Role of diagnostic radiology in the management of intrahepatic cholangiocarcinoma
Abstract
Intrahepatic cholangiocarcinoma (ICC) is a rare and aggressive form of primary liver cancer that presents significant diagnostic and treatment challenges. In this review, we discuss the role of diagnostic radiology in the management of ICC, and future directions for research and clinical practice in the management of ICC.
Keywords
INTRODUCTION
Epidemiology and etiology of ICC
Intrahepatic cholangiocarcinoma (ICC) is a rare and aggressive malignancy originating from the bile duct epithelium within the liver. ICC accounts for 10%-15% of primary liver cancers and has a rising worldwide incidence[1]. The highest incidence of ICC has been observed in Southeast Asia, particularly in Thailand, where it is associated with liver fluke infections (Opisthorchis viverrini and Clonorchis sinensis)[2]. Other risk factors include primary sclerosing cholangitis, chronic viral hepatitis B and C, cirrhosis, exposure to thorotrast, and certain metabolic disorders[3,4].
Clinical presentation and prognosis
ICC often presents at an advanced stage due to its asymptomatic nature in the early stages of the disease. Patients may experience nonspecific symptoms such as abdominal pain, weight loss, fatigue, and jaundice. The presence of these symptoms is usually indicative of advanced disease, and patients may already have liver dysfunction or metastases at the time of diagnosis[5]. Therefore, the prognosis associated with ICC is generally poor, with a 5-year survival of approximately 10%-15%[6].
Importance of early and accurate diagnosis
Early and accurate diagnosis of ICC is essential to improve patient outcomes, as it allows for timely initiation of appropriate treatments. Early-stage tumors may be amenable to surgical resection, which offers the best chance of long-term survival. In contrast, advanced-stage tumors are usually not amenable to surgical resection; in turn, patients with advanced disease are often only eligible for non-curative treatment options such as chemotherapy, radiation therapy, or targeted therapies[5]. Precise diagnosis is also crucial to differentiate ICC from other hepatic malignancies, such as hepatocellular carcinoma (HCC), as the management strategies and prognoses for these tumors differ significantly[7]. This review aims to summarize the current literature regarding the role of diagnostic radiology in the management of ICC, and prospects for research and clinical practice in the management of ICC.
The role of radiology in the diagnosis
The diagnosis of ICC primarily relies on imaging studies and biopsy. Conventional imaging methods such as ultrasound, computed tomography (CT), magnetic resonance imaging (MRI), and Positron Emission Tomography (PET) [Figure 1] are commonly used to identify and characterize liver lesions. Distinguishing ICC from other primary liver cancers, such as HCC, combined hepatocellular cholangiocarcinoma (cHCC-CCA) [Figure 2], and secondary liver tumors (e.g., metastases from colorectal cancer) can be challenging, however, due to overlapping imaging features and nonspecific clinical presentation[8,9], precise differentiation is essential as management strategies and prognoses for these tumors differ significantly.
Figure 1. ICC in a 76-year-old female. Axial CT in the hepatic arterial phase (A) shows hypoattenuating and peripherally enhancing mass (arrow) involving nearly the entire left lobe of the liver, segment IV, and portions of segment VIII. Axial gadolinium-enhanced T1-weighted MR image in portal venous phase; (B) shows the tumor as a large multilobulated mass in the left hepatic lobe demonstrating continuous nodular peripheral enhancement with delayed fill-in. There is mild intrahepatic biliary dilatation peripheral to the mass in the left hepatic lobe. Axial maximum-intensity-projection image of 18F-FDG PET; (C) demonstrates a large left liver lobe lesion with peripheral hypermetabolic activity.
Figure 2. cHCC-ICC in a 64-year-old male with chronic hepatitis C. Axial CT in the portal venous phase (A) shows a hypoattenuating wedge-shaped subcapsular mass (arrow). Axial gadolinium-enhanced T1-weighted MR image in portal venous phase; (B) shows mild surface nodularity suggestive of cirrhosis. The mass demonstrates a hypointense wedge-shaped subcapsular lesion with peripheral arterial enhancement and persistent enhancement on delayed images. There is associated capsular retraction.
MRI generally outperforms CT and Ultrasound in the diagnosis of hepatic tumors. This is because MRI allows for the comparison of conventional T2-W and T1-W data with functional DWI and DCE-MRI data in a single protocol. Granata et al. evaluated MR features of patients with ICC and compared the radiographic features with control groups, including colorectal hepatic metastases (group A), peribiliary metastases (group B), HCC (group C), cHCC-CCA (group D), and hemangioma (group E). Of note, T1 and T2 W signal intensity (SI), restricted diffusion, (transitional phase) TP, and (hepatobiliary phase) HP appearance was able to differentiate ICCs from mimickers; the arterial phase (AP) appearance differentiated the study group from the control groups C and D. Portal phase and washout appearance distinguished ICC tumors from control groups A, C, and D[10].
Conventional MRI sequence characteristics can distinguish HCC and cHCC-CCA. The radiologist should suspect cHCC-CCA if the lesion has satellite nodules, a hyperintense signal on T2-W, restricted diffusion, and no capsule in a nodule with peripheral and increasing contrast enhancement[11].
MRI with diffusion-weighted imaging (DWI) provides quantifiable functional parameters for measuring the mobility of water ions in the tissue microstructure. Viscosity, vascularity, and tissue cell density are all connected to water diffusion mobility. Numerous researchers have examined the role of DWI in ICC with a specific focus on how DWI affects the capability to distinguish between IMCC and HCC. A study by Park et al. employed the target appearance (TA) on the DWI to differentiate small ICC from HCC[12,13], and numerous studies have corroborated this finding[13,14].
