The role of miRNAs in the extracellular vesicle-mediated interplay between breast tumor cells and cancer-associated fibroblasts
Abstract
The tumor microenvironment (TME) of breast cancer (BC) is depicted as an immunosuppressive dwelling that comprises a myriad of cell types embedded in the extracellular matrix. As one of the most abundant cell populations within the TME, cancer-associated fibroblasts (CAFs) play indispensable roles in increasing cancer aggressiveness and promoting resistance to standard-of-care therapies. Extracellular vesicles (EVs) represent a diverse array of biological nanoparticles, encompassing exosomes, microvesicles, and apoptotic bodies. In recent years, these cell-derived membranous structures have raised great interest as they can encapsulate numerous types of cellular cargo, such as proteins, lipids, and miRNAs. By transmitting bioactive content to recipient cells, EVs play pivotal roles in intercellular communication between CAFs and tumor cells. EVs secreted from tumor cells typically activate resident fibroblasts to acquire a myofibroblastic phenotype, while EVs diffused by CAFs, in turn, substantially increase the progression of BC. This review summarizes the latest findings to highlight the functional role of EV cargo, especially miRNAs, in the regulatory network. A better understanding of the EV-mediated cell-cell interactions is crucial to achieving effective treatment in patients with BC.
Keywords
INTRODUCTIONS
According to the latest global cancer statistics, breast cancer (BC) is the most commonly diagnosed cancer in women, with approximately 2.3 million new cases in 2022[1]. Although much effort has been invested in improving the prognosis of patients in recent decades, BC continues to be one of the main causes of tumor-associated death in women[2]. The current treatment methods for BC are primarily surgery, radiotherapy, chemotherapy, endocrine therapy, and targeted therapy[3]. However, the immunosuppressive cells surrounding the tumor greatly limit the therapeutic efficacy and promote the progression of cancer[4].
The tumor microenvironment (TME), which is of vital significance in influencing the conditions that impact tumor development and progression, refers to the noncancerous elements in the vicinity of tumor cells, such as immune cells, stromal cells, the extracellular matrix (ECM), and signaling molecules produced by these various cells[5]. As a key component of the TME, cancer-associated fibroblasts (CAFs) exhibit a spindle-shaped morphology with irregular nuclei and abundant cytoplasm. Compared with normal fibroblasts (NFs), CAFs demonstrate increased activity levels and produce greater amounts of cytokines and immunomodulatory factors, thus modulating the TME and influencing tumor growth, angiogenesis, and metastasis[6]. Recent studies revealed that the intercellular communication between neoplastic cells and surrounding CAFs is not only based on ECM remodeling but also modulated by paracrine signals[7]. CAFs stimulate the survival and self-renewal programs of cancer cells, which increases their motility and promotes the metastasis of malignant cells[8].
Extracellular vesicles (EVs) are membranous vesicles that can be produced in response to various stimuli and are widely distributed in tissue fluids, such as human blood, lymph fluid, cerebrospinal fluid, urine, and saliva[9]. These cell-derived membranous structures can diffuse from a vast majority of eukaryotic cell types both under physiological conditions and during pathological processes[10]. According to studies on the formation processes of EVs, these vesicles can generally be classified into three main categories: exosomes, microvesicles, and apoptotic bodies. Exosomes typically originate through inward budding of the endosomal membrane and are released after the fusion of multivesicular endosomes (MVEs) with the cell membrane. Microvesicles are produced via outward budding of the plasma membrane, after which they are secreted into the extracellular environment[11]. On the other hand, apoptotic bodies are vesicles produced by the plasma membrane during cell disintegration as a result of programmed cell death[9]. EVs are capable of transporting proteins, lipids, and nucleic acids and act on recipient cells in a paracrine or endocrine manner, thereby affecting the physiological functions and phenotypes of the recipient cells[12]. These vesicles also play essential roles in multiple pathological conditions. For example, EVs can transfer chemotherapeutic agents out of cancer cells, leading to increased drug resistance in malignant tumors[13].
In recent decades, emerging evidence has shown that EVs secreted by CAFs can regulate tumor proliferation and migration through complex signaling networks; correspondingly, EVs secreted by tumor cells can induce the differentiation of NFs into CAFs, thus creating a specific microenvironment favorable for tumor growth[6]. Therapeutic strategies targeting EVs have become a promising direction in the research field of BC treatment. Here, to deepen the understanding of EV-based bidirectional signal transduction, we systematically summarized the interplay between CAFs and BC cells from tumorigenesis to tumor metastasis. Moreover, EV-related therapeutic approaches for the diagnosis and treatment of BC are also summarized in this review.
THE PATHOLOGICAL ROLE OF CAFs
The TME is mainly composed of tumor cells, stromal cells, and the extracellular matrix (ECM), among which stromal cells primarily include fibroblasts, immune cells, and endovascular cells[14]. As a prominent component of stromal cells in the TME, CAFs are identified by the presence of activation biomarkers, including α-smooth muscle actin (α-SMA) and fibroblast activation protein (FAP), along with a variety of secreted factors that are interrelated with ECM remodeling and immune infiltration[15].
The origin of CAFs
Currently, although the precise origin of CAFs has yet to be elucidated, evidence has shown that CAFs are derived from a wide range of sources. CAFs mainly originate from local resident fibroblasts and stellate cells, which can be activated by pathological stimulation. For example, quiescent stellate cells can acquire a myofibroblast-like phenotype and transcriptional features under the influence of inflammatory factors[16,17]. In addition, CAFs can arise from bone marrow-derived mesenchymal stem cells (BM-MSCs).
Subpopulations of CAFs
The diverse origins of CAFs lead to their phenotypic heterogeneity; thus, CAFs generate a variety of markers, such as α-SMA, FAP, fibroblast-specific protein-1 (FSP-1), S100A4, platelet-derived growth factor receptor (PDGFR), and vimentin (VIM)[22]. However, these markers lack specificity because they are not exclusively expressed by CAFs and can be detected in other healthy tissues. The increasing discovery of various subtypes of CAFs highlights the need for a more precise classification system to effectively guide therapeutic strategies targeting CAFs[23].
