulkarni JA, Leung J, Chen S, Cullis PR, van der Meel R. Lipid nanoparticle technology for therapeutic gene regulation in the liver. Adv Drug Deliv Rev 2020;159:344-63.
73. Hou X, Zaks T, Langer R, Dong Y. Lipid nanoparticles for mRNA delivery. Nat Rev Mater 2021;6:1078-94.
74. Gilleron J, Querbes W, Zeigerer A, et al. Image-based analysis of lipid nanoparticle-mediated siRNA delivery, intracellular trafficking and endosomal escape. Nat Biotechnol 2013;31:638-46.
75. Sato Y, Kinami Y, Hashiba K, Harashima H. Different kinetics for the hepatic uptake of lipid nanoparticles between the apolipoprotein E/low density lipoprotein receptor and the N-acetyl-d-galactosamine/asialoglycoprotein receptor pathway. J Control Release 2020;322:217-26.
76. Kamaly N, Xiao Z, Valencia PM, Radovic-Moreno AF, Farokhzad OC. Targeted polymeric therapeutic nanoparticles: design, development and clinical translation. Chem Soc Rev 2012;41:2971-3010.
77. Rai R, Alwani S, Badea I. Polymeric nanoparticles in gene therapy: new avenues of design and optimization for delivery applications. Polymers 2019;11:745.
79. Ke L, Cai P, Wu Y, Chen X. Polymeric nonviral gene delivery systems for cancer immunotherapy. Adv Ther 2020;3:1900213.
80. Aqil F, Munagala R, Jeyabalan J, et al. Milk exosomes - natural nanoparticles for siRNA delivery. Cancer Lett 2019;449:186-95.
81. Liang Y, Wang Y, Wang L, et al. Self-crosslinkable chitosan-hyaluronic acid dialdehyde nanoparticles for CD44-targeted siRNA delivery to treat bladder cancer. Bioact Mater 2021;6:433-46.
82. Höbel S, Appeldoorn CC, Gaillard PJ, Aigner A. Targeted CRM197-PEG-PEI/siRNA complexes for therapeutic RNAi in glioblastoma. Pharmaceuticals 2011;4:1591-606.
83. Pandey AP, Sawant KK. Polyethylenimine: a versatile, multifunctional non-viral vector for nucleic acid delivery. Mater Sci Eng C Mater Biol Appl 2016;68:904-18.
84. Shi YC, Zhao H, Yin C, et al. C/EBPα inhibits hepatocellular carcinoma by reducing Notch3/Hes1/p27 cascades. Dig Liver Dis 2013;45:844-51.
85. Voutila J, Reebye V, Roberts TC, et al. Development and mechanism of small activating RNA targeting CEBPA, a novel therapeutic in clinical trials for liver cancer. Mol Ther 2017;25:2705-14.
86. Sarker D, Plummer R, Meyer T, et al. MTL-CEBPA, a small activating RNA therapeutic upregulating C/EBP-α, in patients with advanced liver cancer: a first-in-human, multicenter, open-label, phase I trial. Clin Cancer Res 2020;26:3936-46.
87. Schaub FX, Dhankani V, Berger AC, et al. Cancer Genome Atlas Network. Pan-cancer alterations of the MYC oncogene and its proximal network across the cancer genome atlas. Cell Syst 2018;6:282-300.e2.
88. Liu F, Liao Z, Zhang Z. MYC in liver cancer: mechanisms and targeted therapy opportunities. Oncogene 2023;42:3303-18.
89. Liu X. Targeting polo-like kinases: a promising therapeutic approach for cancer treatment. Transl Oncol 2015;8:185-95.
90. Semple SC, Judge AD, Robbins M, et al. Abstract 2829: preclinical characterization of TKM-080301, a lipid nanoparticle formulation of a small interfering RNA directed against polo-like kinase 1. Cancer Res 2011;71:2829-2829.
91. El Dika I, Lim HY, Yong WP, et al. An open-label, multicenter, Phase I, dose escalation study with phase ii expansion cohort to determine the safety, pharmacokinetics, and preliminary antitumor activity of intravenous TKM-080301 in subjects with advanced hepatocellular carcinoma. Oncologist 2019;24:747-e218.
92. Cervantes A, Alsina M, Tabernero J, et al. Phase I dose-escalation study of ALN-VSP02, a novel RNAi therapeutic for solid tumors with liver involvement. JCO 2011;29:3025.
93. Zhang L, Liao Y, Tang L. MicroRNA-34 family: a potential tumor suppressor and therapeutic candidate in cancer. J Exp Clin Cancer Res 2019;38:53.
94. Hong DS, Kang YK, Borad M, et al. Phase 1 study of MRX34, a liposomal miR-34a mimic, in patients with advanced solid tumours. Br J Cancer 2020;122:1630-7.
95. Hu Z, Leet DE, Allesøe RL, et al. Personal neoantigen vaccines induce persistent memory T cell responses and epitope spreading in patients with melanoma. Nat Med 2021;27:515-25.
96. Weber JS, Carlino MS, Khattak A, et al. Individualised neoantigen therapy mRNA-4157 (V940) plus pembrolizumab versus pembrolizumab monotherapy in resected melanoma (KEYNOTE-942): a randomised, phase 2b study. Lancet 2024;403:632-44.
97. Rodriguez-rivera II, Wu T, Ciotti R, et al. A phase 1/2 open-label study to evaluate the safety, tolerability, pharmacokinetics, pharmacodynamics, and preliminary antitumor activity of OTX-2002 as a single agent and in combination with standard of care in patients with hepatocellular carcinoma and other solid tumor types known for association with the MYC oncogene (MYCHELANGELO I). JCO 2023;41:TPS627-TPS627.
98. Oliver SE, Gargano JW, Marin M, et al. The advisory committee on immunization practices' interim recommendation for use of Pfizer-BioNTech COVID-19 vaccine - United States, December 2020. MMWR Morb Mortal Wkly Rep 2020;69:1922-4.
99. Ledford H. Moderna COVID vaccine becomes second to get US authorization. Nature 2020:Online ahead of print.
100. Childs-Disney JL, Yang X, Gibaut QMR, Tong Y, Batey RT, Disney MD. Targeting RNA structures with small molecules. Nat Rev Drug Discov 2022;21:736-62.
101. Falese JP, Donlic A, Hargrove AE. Targeting RNA with small molecules: from fundamental principles towards the clinic. Chem Soc Rev 2021;50:2224-43.
102. Winkle M, El-Daly SM, Fabbri M, Calin GA. Noncoding RNA therapeutics - challenges and potential solutions. Nat Rev Drug Discov 2021;20:629-51.
Cite This Article

How to Cite
Download Citation
Export Citation File:
Type of Import
Tips on Downloading Citation
Citation Manager File Format
Type of Import
Direct Import: When the Direct Import option is selected (the default state), a dialogue box will give you the option to Save or Open the downloaded citation data. Choosing Open will either launch your citation manager or give you a choice of applications with which to use the metadata. The Save option saves the file locally for later use.
Indirect Import: When the Indirect Import option is selected, the metadata is displayed and may be copied and pasted as needed.
About This Article
Copyright
Data & Comments
Data
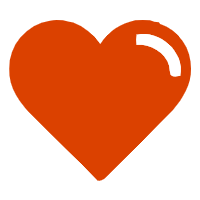
Comments
Comments must be written in English. Spam, offensive content, impersonation, and private information will not be permitted. If any comment is reported and identified as inappropriate content by OAE staff, the comment will be removed without notice. If you have any queries or need any help, please contact us at [email protected].