Recently, contrast-enhanced imaging techniques have been developed, which have markedly improved the visualization of tumor vascularity, aiding in the characterization and differentiation of ICC from other hepatic malignancies. Multi-phase contrast-enhanced CT involves acquiring images at different time points after the administration of a contrast agent, highlighting the arterial, portal venous, and delayed phases of tumor enhancement. Similarly, dynamic contrast-enhanced MRI (DCE-MRI) involves the acquisition of images before and after the administration of a gadolinium-based contrast agent, providing information about the tumor’s vascular pattern and perfusion[15].
Gd-DTPA or its derivatives, such as Gd-DTPA-EOB (Eovist®), can be used for post-contrast MRI. Eovist, hepatocyte-specific contrast agents (HSCAs), are taken up by hepatocyte transporters and are discharged via the biliary tree. In the hepatobiliary phase, a lesion will maintain contrast only if it contains hepatocytes, which helps refine differential diagnosis. Eovist has been demonstrated to be more effective at identifying and characterizing hepatic lesions in patients with diffuse liver disease in which ICCs may show atypical enhancement patterns after gadolinium-based extracellular contrast agents. Hepatocyte-specific extracellular contrast agents like Eovist increase the liver's post-contrast signal intensity, making ICC more apparent and hypointense on both early and delayed phase sequences[16] [Figure 3]. This approach contrasts the lesion from the liver tissue, making tumor size and satellite lesions, which occur in 10%-20% of ICCs, easier to assess[16].
Figure 3. Intrahepatic cholangiocarcinoma (ICC) in a 57-year-old female. Axial gadolinium-enhanced T1-weighted MR image in hepatoarterial phase (A) and portal venous phase (B) shows a hypointense large mass (arrow) with capsular retraction and also progressive central enhancement on portal venous phase. Axial T2-weighted MR image; (C) shows the tumor has heterogeneous high signal intensity. Axial Evoist-enhanced T1-weighted MR image (D) shows rim-enhancing centrally necrotic mass. Axial diffusion-weighted MR image (E) demonstrates that the tumor restricts diffusion with the heterogeneous peripheral increased signal. ADC map (F) demonstrates a low ADC value in the rim area and a heterogenous higher ADC value in the center of the lesion.
In mass-forming cholangiocarcinoma, Eovist causes the “gadoxetic acid cloud” indication on hepatobiliary imaging. The fibrotic stroma may have extracellular contrast accumulation. Hepatobiliary phase imaging can help identify intrahepatic metastases, daughter nodules, and other abnormalities associated with poor mortality[17]. Moderately differentiated tumors often exhibit more relative enhancement in the hepatobiliary phase than poorly differentiated cancers. The hepatobiliary phase can also demonstrate a higher relative tumor enhancement[18].
THE ROLE OF DIAGNOSTIC RADIOLOGY IN THE STAGING AND PROGNOSIS
Resectable ICC
Numerous staging methods estimate the prognosis of patients with resectable ICC[19-22]. The most used technique is the American Joint Committee on Cancer staging system (AJCC) staging system, a tumor, nodes, and metastases (TNM) staging system[23]. Due to the restricted number of tumor-related characteristics and the inability to customize prognostication for unresectable tumors, this staging approach has drawn criticism[21]. Numerous nomograms have also been created to improve outcome prediction, some of which have shown superior accuracy to the AJCC staging system[19,22,24,25]. These prognostic models consider various factors, such as certain biomarkers, anatomical-related imaging features, and specific pathological parameters. However, none of them contain functional information about a patient's tumor. Furthermore, similar to the AJCC staging system, they are all based on tissue acquisition and may not be applied in unresectable ICC patients.
The role of radiomics or radiogenomics in ICC prognosis has been assessed in a number of research studies[26-38], and the evaluation of recurrence following surgical resection has been the main focus[36,39-42]. Yang et al. evaluated MRI-extracted quantitative features for evaluation of overall survival in ICC patients and developed a combined model integrating radiomics features with clinical factors that increased the precision of prediction compared with TNM stage alone[43].
Unresectable ICC
Due to frequent delays in diagnosis, about 70% of patients with ICCs present with advanced-stage tumors and are considered unresectable[44]. In addition, many patients have comorbid conditions that prevent them from being candidates for resection; these patients may be eligible for loco-regional therapy. In this regard, there are challenges for tumor staging and monitoring treatment response.
Jiang et al. provided the first nonsurgical-pathological prediction model (Fudan scoring system) for ICC, which required clinical data obtained before surgery through imaging and biochemical blood tests and could be used as a prediction model for unresectable ICC. However, the author mentioned that this model’s subjective assessment of tumor margins is a significant limitation that could lower prediction accuracy, so a more objective parameter is required. Ignorance of lymph node (LN) status is another limitation pointed out by the author, which has been strongly associated with prognosis and poor prediction accuracy[45].
According to prior research, factors related to postoperative survival in patients with ICC include microvascular invasion (MVI), tumor size, tumor grade, multiple tumors, and lymph node involvement[46].
MVI, an important predictor of ICC outcome, is characterized by the presence of a tumor in a portal vein, hepatic vein, or a large capsular vessel[47]. To date, MVI can only be identified through postoperative pathology and is only helpful as a reference factor for adjuvant chemotherapy following ICC surgery. Consequently, it is essential to predict MVI before surgery. According to the study by Lee et al., the level of diffusion restriction in ICC was a standalone predictive parameter for the outcome[48]. The ADC value of the ICC has been demonstrated by Zhou et al. to be significantly linked with microvascular invasion (MVI)[49]. Ma et al. suggested some quantitative and qualitative MRI features from preoperative DCE-MR images to evaluate MVI before surgery[46]. Their finding showed that MVI of ICC is correlated with six MR characteristics, including two quantitative parameters (arterial phase edge enhancement ratio and maximum tumor diameter) and four qualitative features (tumor morphology, intrahepatic duct dilatation, arterial phase enhancement pattern, and visible hepatic artery penetration sign).