Research has revealed that two distinct subpopulations of CAFs were present in virtually all types of cancers: myofibroblastic CAFs (myCAFs), which are characterized by elevated levels of α-SMA expression, and inflammatory CAFs (iCAFs), which do not express α-SMA but instead secrete IL6[24,25]. MyCAFs are found adjacent to cancer cells and can produce ECM components and remodel the ECM, while iCAFs are located distant from tumor cells within the stroma and express high levels of cytokines and chemokines[26].
CAFs have been reported to arise from NFs that reside in the breast tissue of invasive lobular BC patients, among which CD26+ NFs are converted to protumorigenic iCAFs. These iCAFs play crucial roles in recruiting myeloid cells in a CXCL12-dependent manner. Additionally, they contribute to increasing the invasive ability of malignant tumor cells via increasing matrix metalloproteinase (MMP) activity[27]. Notably, the myCAF and iCAF subpopulations can be interconverted through specific signaling pathways[21]. These findings suggest that manipulating the subtypes of CAFs may hinder the growth and invasion of malignant tumors, offering valuable insights for cancer treatment.
Through single-cell RNA sequencing (scRNA-seq), researchers have identified a new subtype of CAF that expresses major histocompatibility complex (MHC) class II and CD74 but lacks expression of classical costimulatory molecules. These cells were defined as “antigen-presenting CAFs” (apCAFs). ApCAFs are capable of inducing CD4+ T cell activation in an antigen-specific fashion. Interestingly, two-dimensional (2D)-cultured apCAFs lost MHCII expression but upregulated myCAF markers, further confirming that subpopulations of CAFs are interconvertible[28]. By applying scRNA-seq, other rare subtypes of CAFs have been discovered, such as vascular CAFs (vCAFs), cycling CAFs (cCAFs), developmental CAFs (dCAFs), and matrix CAFs (mCAFs)[29]. Given the discrepancies in the classification of CAFs, in this review, we focused on the universal characteristics of CAFs rather than specific subgroups.
BIOGENESIS AND SUBPOPULATIONS OF EVs
EVs are cell-derived spherical particles enclosed by a phospholipid bilayer[30]. These lipid-membrane-bound vesicles typically range from 30 nm to 5 μm in size and carry a wide spectrum of cell-released biomolecules (e.g., proteins, metabolites, and nucleic acids)[31]. Under certain circumstances, they can also contain subcellular organelles such as mitochondria[32]. Malignant cells have recently been shown to release more EVs than normal neighboring cell types do, and they can be detected in bodily fluids, making them promising diagnostic and prognostic biomarkers for cancer treatment[33].
According to their biogenesis pathways, EVs can be categorized into three classic subtypes: exosomes, microvesicles, and apoptotic bodies[34]. The generation process and biological features vary distinctly among these subpopulations. Exosomes and microvesicles exhibit constitutive and inducible expression from a vast number of eukaryotes and show remarkable potential as blood-based or urine-based indicators for cancer patients, whereas apoptotic bodies partially reflect the prevalence and cellular composition of dying cells[35].
Exosomes
Exosomes (30-200 nm) are the smallest type of EVs with an endosomal origin[36,37]. The biogenesis of exosomes occurs through the inward growth of the plasma membrane and the generation of multivesicular bodies (MVBs) carrying intraluminal vesicles (ILVs)[38]. In the initiation stage of exosomes, the invaginated plasma membrane forms a cup-like structure housing cell-surface proteins and soluble proteins associated with the extracellular space[38,39]. The endosomal membrane subsequently generates an intraluminal budding process toward the lumen to create MVBs[40]. These endocytic structures end up in the cell in two ways, either merging with lysosomes or autophagosomes for degradation or integrating with the outer cell membrane, resulting in the secretion of the contained ILVs, commonly known as exosomes, into the ECM[38,41]. These vesicles can travel freely in body fluids to transmit information in autocrine, paracrine, or endocrine manners, thus modulating the biological behavior of target cells[42,43]. In pathological states, such as cancer, the dysregulation of exosomes has been found to be associated with the clinical features and survival outcomes of patients[44]. It was reported that the endogenously expressed protein TRIM1-269aa could be packed into exosomes, thus activating the PI3K/AKT/mTOR pathway and promoting the chemoresistance and metastasis of triple-negative breast cancer (TNBC)[45].
Microvesicles
Microvesicles (also referred to as ectosomes or microparticles) are apparently larger than exosomes[46]. Both exosomes and microvesicles are composed of a lipid bilayer membrane-enclosed structure, which protects the contents from degradation and potential environmental threats[11]. However, unlike exosomes, microvesicles are directly formed by outward budding and fission of the plasma membrane, followed by the instantaneous secretion of vesicles into the intercellular milieu[47]. To the best of our knowledge, the formation of microvesicles basically includes four stages: intracellular Ca2+ mobilization, remodeling of the actin cytoskeleton, kinase phosphorylation, and NF-κB activation[48]. Because they belong to distinct subsets of EVs, submicron-sized microvesicles have not attracted as much attention as exosomes have. However, in recent decades, there have been profound changes in our understanding of these particles[49]. Microvesicles have been shown to have a significant effect on various pathological processes, including vascular inflammation and aberrant angiogenesis[50,51]. Baj-Krzyworzeka et al. reported that tumor-derived microvesicles play an essential role in regulating the differentiation of monocytes in the TME[52]. Moreover, the functions of CD8+ T cells are strongly disrupted by microvesicle-shuttled PD-L1, which leads to a suppressive TME in TNBC patients[51].
Apoptotic bodies
Apoptotic bodies are a special subset of EVs that are generated from cells undergoing programmed cell death[53]. These vesicles are shown to have structures and phenotypic properties comparable to those of exosomes and microvesicles[35]. However, because of the lysis of apoptotic cells, the size and composition of apoptotic bodies vary, making it more difficult to establish common standards for investigation[54]. The formation of apoptotic bodies begins with cell shrinkage, nuclear chromatin condensation and internucleosomal fragmentation of genomic DNA, followed by extensive membrane ruffling and blebbing, leading to disintegration of the cellular content into separate membrane-encapsuled vesicles[55]. Once secreted into the extracellular space, similar to other subtypes of EVs, apoptotic bodies can also strongly regulate target cells by releasing their composition, such as histones, organelles, and DNA fragments[56]. According to Yin et al., apoptosis induced by Photodynamic therapy and chemodynamic therapy could generate δ-ALA-containing apoptotic bodies, which further facilitate tumor-killing effects in deep malignant cells[57]. Moreover, these vesicles showed distinct advantages in the application of nanomedicine systems. Using apoptotic bodies and Ti2N nanosheets, Yang et al. reported a new drug delivery system (Ti2N-DOX@ABs), which exhibits a high drug loading capacity[58].