Another critical predictor of outcome in ICC patients is LNM[50,51]. In light of the fact that neoadjuvant therapy can increase the survival of ICC with LNM[52] and LN dissection did not improve chances of survival in ICC without LNMs, it is crucial to identify LNM by imaging before surgery[53].
However, there are still concerns about preoperative imaging to detect LNM accurately, particularly for periductal infiltrating ICC subtypes[54,55].
The size, shape, and necrosis are key imaging characteristics in the detection of LNM[56-58], and micrometastases are frequently missed in the imaging of normal-sized LNs[56,59,60]. Additionally, individuals with primary sclerosing cholangitis (PSC), where slightly enlarged lymph nodes are a frequent sign, can experience false-positive cases[61].
T1- and T2-weighted fat-suppressed images are very useful for the diagnosis of lymphadenopathy[62].
In this regard, also, Ji et al. proposed a nomogram to predict LNM using preoperative CA19-9 and eight LN CT-based quantitative features[64]. Another study by Meng and associates suggested a nomogram that used preoperative CA19-9 levels, the location of the original tumor, the size of the LNs on CT, and the growth trend of the tumor to forecast LNM in ICC[65].
While CT and MRI have poor sensitivity and specificity in detecting metastases in ICC, the ability of PET to measure metabolic activity makes it superior. In particular, PET can resolve spatial and temporal information when combined with CT. In a recent meta-analysis, PET/CT was reported to detect ICCs with 95% sensitivity and 93% specificity[66]. This technique may also improve nodal staging and identification of distant metastasis, altering the clinical treatment of 17%-30% of patients[67].
PET/CT may also aid in stratifying patients relative to prognosis. Specifically, SUVmax has been associated with overall survival and recurrence. Ma et al. reported that SUVmax values above 8 were associated with decreased survival. In contrast, tumors with low SUVmax had a more favorable prognosis, were more likely not to be associated with metastatic disease, and had a more favorable prognosis following surgical resection[68].
PET/MRI is another imaging modality increasingly used for cancer detection and staging. Kirchner et al. reported that PET/MRI with the liver-specific contrast agent gadobenate dimeglumine assisted in ICC detection and diagnosis. PET/MRI classified tumors as malignant or benign with 98% and 100% accuracy[69]. Nonetheless, ICC-specific PET/MRI investigations are needed to determine its role in diagnosis and staging.
More recently, novel ligands have been developed alongside the PET technique. Of note, in contrast to cancer-associated fibroblasts, normal human tissues have very little fibroblast activation protein (FAP) expression[70]. PET can image this pathophysiological process using the new ligand “fibroblast activation protein inhibitor (FAPI)” attached to fluorine-18 or gallium-68. FAPI PET is safe and has outperformed FDG in early clinical trials[71]. In a recent study, Veldhuijzen van Zanten et al. compared FAPI PET to FDG PET, CECT, and MRI to stage pancreatic, gastric, and cholangiocarcinoma. While initial studies have suggested that FAPI PET may outperform other conventional diagnostic methods, few investigations have been done, and more data are needed. FAPI has theranostic potential since its ligand can bind to diagnostic or therapeutic radioisotopes[72].
THE ROLE OF DIAGNOSTIC RADIOLOGY IN MONITORING THE TREATMENT RESPONSE
Conventional tumor response criteria proposed by the World Health Organization WHO, the response evaluation criteria in solid tumors (RECIST), the modified RECIST (mRECIST), and the European Association for the Study of the Liver all use a decrease in tumor size or enhancement as an indicator of tumor response[73]. However, these methods may not be very reliable or very reproducible[74]. In addition, it can be difficult to use mRECIST and European Association for the Study of the Liver criteria because of the hypovascular character of ICC caused by its fibrous stroma[75].
The most recent innovation in functional MRI techniques has enabled quantitative assessment of cellular integrity. Data have suggested a potential value of functional MRI characteristics to determine the efficacy of local-regional therapies, particularly intraarterial therapies, for various hepatic malignancies[3].
Multiparametric MRI, which includes T2-weighted imaging, DWI, DCE-MRI, and spectroscopic imaging, is more sensitive to cellular changes throughout treatment, allowing for a more accurate description of the therapeutic response and prognosis. Using these methods, it is possible to detect early cellular damage in tumors before any change in size[76]. Increased cell membrane permeability caused by cellular necrosis allows water molecules to circulate freely, hence elevating ADC values[37].
Monitoring the response to TACE
A more precise and tailored patient selection to TACE would be possible with the help of forecasting therapeutic outcomes prior to this therapy and its probable efficacy. In this regard, Halappa et al. evaluated volumetric changes in ADC and contrast enhancement on MRI in ICC patients undergoing TACE. According to their results, patients with a percentage tumor volume increase in ADC of 45% or greater and 60% or greater above the threshold level of 1.60 × 10-3 mm2/sec had a favorable response to therapy and improved survival[76].
Recently, Pandey et al. performed a study to evaluate the efficacy of MRI-extracted features for assessment of response to TACE. They reported that changes in volumetric ADC can predict the outcome among these patients[77].
In another study, Pandey et al. reported that certain characteristics observed in baseline MRI scans can serve as predictors of higher survival rates in patients with ICC, independent of clinical factors. These predictors include a low ADC, indicating a smaller viable tumor volume and a higher level of necrosis and cell membrane damage. These characteristics suggest a more aggressive tumor phenotype, as indicated by the hypoxic environment and resistance to systemic therapy[78].
Monitoring the response to TARE
Over the past ten years, TARE has become more often used to treat ICCs that are not amenable to surgery. According to findings from many centers, such a strategy can increase survival[79-82]. However, there is much variation in the studies regarding the baseline clinical and tumor characteristics, the response, and survival, with the latter ranging from 9 to 22 months.
The potential to choose the best patients for ablative treatment is currently a subject of focus in radiomics. Mosconi et al. evaluated the associations between baseline CT quantitative features and the survival parameters[83].
Their findings support the theory that quantitative features could identify the ICC patients most likely to respond well to TARE. Hypervascular tumors with a more homogenous uptake of iodine contrast in the arterial phase were those most likely to be efficiently treated by TARE, and, of special importance, this hypervascularization could be assessed by radiomics.
It is becoming increasingly apparent that dosimetry is an essential component of TARE. A SARAH trial auxiliary analysis noted that patients whose tumors had all received a mean dosage of at least 100 Gy lived longer[84]. However, as dosimetry is typically only done after the process, there is currently no input on the actual dose distribution during treatment with TARE.
According to a recent study conducted as a phase I trial by Roosen et al., intraprocedural MRI-based dosimetry allowed for dynamic insight into the distribution of microspheres during TARE. This proof of concept offers unique opportunities to enhance therapy efficacy through treatment tailoring and to better comprehend microsphere distribution in vivo[85].
Monitoring the response to immunotherapy
Tumor immunotherapy has recently offered new insight into cancer treatment. Unfortunately, just about one-third of patients respond to immunotherapy, so the primary challenge is choosing the candidate who would benefit from this treatment and minimizing potential adverse effects[86,87].
The quantitative features connected to outcomes have been documented in several studies[88-90]. In a study, Zhang et al. explored the capability of MRI quantitative features for outcomes in ICC patients. They reported that four features significantly differed between the responded and non-responded patients with an AUC of 0.919[91].
Monitoring the response to external beam radiation therapy
The efficacy of EBRT for salvage therapy and consolidative treatment in unresectable ICC is supported by many studies[92]. Also, adjuvant EBRT with concomitant chemotherapy is conditionally advised for patients with resectable ICC who undergo curative resection, especially when there are high-risk prognostic markers such as positive surgical margins and lymph node involvement[17]. Across the range of studies, among patients treated with EBRT, 1-year OS ranged from 39% to 70%[93].
Imaging methods such as CT and MRI are frequently used to assess the effectiveness of the treatment. Imaging after EBRT may show a decrease in tumor size or enhancement, demonstrating a successful outcome. Given the gradual tumor shrinking that occurs right after EBRT, relying just on dimensional data would make it impossible to assess the treatment's effectiveness. Additionally, although diminished enhancement has traditionally been a biomarker of response in liver tumors, these tumors frequently exhibit persistent enhancement early after treatment, which can last up to and beyond 1 year and should not be confused with a viable tumor[94]. Other imaging characteristics may also provide information in the assessment of tumor response, particularly T2 signal intensity and ADC at MRI. Typically, tumors demonstrate a decrease in T2 signal intensity and DWI, and an increase in ADC after treatment, suggestive of tumor necrosis[95].
FUTURE DIRECTIONS AND CHALLENGES
Integrating AI with radiomics and genomics for personalized medicine
Integrating artificial intelligence (AI) with radiomics and genomics has the potential to revolutionize the field of personalized medicine in ICC management. By combining quantitative imaging features (radiomics) with molecular and genetic information (genomics), AI algorithms can potentially identify unique tumor signatures, predict treatment response, and estimate patient prognosis more accurately. This integration will facilitate the development of tailored therapeutic strategies based on individual tumor characteristics and genetic makeup, ultimately improving patient outcomes[96].
Development of AI-based prognostic models
AI-based prognostic models can help clinicians estimate patient survival and disease progression more accurately, enabling better-informed decisions regarding treatment and follow-up. By analyzing a wide range of data, including imaging, clinical, and molecular information, AI algorithms can identify subtle patterns and interactions that may be overlooked by traditional prognostic models[97].
Developing and validating AI-based prognostic models for ICC will require extensive research and access to large, diverse, and well-annotated datasets, requiring collaboration between institutions and researchers to share and standardize data. Additionally, the integration of these models into clinical practice will necessitate a rigorous evaluation of their performance, interpretability, and generalizability, as well as the development of user-friendly interfaces for clinical use. Moreover, an interdisciplinary collaboration among radiologists, oncologists, geneticists, and computer scientists will be crucial to advance the field and translate AI-based tools into clinical practice[98].
Ethical considerations and data security in AI applications
The implementation of AI in ICC diagnosis and treatment raises several ethical considerations and data security concerns. Ensuring patient privacy and the secure handling of sensitive medical data is paramount[98].
Another ethical concern is the potential for biases in AI algorithms, which may be introduced by the training data or the model design. Biased algorithms can lead to unfair or discriminatory treatment decisions, negatively impacting patient care. It is essential to continuously evaluate and refine AI models to minimize potential biases and ensure equitable and accurate predictions for all patient populations.
Finally, the widespread adoption of AI in clinical practice requires addressing issues related to accountability and liability in the case of AI-generated errors or misdiagnoses. Developing guidelines and legal frameworks that clarify the responsibilities of various stakeholders, including AI developers, healthcare providers, and patients, will be critical to address these concerns.
CONCLUSION
Radiology has a pivotal role in the management of intrahepatic cholangiocarcinoma (ICC), providing valuable information about tumor characteristics, staging, and treatment response. The integration of radiology and AI has the potential to revolutionize ICC management, leading to improved patient outcomes and personalized treatment strategies. However, continued research, collaboration, and careful consideration of ethical and data security issues are essential to successfully translate AI-based tools into clinical practice.
DECLARATIONS
Authors’ contributionsContributed significantly to the creation of this manuscript: Afyouni S, Borhani A, Mohseni A, Mirza-Aghazadeh-Attari M, Madani SP, Shahbazian H, Zandieh G, Pawlik TM, Kamel IR
Availability of data and materialsNot applicable.