On the basis of these three canonical subtypes, technological advances have further enabled more precise classification of these particles, such as matrix vesicles, migrasomes, and large oncosomes[59]. Nevertheless, separating multiple subpopulations of EVs is still challenging because reliable biomarkers are lacking. Therefore, the general term EV was adopted in most published articles.
CANCER-DERIVED EVs DICTATE PREFERABLE CAF CHARACTERISTICS FOR BC PROGRESSION
During the development and progression of a neoplasm, various cells, such as MSCs and macrophages, migrate into the stromal microenvironment in response to the recruiting effects of tumor cells, whereas fibroblasts typically populate both primary and distant lesions[60]. These cells within the TME are “reeducated” by malignant cells and subsequently acquire protumoral activities[60,61]. In summary, the cancer immunoediting process comprises three phases: tumor elimination, tumor dormancy, and tumor escape from immune surveillance[62]. As important factors involved in the interplay between tumor cells and nontumor cells, EVs significantly influence different stages of tumor progression, including angiogenesis, cell migration, tumor-associated immune modulation, and TME remodeling[63]. According to Li et al., tumor-derived miR-770 can be translocated to tumor-associated macrophages (TAMs) via exosomes, thereby mediating immune remodeling and drug resistance in TNBC cells[64]. Consistently, emerging evidence has shown that fibroblasts can also communicate with cancer cells via EVs to acquire a specific phenotype. As shown in Table 1, BC-derived EVs could effectively influence various biological processes in surrounding CAFs.
The role of cargos in BC-derived EVs on CAFs
Cargo | Target | Impact on CAFs | PMID |
FN and Ttg | Mitogenic signaling pathway | Cellular transformation | 21368175 |
miR-122 | PKM2 and GLUT1 | Suppress glucose uptake | 25621950 |
miR-9 | EFEMP1, MMP1, COL1A1 | Fibroblast activation | 27468688 |
TGF-β | MAPK signaling pathway | Myofibroblastic differentiation | 27913195 |
miR-105 | MYC signaling pathway | Promote glucose and glutamine metabolism | 29662176 |
miR-125b | TP53 and TP53INP1 | Fibroblast activation | 31044053 |
ITGB4 | BNIP3L | Promote aerobic glycolysis | 31534187 |
miR-146a | TXNIP | Fibroblast activation | 32268136 |
Survivin | SOD1 | Myofibroblastic differentiation | 32709750 |
miR-105 and miR-204 | RAGC | Suppress amino-acid-induced protein synthesis | 33345445 |
miR-370-3p | CYLD/NF-κB signaling pathway | Fibroblast activation | 33629796 |
Integrin αvβ1 | - | Fibroblast activation | 35923105 |
miR-130b-3p | SPIN90 | Fibroblast activation | 35948548 |
NME1 and NME2 | FASN, ACSS2 | Suppress fatty acid synthesis | 36010906 |
Tg2 | FAK signaling pathway | Fibroblast activation | 36475545 |
In 2014, Papi et al. proposed that exosomes released by BC cells under hypoxic conditions stimulate mammary gland fibroblasts into a proinflammatory phenotype[65]. Additionally, Jung et al. reported that tumor cells substantially increase the production of ECM fibrils in the early stage of BC, which facilitates the secretion of EVs that shuttle CAF-promoting molecules[66]. Adipose-derived stem cells (ASCs) treated with EVs released from BC cells subsequently undergo differentiation into myofibroblasts, with high expression of α-SMA and activation of TGF-β-associated signaling networks[67]. Moreover, EVs secreted from distinct subpopulations of tumor cells have been demonstrated to have different effects on CAFs. As reported by González-Callejo et al., cancer stem cell (CSC)-derived EVs can efficiently switch CAFs into a myofibroblastic phenotype, whereas EVs from nonstem cells stimulate secretory CAFs to help CSCs maintain stemness via the IL-6/IL-8 signaling pathway[68].
The transglutaminase family comprises versatile molecules with enzymatic and scaffolding functions that are involved in the modulation of cell destiny in numerous cellular systems and have been demonstrated to be crucial players in various pathological processes[69]. A decade ago, scientists demonstrated that BC-derived tissue transglutaminase transmitted by microvesicles was tightly associated with mitogenic signaling activities and fibroblast transformation[70]. It was further proposed by Schwager et al. that tissue transglutaminase 2-rich microvesicles could effectively stimulate fibroblasts into CAFs[71], thereby augmenting the migration of BC cells in the TME. Survivin (also known as BIRC5), which is highly expressed in neoplastic tissue[72,73], was reported to be intimately correlated with poor survival rates in multiple malignancies[72]. EV-encapsulated survivin derived from BC could be received by surrounding stromal cells and effectively promote their transdifferentiation into a myofibroblastic state[74].
MicroRNAs (miRNAs) are small endogenous RNAs that typically range from 18 to 25 nucleotides in length[75]. In 1993, the first miRNAs were discovered by Lee et al. in C. elegans[76]. Since then, many miRNAs have been found in a broad range of organisms[77]. Primary miRNAs (pri-miRNAs) are generated from RNA polymerase II/III-specific transcripts in the nucleus to form a hairpin structure[78]. A heterotrimeric complex microprocessor, which is composed of DROSHA and its cofactor DGCR8, subsequently cleaves the local hairpin structures into ~70-nucleotide small stem loops[79]. After being exported into the cytoplasm, the loop is cleaved by RNase III Dicer, thereby forming a double-stranded structure of miRNA and antisense miRNA[80]. The latter is typically degraded, whereas the other strand guides the miRNA-induced silencing complex (miRISC) to partially complementary sequences in target mRNAs for degradation and translational suppression[81,82]. MiRNAs are involved in multiple biological processes in cancer, such as cell apoptosis, migration, and angiogenesis, and also serve as biomarkers for cancer diagnosis and assessment of prognosis. Recently, emerging evidence has shown that miRNAs also play a functional role in EV-based intercellular crosstalk.