Financial support and sponsorshipNone.
Conflicts of interestNot applicable.
Ethical approval and consent to participateNot applicable.
Consent for publicationNot applicable.
Copyright© The Author(s) 2023.
REFERENCES
1. Jiang Y, Jiang L, Li F, et al. The epidemiological trends of biliary tract cancers in the United States of America. BMC Gastroenterol 2022;22:546.
2. Sripa B, Brindley PJ, Mulvenna J, et al. The tumorigenic liver fluke Opisthorchis viverrini--multiple pathways to cancer. Trends Parasitol 2012;28:395-407.
3. Burak K, Angulo P, Pasha TM, Egan K, Petz J, Lindor KD. Incidence and risk factors for cholangiocarcinoma in primary sclerosing cholangitis. Am J Gastroenterol 2004;99:523-6.
4. Boberg KM, Bergquist A, Mitchell S, et al. Cholangiocarcinoma in primary sclerosing cholangitis: risk factors and clinical presentation. Scand J Gastroenterol 2002;37:1205-11.
5. Cheng R, Du Q, Ye J, Wang B, Chen Y. Prognostic value of site-specific metastases for patients with advanced intrahepatic cholangiocarcinoma: a SEER database analysis. Medicine 2019;98:e18191.
6. Chan KM, Tsai CY, Yeh CN, et al. Characterization of intrahepatic cholangiocarcinoma after curative resection: outcome, prognostic factor, and recurrence. BMC Gastroenterol 2018;18:180.
7. Bridgewater J, Galle PR, Khan SA, et al. Guidelines for the diagnosis and management of intrahepatic cholangiocarcinoma. J Hepatol 2014;60:1268-89.
8. Kim SA, Lee JM, Lee KB, et al. Intrahepatic mass-forming cholangiocarcinomas: enhancement patterns at multiphasic CT, with special emphasis on arterial enhancement pattern--correlation with clinicopathologic findings. Radiology 2011;260:148-57.
9. Hanna RF, Aguirre DA, Kased N, Emery SC, Peterson MR, Sirlin CB. Cirrhosis-associated hepatocellular nodules: correlation of histopathologic and MR imaging features. Radiographics 2008;28:747-69.
10. Granata V, Grassi R, Fusco R, et al. Intrahepatic cholangiocarcinoma and its differential diagnosis at MRI: how radiologist should assess MR features. Radiol Med 2021;126:1584-600.
11. Granata V, Fusco R, Venanzio Setola S, et al. Major and ancillary features according to LI-RADS in the assessment of combined hepatocellular-cholangiocarcinoma. Radiol Oncol 2020;54:149-58.
12. Fattach HE, Dohan A, Guerrache Y, et al. Intrahepatic and hilar mass-forming cholangiocarcinoma: Qualitative and quantitative evaluation with diffusion-weighted MR imaging. Eur J Radiol 2015;84:1444-51.
13. Park HJ, Kim YK, Park MJ, Lee WJ. Small intrahepatic mass-forming cholangiocarcinoma: target sign on diffusion-weighted imaging for differentiation from hepatocellular carcinoma. Abdom Imaging 2013;38:793-801.
14. Zou X, Luo Y, Li Z, et al. Volumetric apparent diffusion coefficient histogram analysis in differentiating intrahepatic mass-forming cholangiocarcinoma from hepatocellular carcinoma. J Magn Reson Imaging 2019;49:975-83.
15. Joo I, Lee JM, Yoon JH. Imaging diagnosis of intrahepatic and perihilar cholangiocarcinoma: recent advances and challenges. Radiology 2018;288:7-13.
16. Heye T, Merkle EM, Reiner CS, et al. Reproducibility of dynamic contrast-enhanced MR imaging. Part II. comparison of intra- and interobserver variability with manual region of interest placement versus semiautomatic lesion segmentation and histogram analysis. Radiology 2013;266:812-21.
17. Apisarnthanarax S, Barry A, Cao M, et al. External beam radiation therapy for primary liver cancers: an ASTRO clinical practice guideline. Pract Radiat Oncol 2022;12:28-51.
18. Kang Y, Lee JM, Kim SH, Han JK, Choi BI. Intrahepatic mass-forming cholangiocarcinoma: enhancement patterns on gadoxetic acid-enhanced MR images. Radiology 2012;264:751-60.
19. Hyder O, Marques H, Pulitano C, et al. A nomogram to predict long-term survival after resection for intrahepatic cholangiocarcinoma: an Eastern and Western experience. JAMA Surg 2014;149:432-8.
20. Spolverato G, Ejaz A, Kim Y, et al. Tumor size predicts vascular invasion and histologic grade among patients undergoing resection of intrahepatic cholangiocarcinoma. J Gastrointest Surg 2014;18:1284-91.
21. Mavros MN, Economopoulos KP, Alexiou VG, Pawlik TM. Treatment and Prognosis for patients with intrahepatic cholangiocarcinoma: systematic review and meta-analysis. JAMA Surg 2014;149:565-74.
22. Wang Y, Li J, Xia Y, et al. Prognostic nomogram for intrahepatic cholangiocarcinoma after partial hepatectomy. J Clin Oncol 2013;31:1188-95.
23. Amin MB, Greene FL, Edge SB, et al. The eighth edition AJCC cancer staging manual: continuing to build a bridge from a population-based to a more “personalized” approach to cancer staging. CA Cancer J Clin 2017;67:93-9.
24. Ma K, Dong B, Wang L, et al. Nomograms for predicting overall survival and cancer-specific survival in patients with surgically resected intrahepatic cholangiocarcinoma. Cancer Manag Res 2019;11:6907-29.
25. Shen H, Zhang S, Xia Y, et al. A nomogram in predicting risks of intrahepatic cholangiocarcinoma after partial hepatectomy for hepatolithiasis. J Gastrointest Surg 2021;25:2258-67.
26. Aherne EA, Pak LM, Goldman DA, et al. Intrahepatic cholangiocarcinoma: can imaging phenotypes predict survival and tumor genetics? Abdom Radiol 2018;43:2665-72.
27. Zhao L, Ma X, Liang M, et al. Prediction for early recurrence of intrahepatic mass-forming cholangiocarcinoma: quantitative magnetic resonance imaging combined with prognostic immunohistochemical markers. Cancer Imaging 2019;19:49.
28. Xue B, Wu S, Zhang M, et al. A radiomic-based model of different contrast-enhanced CT phase for differentiate intrahepatic cholangiocarcinoma from inflammatory mass with hepatolithiasis. Abdom Radiol 2021;46:3835-44.
29. Ren S, Li Q, Liu S, et al. Clinical value of machine learning-based ultrasomics in preoperative differentiation between hepatocellular carcinoma and intrahepatic cholangiocarcinoma: a multicenter study. Front Oncol 2021;11:749137.
30. Xue B, Wu S, Zheng M, et al. Development and validation of a radiomic-based model for prediction of intrahepatic cholangiocarcinoma in patients with intrahepatic lithiasis complicated by imagologically diagnosed mass. Front Oncol 2020;10:598253.
31. Zhang J, Wu Z, Zhang X, et al. Machine learning: an approach to preoperatively predict PD-1/PD-L1 expression and outcome in intrahepatic cholangiocarcinoma using MRI biomarkers. ESMO Open 2020;5:e000910.
32. Wang Y, Shao J, Wang P, et al. Deep learning radiomics to predict regional lymph node staging for hilar cholangiocarcinoma. Front Oncol 2021;11:721460.
33. Xu L, Wan Y, Luo C, et al. Integrating intratumoral and peritumoral features to predict tumor recurrence in intrahepatic cholangiocarcinoma. Phys Med Biol 2021;66:125001.
34. Deng L, Chen B, Zhan C, et al. A novel clinical-radiomics model based on sarcopenia and radiomics for predicting the prognosis of intrahepatic cholangiocarcinoma after radical hepatectomy. Front Oncol 2021;11:744311.
35. Peng YT, Zhou CY, Lin P, et al. Preoperative ultrasound radiomics signatures for noninvasive evaluation of biological characteristics of intrahepatic cholangiocarcinoma. Acad Radiol 2020;27:785-97.
36. Liang W, Xu L, Yang P, et al. Novel nomogram for preoperative prediction of early recurrence in intrahepatic cholangiocarcinoma. Front Oncol 2018;8:360.
37. King MJ, Hectors S, Lee KM, et al. Outcomes assessment in intrahepatic cholangiocarcinoma using qualitative and quantitative imaging features. Cancer Imaging 2020;20:43.
38. Park HJ, Park B, Park SY, et al. Preoperative prediction of postsurgical outcomes in mass-forming intrahepatic cholangiocarcinoma based on clinical, radiologic, and radiomics features. Eur Radiol 2021;31:8638-48.
39. Chu H, Liu Z, Liang W, et al. Radiomics using CT images for preoperative prediction of futile resection in intrahepatic cholangiocarcinoma. Eur Radiol 2021;31:2368-76.
40. Qin H, Hu X, Zhang J, et al. Machine-learning radiomics to predict early recurrence in perihilar cholangiocarcinoma after curative resection. Liver Int 2021;41:837-50.
41. Li MD, Lu XZ, Liu JF, et al. Preoperative survival prediction in intrahepatic cholangiocarcinoma using an ultrasound-based radiographic-radiomics signature. J Ultrasound Med 2022;41:1483-95.
42. Hao X, Liu B, Hu X, et al. A radiomics-based approach for predicting early recurrence in intrahepatic cholangiocarcinoma after surgical resection: a multicenter study. Annu Int Conf IEEE Eng Med Biol Soc 2021;2021:3659-62.
43. Yang Y, Zou X, Zhou W, et al. Multiparametric MRI-based radiomic signature for preoperative evaluation of overall survival in intrahepatic cholangiocarcinoma after partial hepatectomy. J Magn Reson Imaging 2022;56:739-51.
44. Nathan H, Aloia TA, Vauthey JN, et al. A proposed staging system for intrahepatic cholangiocarcinoma. Ann Surg Oncol 2009;16:14-22.
45. Jiang W, Zeng ZC, Tang ZY, et al. A prognostic scoring system based on clinical features of intrahepatic cholangiocarcinoma: the Fudan score. Ann Oncol 2011;22:1644-52.
46. Ma X, Liu L, Fang J, et al. MRI features predict microvascular invasion in intrahepatic cholangiocarcinoma. Cancer Imaging 2020;20:40.
47. Tsukamoto M, Yamashita YI, Imai K, et al. Predictors of cure of intrahepatic cholangiocarcinoma after hepatic resection. Anticancer Res 2017;37:6971-5.
48. Lee J, Kim SH, Kang TW, Song KD, Choi D, Jang KT. Mass-forming intrahepatic cholangiocarcinoma: diffusion-weighted imaging as a preoperative prognostic marker. Radiology 2016;281:119-28.
49. Zhou Y, Wang X, Xu C, et al. Mass-forming intrahepatic cholangiocarcinoma: can diffusion-weighted imaging predict microvascular invasion? J Magn Reson Imaging 2019;50:315-24.
50. de Jong MC, Nathan H, Sotiropoulos GC, et al. Intrahepatic cholangiocarcinoma: an international multi-institutional analysis of prognostic factors and lymph node assessment. J Clin Oncol 2011;29:3140-5.
51. Ali SM, Clark CJ, Mounajjed T, et al. Model to predict survival after surgical resection of intrahepatic cholangiocarcinoma: the Mayo Clinic experience. HPB 2015;17:244-50.