Recently, increasing evidence has suggested that EV-encapsulated miRNAs, such as miR-370-3p[83],
CAFs can undergo energy metabolism reprogramming under specific circumstances, thus influencing the aggressiveness of surrounding tumor cells[91]. Integrin beta4 (ITGB4) can be released by TNBC cells through exosomes, which then induces BNIP3L-dependent mitophagy and lactate generation in CAFs[92]. Tumor-released miRNAs, such as miR-105 and miR-122, have also been shown to be key players in the regulatory mechanism of glycolysis in fibroblasts[89,93]. In addition, an association was found between tumor-derived EVs and lipid metabolism in CAFs. Fibroblasts treated with NME1/2 protein-containing EVs presented markedly lower expression of genes associated with fatty acid and cholesterol metabolism[94].
CAF-DERIVED EVs CONFER MALIGNANT PROPERTIES ON BC CELLS
As the dominant cell population in the TME, CAFs are known to be pivotal for mediating cancer progression and drug resistance via deposition of the ECM[95]. The dense ECM in solid tumors significantly abrogates the penetration of therapeutic agents, thus substantially impairing the therapeutic results[96]. Recent research revealed that EVs can transmit various kinds of factors inherited from their parent cells, which also play an indispensable role in such cell-cell interactions. As shown in Table 2, CAF-derived EVs could significantly regulate the malignant behavior and the gene expression profiles in BC cells.
The effects of molecules in CAF-derived EVs on BC cells
Cargo | Target | Impact on CAFs | PMID |
Wnt10b | Wnt/β-catenin pathway | EMT/metastasis | 28394344 |
miR-7641 | HIF-1α pathway | Stemness/glycolysis | 34238918 |
miRNA-1-3p | GLIS1 | Proliferation/metastasis | 33154575 |
miR-221 | ER lo/Notch hi feed-forward loop | Metastases/therapy-resistant | 28202520 |
RN7SL1 | PRR, RIG-I | Proliferation/metastasis/therapy resistance | 28709002 |
miRNA-92 | LATS2 | Proliferation/metastasis | 33162971 |
miR-3613-3p | SOCS2 expression | Proliferation/metastasis | 32344463 |
Cd81 | Wnt11 | Metastasis | 23806092 |
miR-22 | Erα, PTEN | Tamoxifen resistance | 33173749 |
miR-4516 | FOSL1 | Proliferation | 31672492 |
miR-214 | TFAP2C | Metastasis | 36639824 |
miR-16 | CCNE1, TWIST1 | Metastasis | 31988451 |
miR-148a | WNT1, WNT10B | Metastasis | 31988451 |
CD44v3 | ESCRT signaling pathway | Radioresistance/expansion | 36106109 |
miR221/222 | MAPK signaling pathway | ER repression | 26186233 |
mtDNA | - | Metabolic changes | 29073103 |
lncRNA SNHG3 | miR-330-5p/PKM signaling pathway | Proliferation/metabolic changes | 31956955 |
miR-185-5p | - | Metastasis | 35317202 |
miR-652-5p | - | Metastasis | 35317202 |
miR-1246 | - | Metastasis | 35317202 |
miR-181d-5p | CDX2, HOXA5 | Proliferation/anti-apoptosis | 31955007 |
miR-500a-5p | USP28 | Proliferation/metastasis | 33664871 |
lncRNA H19 | miR-497/DNMT1 signaling pathway | Proliferation/metastasis/chemoresistance | 38558442 |
Cd81 | Wnt-PCP signaling pathway | Metastasis | 23260141 |
ADAM10 | RhoA and Notch signaling pathway | Motility | 25150980 |
circTBPL1 | miR-653-5p/TPBG signaling pathway | Proliferation/metastasis | 37495592 |
Proliferation, migration, and invasion
Recent studies have highlighted the significant impact of metastasis on the prognosis of patients with malignant tumors, with CAFs identified as key players in promoting metastasis across various cancer types. Luga et al. reported that stromal exosomes could considerably facilitate the protrusive activity and cell migration in BC through the Wnt-PCP signaling pathway[97]. Chen et al. reported that p85a-deficient CAFs could markedly augment BC progression through exosomal Wnt10b in a paracrine manner[98]. Upregulated Wnt10b in tumor cells led to activation of the Wnt/β-catenin signaling pathway, which efficiently induced the metastasis of tumor cells via EMT. Hypoxic CAFs secrete exosomes rich in the protein GPR64, which triggers the noncanonical NF-κB pathway to facilitate the expression of MMP9 and IL-8 in recipient BC cells, thereby increasing cell migration and invasion[99]. Furthermore, ADAM10-enriched exosomes secreted by CAFs were shown to activate the Notch pathway to augment the expression of aldehyde dehydrogenase and remarkably accelerate cancer metastasis by regulating the GTPase RhoA[100].
Recent studies revealed that CAF-released miRNAs also play essential roles in regulating biological processes in cancer cells. miR-4516 secreted by NFs effectively inhibits tumor progression by interacting with the target gene FOSL1. However, CAFs presented markedly lower levels of miR-4516, which resulted in proliferative and migrative features in TNBC cells[101]. Overexpression of miR-214 was found to be highly associated with stromal components, especially CAFs and MSCs, in samples from BC patients. Cancer-mediated activation of IL-6/STAT3 signaling in CAFs led to the accumulation of miR-214-enriched EVs in TME, which subsequently enhanced extravasation and metastasis formation of BC[102]. In coculture systems, miR-181d-5p encapsulated by CAF-derived EVs can be transmitted into MCF-7 cells and directly target the CDX2/HOXA5 signaling pathway by antagonizing apoptosis and promoting EMT in BC[103]. It was revealed by Tao et al. that miRNA-1-3p encapsulated by fibroblast-derived EVs could interact with Krüppel-like zinc-finger protein Gli-similar 1 (GLIS1) to inhibit BC growth and migration[104]. The expression level of miR-1-3p was significantly downregulated in CAF-secreted EVs, making it a potential therapeutic target in BC patients. miR-16 and miR-148a reportedly decelerate tumor metastasis, which is markedly suppressed in EVs from CAFs with FAK signaling activation[105]. In addition, according to our previous study, exosomal miR-500a-5p released by CAFs enhances the malignant properties of BC cells via interactions with ubiquitin-specific peptidase 28 (USP28)[106]. On the basis of advances in high-throughput sequencing technologies, more differentially expressed miRNAs have been identified between EVs derived from NFs and those derived from CAFs in BC patients. In the research of Liu et al., a miRNA array revealed that miR-3613-3p was sharply upregulated in CAF exosomes[107]. EV-shuttled miR-3613-3p from CAFs substantially enhanced the proliferative and migrative capabilities of BC cells via direct targeting of the tumor-suppressing gene SOCS2. According to Dou et al., a significantly increased expression level of miR-92 was found in CAF-derived exosomes, which led to the downregulation of the target gene LATS2 in BC cells, thus promoting both the proliferation and migration of cancer cells[108]. Consistently, a recent study further revealed that these miR-92-containing stromal exosomes could also increase the invasive ability of tumor cells by attenuating the expression of another target gene, G3BP2[109].