52. Al-Adra DP, Gill RS, Axford SJ, Shi X, Kneteman N, Liau SS. Treatment of unresectable intrahepatic cholangiocarcinoma with yttrium-90 radioembolization: a systematic review and pooled analysis. Eur J Surg Oncol 2015;41:120-7.
53. Chun YS, Pawlik TM, Vauthey JN. 8th edition of the AJCC cancer staging manual: pancreas and hepatobiliary cancers. Ann Surg Oncol 2018;25:845-7.
54. Zhang XF, Lv Y, Weiss M, et al. Should utilization of lymphadenectomy vary according to morphologic subtype of intrahepatic cholangiocarcinoma? Ann Surg Oncol 2019;26:2242-50.
55. Tsilimigras DI, Sahara K, Paredes AZ, et al. Predicting lymph node metastasis in intrahepatic cholangiocarcinoma. J Gastrointest Surg 2021;25:1156-63.
56. Weber SM, Ribero D, O'Reilly EM, Kokudo N, Miyazaki M, Pawlik TM. Intrahepatic cholangiocarcinoma: expert consensus statement. HPB 2015;17:669-80.
57. Songthamwat M, Chamadol N, Khuntikeo N, et al. Evaluating a preoperative protocol that includes magnetic resonance imaging for lymph node metastasis in the cholangiocarcinoma screening and care program (CASCAP) in Thailand. World J Surg Oncol 2017;15:176.
58. Paño B, Sebastià C, Ripoll E, et al. Pathways of lymphatic spread in gynecologic malignancies. Radiographics 2015;35:916-45.
59. Park HS, Lee JM, Choi JY, et al. Preoperative evaluation of bile duct cancer: MRI combined with MR cholangiopancreatography versus MDCT with direct cholangiography. AJR Am J Roentgenol 2008;190:396-405.
60. Hänninen EL, Pech M, Jonas S, et al. Magnetic resonance imaging including magnetic resonance cholangiopancreatography for tumor localization and therapy planning in malignant hilar obstructions. Acta Radiol 2005;46:462-70.
61. Park MS, Lee DK, Kim MJ, et al. Preoperative staging accuracy of multidetector row computed tomography for extrahepatic bile duct carcinoma. J Comput Assist Tomogr 2006;30:362-7.
62. Fábrega-Foster K, Ghasabeh MA, Pawlik TM, Kamel IR. Multimodality imaging of intrahepatic cholangiocarcinoma. Hepatobiliary Surg Nutr 2017;6:67-78.
63. Zhou Y, Zhou G, Gao X, Xu C, Wang X, Xu P. Apparent diffusion coefficient value of mass-forming intrahepatic cholangiocarcinoma: a potential imaging biomarker for prediction of lymph node metastasis. Abdom Radiol 2020;45:3109-18.
64. Ji GW, Zhu FP, Zhang YD, et al. A radiomics approach to predict lymph node metastasis and clinical outcome of intrahepatic cholangiocarcinoma. Eur Radiol 2019;29:3725-35.
65. Meng ZW, Lin XQ, Zhu JH, Han SH, Chen YL. A nomogram to predict lymph node metastasis before resection in intrahepatic cholangiocarcinoma. J Surg Res 2018;226:56-63.
66. Annunziata S, Caldarella C, Pizzuto DA, et al. Diagnostic accuracy of fluorine-18-fluorodeoxyglucose positron emission tomography in the evaluation of the primary tumor in patients with cholangiocarcinoma: a meta-analysis. Biomed Res Int 2014;2014:247693.
67. Fowler KJ, Saad NE, Linehan D. Imaging approach to hepatocellular carcinoma, cholangiocarcinoma, and metastatic colorectal cancer. Surg Oncol Clin N Am 2015;24:19-40.
68. Ma KW, Cheung TT, She WH, et al. Diagnostic and prognostic role of 18-FDG PET/CT in the management of resectable biliary tract cancer. World J Surg 2018;42:823-34.
69. Kirchner J, Sawicki LM, Deuschl C, et al. 18F-FDG PET/MR imaging in patients with suspected liver lesions: value of liver-specific contrast agent Gadobenate dimeglumine. PLoS One 2017;12:e0180349.
70. Dendl K, Koerber SA, Kratochwil C, et al. FAP and FAPI-PET/CT in malignant and non-malignant diseases: a perfect symbiosis? Cancers 2021;13:4946.
71. Hicks RJ, Roselt PJ, Kallur KG, Tothill RW, Mileshkin L. FAPI PET/CT: will it end the hegemony of 18F-FDG in oncology? J Nucl Med 2021;62:296-302.
72. Veldhuijzen van Zanten SEM, Pieterman KJ, Wijnhoven BPL, et al. FAPI PET versus FDG PET, CT or MRI for staging pancreatic-, gastric- and cholangiocarcinoma: systematic review and head-to-head comparisons of diagnostic performances. Diagnostics 2022;12:1958.
73. Corona-Villalobos CP, Kamel IR. Functional volumetric MRI in assessing treatment response to intra-arterial therapy of primary and secondary liver tumors. J Comput Assist Tomogr 2014;38:513-7.
74. Suzuki C, Torkzad MR, Jacobsson H, et al. Interobserver and intraobserver variability in the response evaluation of cancer therapy according to RECIST and WHO-criteria. Acta Oncol 2010;49:509-14.
75. Chung YE, Kim MJ, Park YN, et al. Varying appearances of cholangiocarcinoma: radiologic-pathologic correlation. Radiographics 2009;29:683-700.
76. Halappa VG, Bonekamp S, Corona-Villalobos CP, et al. Intrahepatic cholangiocarcinoma treated with local-regional therapy: quantitative volumetric apparent diffusion coefficient maps for assessment of tumor response. Radiology 2012;264:285-94.