Circular RNAs (circRNAs) are single-stranded RNA molecules that are created through back-splicing, resulting in covalently closed loops[110]. Once considered transcription noise, these non-coding RNAs were subsequently shown to be crucial players in the progression of various cancers. Scientists have reported that circRNAs, such as circHIF1A and circRNA-CREIT, can also be packaged into EVs, thus influencing cell-cell communication in BC[111,112]. With respect to tumor-stromal interactions, exosomal circTBPL1 secreted from CAFs could markedly protect TPBG from miRNA-induced degradation by acting as a miR-653-5p sponge, consequently reinforcing the proliferation and metastasis of BC cells[113].
The signal recognition particle (SRP), an ancient ribonucleoprotein machine composed of a 7S RNA and six polypeptides, is crucial for the delivery of secretory and membrane proteins across the endoplasmic reticulum (ER)[114]. SRP RNA plays a fundamental role in SRP assembly, translational elongation stalling, and stimulation of SRP guanosine triphosphatases[115]. In BC, unshielded SRP RNA RN7SL1 covered by stromal EVs could be released into the extracellular space and received by tumor cells, thereby exacerbating cell proliferation and migration by triggering the pattern recognition receptor RIG-I[116].
Metabolic changes
It has been seven decades since Otto Warburg discovered reprogrammed metabolism in malignant cells, which includes increased glucose uptake and increased glycolysis[117]. Alterations in metabolic processes in neoplasms, such as increased cell growth, metastasis and angiogenesis, are interrelated with the phenotypic traits of tumor cells compared with those of normal cells[118]. These distinct metabolic characteristics satisfy the increased energy demand in neoplasms, thus playing a crucial role in the development and progression of cancer[119]. In recent years, emerging evidence has shown that the functional significance of these biological changes in metabolism is not only based on different BC subtypes but also associated with communication between tumor cells and intricate TMEs[120].
A recent study revealed that miR-7641 plays a key role in the regulation of glycolysis by interacting with HIF-1a signaling networks. Compared with that in NFs, a lower expression level of miR-7641 was found in CAF-derived small EVs, which facilitated glycolysis and adjacent stem cell populations in the BC niche[121].
The mechanism of competitive endogenous RNA (ceRNA) activity involves transcripts that possess shared miRNA binding sites engaging in competition for posttranscriptional regulation during the progression of different types of cancer[122]. The CAF-derived lncRNA SNHG3 can be packaged into exosomes and serve as a miR-330-5p sponge after being received by BC cells, thereby protecting PKM from degradation. Upregulated PKM subsequently suppresses mitochondrial oxidative phosphorylation and markedly increases glycolytic carboxylation, which leads to accelerated cancer growth[123].
Therapy resistance
Drug resistance resulting from long-term drug use has emerged as a critical issue in the treatment of malignant tumors. Tumor drug resistance is influenced not only by tumor cells and the neoplasm microenvironment, but also by the role of CAFs, which has become a prominent topic in research. In recent years, scientists have found that EVs played a significant role in CAF-induced therapy resistance. Despite the serious adverse reactions, chemotherapy remains the gold standard for BC treatment[124]. Epirubicin is a widely used anthracycline for BC patients in oncological practice[125]. When stimulated by epirubicin, stressed CAFs can subsequently release exosomes in a TCF12-mediated manner, which increases the expression of CXCR4 and c-Myc in ER+ BC cells to facilitate treatment resistance to epirubicin[126]. Paclitaxel (PTX) is considered the gold standard chemotherapeutic drug for various malignancies, such as pancreatic cancer and BC[127]. In BC research, Tao et al. reported that hypoxia-induced CAFs released lncRNA H19, which could be delivered to surrounding tumor cells via EVs, which led to paclitaxel resistance via regulation of the expression of miR-497[128].
Radiotherapy plays an indispensable part in the recent progress of anti-cancer therapies, including elevation in organ-sparing treatment and achieving lower local recurrence and longer survival time[129]. CAFs triggered by radiotherapy can secrete increased levels of EVs into the TME, which substantially increase the survival of BC cells through exosomal srpRNA RN7SL1 and the expression of the heparan sulfate proteoglycan CD44v3 on the outside membrane of the vesicles[116,130].
Endocrine therapy markedly prolongs overall survival in both pre- and postmenopausal patients with hormone receptor (HR)-positive BC[131]. However, the development of endocrine resistance was a major obstacle to existing treatments. Novel approaches showed that the expression of ER was at least partially dependent on the regulation of CAF-derived EVs. It was reported by Shah et al. that miR221/222 in the exosomes at least partially lead to MAPK-related ER repression in the basal-like BC subtype[132]. miR-221 shuttled by stromal microvesicles could transfer into tumor cells by endocytosis and remarkably triggered the ERlo/Notchhi feed-forward loop, which converts noncancer stem cells into CSCs, thus contributing to endocrine resistance in patients with luminal BC[133]. A novel CAF subpopulation, CD63+ CAFs, can release large amounts of miR-22 encapsulated by EVs, which directly target ERα and PTEN after being taken up by BC cells. The loss of ERα and PTEN expression subsequently led to decreased tamoxifen sensitivity in BC patients[134]. In ER+ BC, the transition from a hormonal therapy-sensitive state to a treatment-resistant state was found to be tightly associated with host mtDNA-mediated oxidative phosphorylation (OXPHOS). Sansone et al. proposed that CAF-derived EVs play an essential role in the horizontal transfer of the full mitochondrial genome into BC cells, which substantially induces cancer stem-like cells, thus contributing to endocrine therapy resistance in BC patients[135].
THE ROLE OF IMMUNE CELLS IN EV-BASED CROSSTALK IN BC MICROENVIRONMENT
The immune cell population comprises functionally diverse subtypes, such as T cells, B cells, and natural killer (NK) cells, which are essential for protective immunity against pathogens and malignancies[136,137].
Cancer cell-derived EVs deliver bioactive molecules to immune cells to establish an immune escaping and immunosuppressive microenvironment. Exosomes derived from malignant cells decrease the expression of NKG2D on NK cells and CD8+ T cells, resulting in a reduction in their cytotoxic capabilities in vitro, thereby facilitating immune evasion and tumor progression[138]. In BC, an increase in PD-L1-shuttled EVs secreted from BC cells following oscillatory strain leads to additive T cell suppressive functions in the TME[139]. Similarly, it has been reported that a TGF-β type II receptor (TβRII) encapsuled by BC-derived EVs can trigger CD8+ T cells to enter an exhausted state, thus leading to the failure of immunotherapy[140].
CAF-derived EVs can also induce immune tolerance and facilitate immune evasion by modulating the activity and functionality of immune cells[141]. In pancreatic ductal adenocarcinoma, CAF-derived EVs carrying lncRNA RP11-161H23.5 have been shown to induce immune escape by downregulating HLA-A expression levels and inhibiting the activation of CD8+ T cells[142]. Additionally, Shang et al. discovered that hypoxia-induced CAF-derived exosomes encapsulating circHIF1A promoted the proliferation and invasive activity of hepatocellular carcinoma while inhibiting the cytotoxicity of CD8+ T cells through the upregulation of PD-L1 expression[143]. Although increasing evidence has proven the immunoregulatory function of CAFs in BC, few studies have focused on the role of EVs in this process. According to Liao et al., suppression of FAP+ CAFs significantly contributes to their polarization from Th2 to Th1 cells in the TME, which attenuates tumor angiogenesis and lymphangiogenesis[144]. Moreover, CAFs in BC enhance the infiltration and differentiation of CD4+CD25+ Tregs via CXCL12/SDF1-α, thereby hindering the function of effector T cells[145]. Recently, integrated transcriptomics and proteomics analyses revealed an EV-specific signaling network to predict the immunosuppressive effects of CAF-released EVs on macrophages and CD8+ T cells[146]. However, the underlying mechanism remains to be elucidated.
DISCUSSION
In recent years, the incidence of cancer has increased, and cancer is the leading cause of mortality worldwide. BC is the most prevalent malignant tumor among women and represents a significant threat to women’s lives and health[147]. Although personalized comprehensive treatment approaches have significantly increased the survival rates of patients, tumor recurrence and metastasis are still the core problems affecting the outcomes of patients with malignancies[148]. In recent years, advances in technologies have allowed a deeper understanding of the TME. This comprehensive network of cellular organization, which consists of multiple cell types, was shown to play a dual role[62,149]. Along with the development and progression of cancer, numerous factors within the TME can not only exert antitumor effects by damaging immunogenic tumor variants but also fuel the aggressiveness of malignant cells by shaping tumor immunogenicity[62].
CAFs can promote tumor growth by providing cancer cells with a more proliferative and invasive nature[150]. To the best of our knowledge, there are multiple methods of intercellular communication between CAFs and BC cells, among which the secretory properties of CAFs play essential roles in the reconstruction of the TME. For example, fibroblast growth factors (FGFs) expressed in stromal fibroblasts effectively trigger the paracrine stimulation of cancer cells by activating fibroblast growth factor receptors (FGFRs), which leads to tumor progression[151]. It has been reported that CAF-derived basic fibroblast growth factor (bFGF/FGF2) can substantially lead to BC proliferation via sharply increasing the phosphorylation of Akt[152]. Furthermore, the activation of FGFR3, which is expressed on BC cells, contributes to resistance to endocrine therapy through the MAPK/PI3K signaling pathway[153]. Recently, emerging evidence has demonstrated that EVs are also deeply involved in the communication between CAFs and cancer cells in a variety of pathophysiological conditions.
EVs are heterogeneous lipid bilayer vesicles released by living cells that are associated with various biological processes as intermediaries for cell-cell interactions[154]. In addition to approaches in cancer immunology, increasing evidence has indicated that EVs play indispensable roles in the modulation of the immune response in the TME[31]. As depicted in Figure 1, bioactive molecules contained in BC-derived EVs effectively lead to differentiation from NFs to CAFs, while various types of CAFs could, in turn, regulate the behavior of BC cells in an EV-dependent manner.
Scientists found that the diffusion and capture of EVs are modulated in a more controlled way than they expected[155]. However, the regulatory mechanism of EV generation is yet to be elucidated. Hypoxia is a crucial hallmark of various malignancies. On the basis of the neoplastic immune context, hypoxia-mediated processes elicit complicated intercellular contacts, and recent studies revealed that EVs might play a pivotal role in such processes[156]. It has been reported that the resistance-associated lncRNA H19 is markedly overexpressed in EVs derived from hypoxia-induced CAFs[128]. Hypoxia-induced activation of the oxidized ataxia-telangiectasia mutated (ATM) gene can phosphorylate both BNIP3 and ATP6V1G1 to increase the number of EVs secreted from breast CAFs by promoting the accumulation and fusion of autophagosomes[99]. In addition, reactive oxygen species (ROS)-induced autophagy has been shown to be responsible for the loss of CAV-1 and subsequently triggers the HIF-1α signaling pathway under ROS-mediated pseudohypoxic conditions[157]. In BC, genotoxic chemotherapy-induced production of ROS in CAFs could lead to TCF12-dependent diffusion of EVs, thus facilitating chemoresistance in tumor cells[126].
Focal adhesion kinase (FAK) is a cytoplasmic protein tyrosine kinase upregulated in numerous advanced-stage solid tumors, which modulates adhesion-related cell migration, proliferation, and drug resistance[105]. In a model of hypertrophic scar formation, fibroblast-specific FAK-deficient mice have enormously suppressed inflammatory response and fibrosis than animals in the control group[158]. a recent study revealed that the activation of FAK in CAFs plays a central role in mediating cell-cell interactions with BC cells via the regulation of secreted EVs[105]. Depletion of FAK in CAFs effectively resulted in a reduction in the protein and RNA components of these vesicles.