77. Pandey A, Pandey P, Aliyari Ghasabeh M, et al. Unresectable intrahepatic cholangiocarcinoma: multiparametric mr imaging to predict patient survival. Radiology 2018;288:109-17.
78. Pandey A, Pandey P, Ghasabeh MA, et al. Baseline volumetric multiparametric mri: can it be used to predict survival in patients with unresectable intrahepatic cholangiocarcinoma undergoing transcatheter arterial chemoembolization? Radiology 2018;289:843-53.
79. Cucchetti A, Cappelli A, Mosconi C, et al. Improving patient selection for selective internal radiation therapy of intra-hepatic cholangiocarcinoma: a meta-regression study. Liver Int 2017;37:1056-64.
80. Ibrahim SM, Mulcahy MF, Lewandowski RJ, et al. Treatment of unresectable cholangiocarcinoma using Yttrium-90 microspheres: results from a pilot study. Cancer 2008;113:2119-28.
81. Rafi S, Piduru SM, El-Rayes B, et al. Yttrium-90 radioembolization for unresectable standard-chemorefractory intrahepatic cholangiocarcinoma: survival, efficacy, and safety study. Cardiovasc Intervent Radiol 2013;36:440-8.
82. Mouli S, Memon K, Baker T, et al. Yttrium-90 radioembolization for intrahepatic cholangiocarcinoma: safety, response, and survival analysis. J Vasc Interv Radiol 2013;24:1227-34.
83. Mosconi C, Cucchetti A, Bruno A, et al. Radiomics of cholangiocarcinoma on pretreatment CT can identify patients who would best respond to radioembolisation. Eur Radiol 2020;30:4534-44.
84. Hermann AL, Dieudonné A, Ronot M, et al. SARAH trial group. Relationship of tumor radiation-absorbed dose to survival and response in hepatocellular carcinoma treated with transarterial radioembolization with 90Y in the SARAH study. Radiology 2020;296:673-84.
85. Roosen J, Westlund Gotby LEL, Arntz MJ, et al. Intraprocedural MRI-based dosimetry during transarterial radioembolization of liver tumours with holmium-166 microspheres (EMERITUS-1): a phase I trial towards adaptive, image-controlled treatment delivery. Eur J Nucl Med Mol Imaging 2022;49:4705-15.
86. Chen DS, Mellman I. Elements of cancer immunity and the cancer-immune set point. Nature 2017;541:321-30.
87. Kim JM, Chen DS. Immune escape to PD-L1/PD-1 blockade: seven steps to success (or failure). Ann Oncol 2016;27:1492-504.
88. Shields AF, Jacobs PM, Sznol M, et al. Immune modulation therapy and imaging: workshop report. J Nucl Med 2018;59:410-7.
89. Ku YJ, Kim HH, Cha JH, et al. Correlation between MRI and the level of tumor-infiltrating lymphocytes in patients with triple-negative breast cancer. AJR Am J Roentgenol 2016;207:1146-51.
90. Ku YJ, Kim HH, Cha JH, et al. Predicting the level of tumor‐infiltrating lymphocytes in patients with triple-negative breast cancer: Usefulness of breast MRI computer-aided detection and diagnosis. J Magn Reson Imaging 2018;47:760-6.
91. Zhang J, Wu Z, Zhao J, et al. Intrahepatic cholangiocarcinoma: MRI texture signature as predictive biomarkers of immunophenotyping and survival. Eur Radiol 2021;31:3661-72.
92. Sebastian NT, Tan Y, Miller ED, Williams TM, Alexandra Diaz D. Stereotactic body radiation therapy is associated with improved overall survival compared to chemoradiation or radioembolization in the treatment of unresectable intrahepatic cholangiocarcinoma. Clin Transl Radiat Oncol 2019;19:66-71.
93. Brunner TB, Blanck O, Lewitzki V, et al. Stereotactic body radiotherapy dose and its impact on local control and overall survival of patients for locally advanced intrahepatic and extrahepatic cholangiocarcinoma. Radiother Oncol 2019;132:42-7.
94. Brook OR, Thornton E, Mendiratta-Lala M, et al. CT imaging findings after stereotactic radiotherapy for liver tumors. Gastroenterol Res Pract 2015;2015:126245.
95. Navin PJ, Olson MC, Mendiratta-Lala M, Hallemeier CL, Torbenson MS, Venkatesh SK. Imaging features in the liver after stereotactic body radiation therapy. Radiographics 2022;42:2131-48.
96. Arimura H, Soufi M, Kamezawa H, Ninomiya K, Yamada M. Radiomics with artificial intelligence for precision medicine in radiation therapy. J Radiat Res 2019;60:150-7.
97. Peng Q, Shen Y, Fu K, et al. Artificial intelligence prediction model for overall survival of clear cell renal cell carcinoma based on a 21-gene molecular prognostic score system. Aging 2021;13:7361-81.
Cite This Article
How to Cite
Download Citation
Export Citation File:
Type of Import
Tips on Downloading Citation
Citation Manager File Format
Type of Import
Direct Import: When the Direct Import option is selected (the default state), a dialogue box will give you the option to Save or Open the downloaded citation data. Choosing Open will either launch your citation manager or give you a choice of applications with which to use the metadata. The Save option saves the file locally for later use.
Indirect Import: When the Indirect Import option is selected, the metadata is displayed and may be copied and pasted as needed.
About This Article
Special Issue
Copyright
Data & Comments
Data
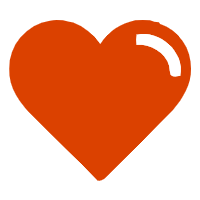
Comments
Comments must be written in English. Spam, offensive content, impersonation, and private information will not be permitted. If any comment is reported and identified as inappropriate content by OAE staff, the comment will be removed without notice. If you have any queries or need any help, please contact us at [email protected].