Notch signaling is an evolutionarily conserved pathway that directly links cell components at the cytoplasmic membrane with the modulation of transcription[15]. Fibroblasts within the TME can be directly stimulated through contact with tumor cells via the activation of Notch signaling[159]. Typically triggered by BC, upregulation of the stromal NOTCH-MYC pathway in CAFs is also tightly associated with alterations in the composition of EVs. The activation of the signaling pathway resulted in increased POL3-dependent expression of the exosomal srpRNA RN7SL1. Unshielded RN7SL1 taken up by recipient neoplastic cells could, in turn, promote tumor progression and drug resistance in BC patients[116].
Increasing evidence has demonstrated the ability of nonmalignant stromal components to normalize tumor cells, indicating the promising role of strategies targeting protumoral communication between cancer cells and fibroblasts in the immunosuppressive TME[160]. In BC, a CAF regulator named dasatinib was shown to have synergistic antitumor effects with an immunogenic cell death (ICD) inducer (epirubicin) by reprogramming CAFs through the modulation of apoptotic vesicles[96]. In addition, scientists have reported that integrin αvβ1 plays a key role in the retention of EVs; thus, suppressing the integrin αvβ1 complex with a galectin-3 inhibitor could significantly attenuate the differentiation of fibroblasts into CAFs[161]. According to Papi et al., nuclear receptors such as pioglitazone can also abrogate the functions of hypoxic MCF7-derived exosomes, thereby promoting a proinflammatory phenotype of fibroblasts in the BC stem cell niche[65].
In addition, tumor-derived exosomes are released by tumor cells and carry substances that can reflect the features of parental tumor cells. Therefore, exosomes can be used as tumor diagnostic markers. A promising clinical trial (NCT03974204) is ongoing to explore the use of exosomes in cerebrospinal fluid as diagnostic markers in BC patients with leptomeningeal metastasis[162]. Although multiple types of EVs, such as exosomes and microvesicles, play pivotal roles in the TME, only exosomes have a suitable size for potential application as novel drug vehicles in cancer treatment[163]. After artificial modification, engineered exosomes carrying antitumor agents could efficiently release these cargos into tumor sites with fewer side effects[164]. Recently, Li et al. reported a HER2-specific exosome-T vaccine that could substantially augment immune functions in patients with HER2-positive BC[165].
CONCLUSION
Tumor development is a comprehensive process that is not only interrelated with biological alterations inside tumor cells, such as altered cell behavior and dysregulated metabolic pathways, but also related to interactions with surrounding stromal cells. EVs are among the most significant media involved in cell-cell contacts. Hence, EV-dependent communication between breast tumor cells and CAFs has received much attention in recent decades. Here, we systematically reviewed the intercellular networks associated with EV transportation and the underlying mechanisms of the phenotypical alterations in both fibroblasts and malignant cells. Strategies targeting secreted EVs in the TME are likely to play a pivotal role in the treatment of patients with BC.
DECLARATIONS
Authors’ contributions
Made substantial contributions to the conception and design of the study, supervision: Yang Q
Writing - original draft preparation: Li C, Yang C
Writing - review and editing, funding acquisition: Yang Q, Li C
Availability of data and materials
Not applicable.
Financial support and sponsorship
This work was supported by the National Natural Science Foundation of China (No.82304971; No. 82004122), the Natural Science Foundation of Shandong Province (No. ZR2023QH194), Special Foundation for Taishan Scholars (No. ts20190971), Foundation from Clinical Research Center of Shandong University (No.2020SDUCRCA015).
Conflicts of interest
All authors declared that there are no conflicts of interest.
Ethical approval and consent to participate
Not applicable.
Consent for publication
Not applicable.
Copyright
© The Author(s) 2024.
REFERENCES
1. Bray F, Laversanne M, Sung H, et al. Global cancer statistics 2022: GLOBOCAN estimates of incidence and mortality worldwide for 36 cancers in 185 countries. CA Cancer J Clin 2024;74:229-63.
2. Britt KL, Cuzick J, Phillips KA. Key steps for effective breast cancer prevention. Nat Rev Cancer 2020;20:417-36.
3. Osborne CK, Knight WA 3rd, Yochmowitz MG, McGuire WL. Modern approaches to the treatment of breast cancer. Blood 1980;56:745-52.
4. Hong J, Lee JH, Zhang Z, et al. PRC2-mediated epigenetic suppression of type I IFN-STAT2 signaling impairs antitumor immunity in luminal breast cancer. Cancer Res 2022;82:4624-40.
5. Rodríguez-Bejarano OH, Parra-López C, Patarroyo MA. A review concerning the breast cancer-related tumour microenvironment. Crit Rev Oncol Hematol 2024;199:104389.
6. Cao L, Ouyang H. Intercellular crosstalk between cancer cells and cancer-associated fibroblasts via exosomes in gastrointestinal tumors. Front Oncol 2024;14:1374742.
7. DiPersio CM, Van De Water L. Integrin regulation of CAF differentiation and function. Cancers 2019;11:715.
8. Fiori ME, Di Franco S, Villanova L, Bianca P, Stassi G, De Maria R. Cancer-associated fibroblasts as abettors of tumor progression at the crossroads of EMT and therapy resistance. Mol Cancer 2019;18:70.
9. Wu J, Ma Y, Chen Y. Extracellular vesicles and COPD: foe or friend? J Nanobiotechnol 2023;21:147.
10. Hu W, Liu C, Bi ZY, et al. Comprehensive landscape of extracellular vesicle-derived RNAs in cancer initiation, progression, metastasis and cancer immunology. Mol Cancer 2020;19:102.
11. Chen S, Zhu X, Huang S. Clinical applications of extracellular vesicle long RNAs. Crit Rev Clin Lab Sci 2020;57:508-21.
12. Yáñez-Mó M, Siljander PRM, Andreu Z, et al. Biological properties of extracellular vesicles and their physiological functions. J Extracell Vesicles 2015;4:27066.
13. Shah R, Patel T, Freedman JE. Circulating extracellular vesicles in human disease. N Engl J Med 2018;379:958-66.
14. Du Y, Sui Y, Cao J, et al. Dynamic changes in myofibroblasts affect the carcinogenesis and prognosis of bladder cancer associated with tumor microenvironment remodeling. Front Cell Dev Biol 2022;10:833578.
15. Meurette O, Mehlen P. Notch signaling in the tumor microenvironment. Cancer Cell 2018;34:536-48.
16. Zhang Q, Lou Y, Fang H, et al. Cancer-associated fibroblasts under therapy-induced senescence in the tumor microenvironment (Review). Exp Ther Med 2024;27:150.
17. Omary MB, Lugea A, Lowe AW, Pandol SJ. The pancreatic stellate cell: a star on the rise in pancreatic diseases. J Clin Invest 2007;117:50-9.
18. Barcellos-de-Souza P, Comito G, Pons-Segura C, et al. Mesenchymal stem cells are recruited and activated into carcinoma-associated fibroblasts by prostate cancer microenvironment-derived TGF-β1. Stem Cells 2016;34:2536-47.
19. Iwano M, Plieth D, Danoff TM, Xue C, Okada H, Neilson EG. Evidence that fibroblasts derive from epithelium during tissue fibrosis. J Clin Invest 2002;110:341-50.
20. Zeisberg EM, Potenta S, Xie L, Zeisberg M, Kalluri R. Discovery of endothelial to mesenchymal transition as a source for carcinoma-associated fibroblasts. Cancer Res 2007;67:10123-8.
21. Yang D, Liu J, Qian H, Zhuang Q. Cancer-associated fibroblasts: from basic science to anticancer therapy. Exp Mol Med 2023;55:1322-32.
22. Nurmik M, Ullmann P, Rodriguez F, Haan S, Letellier E. In search of definitions: cancer-associated fibroblasts and their markers. Int J Cancer 2020;146:895-905.
23. Guo T, Xu J. Cancer-associated fibroblasts: a versatile mediator in tumor progression, metastasis, and targeted therapy. Cancer Metastasis Rev 2024;43:1095-116.
24. Kennel KB, Bozlar M, De Valk AF, Greten FR. Cancer-associated fibroblasts in inflammation and antitumor immunity. Clin Cancer Res 2023;29:1009-16.
25. Öhlund D, Handly-Santana A, Biffi G, et al. Distinct populations of inflammatory fibroblasts and myofibroblasts in pancreatic cancer. J Exp Med 2017;214:579-96.
26. Menezes S, Okail MH, Jalil SMA, Kocher HM, Cameron AJM. Cancer-associated fibroblasts in pancreatic cancer: new subtypes, new markers, new targets. J Pathol 2022;257:526-44.
27. Houthuijzen JM, de Bruijn R, van der Burg E, et al. CD26-negative and CD26-positive tissue-resident fibroblasts contribute to functionally distinct CAF subpopulations in breast cancer. Nat Commun 2023;14:183.
28. Elyada E, Bolisetty M, Laise P, et al. Cross-species single-cell analysis of pancreatic ductal adenocarcinoma reveals antigen-presenting cancer-associated fibroblasts. Cancer Discov 2019;9:1102-23.
29. Bartoschek M, Oskolkov N, Bocci M, et al. Spatially and functionally distinct subclasses of breast cancer-associated fibroblasts revealed by single cell RNA sequencing. Nat Commun 2018;9:5150.
30. van der Pol E, Böing AN, Harrison P, Sturk A, Nieuwland R. Classification, functions, and clinical relevance of extracellular vesicles. Pharmacol Rev 2012;64:676-705.
31. Marar C, Starich B, Wirtz D. Extracellular vesicles in immunomodulation and tumor progression. Nat Immunol 2021;22:560-70.
32. Puhm F, Afonyushkin T, Resch U, et al. Mitochondria are a subset of extracellular vesicles released by activated monocytes and induce type I IFN and TNF responses in endothelial cells. Circ Res 2019;125:43-52.
33. Xu R, Rai A, Chen M, Suwakulsiri W, Greening DW, Simpson RJ. Extracellular vesicles in cancer - implications for future improvements in cancer care. Nat Rev Clin Oncol 2018;15:617-38.
34. Colombo M, Raposo G, Théry C. Biogenesis, secretion, and intercellular interactions of exosomes and other extracellular vesicles. Annu Rev Cell Dev Biol 2014;30:255-89.
35. Cheng L, Hill AF. Therapeutically harnessing extracellular vesicles. Nat Rev Drug Discov 2022;21:379-99.
36. Zhang F, Jiang J, Qian H, Yan Y, Xu W. Exosomal circRNA: emerging insights into cancer progression and clinical application potential. J Hematol Oncol 2023;16:67.
38. Kalluri R, LeBleu VS. The biology, function, and biomedical applications of exosomes. Science 2020;367:eaau6977.
39. Syn N, Wang L, Sethi G, Thiery JP, Goh BC. Exosome-mediated metastasis: from epithelial-mesenchymal transition to escape from immunosurveillance. Trends Pharmacol Sci 2016;37:606-17.
40. Baietti MF, Zhang Z, Mortier E, et al. Syndecan-syntenin-ALIX regulates the biogenesis of exosomes. Nat Cell Biol 2012;14:677-85.
41. Lakkaraju A, Rodriguez-Boulan E. Itinerant exosomes: emerging roles in cell and tissue polarity. Trends Cell Biol 2008;18:199-209.
42. Krylova SV, Feng D. The machinery of exosomes: biogenesis, release, and uptake. Int J Mol Sci 2023;24:1337.
43. Pan M, Zhang Z, Wang Q, Shang L. Exosome-loaded microcarriers for intraocular drug delivery. Sci Bull 2024;69:434-6.
44. Tang W, Xia M, Liao Y, Fang Y, Wen G, Zhong J. Exosomes in triple negative breast cancer: from bench to bedside. Cancer Lett 2022;527:1-9.
45. Li Y, Wang Z, Yang J, et al. CircTRIM1 encodes TRIM1-269aa to promote chemoresistance and metastasis of TNBC via enhancing CaM-dependent MARCKS translocation and PI3K/AKT/mTOR activation. Mol Cancer 2024;23:102.
46. Bian X, Xiao YT, Wu T, et al. Microvesicles and chemokines in tumor microenvironment: mediators of intercellular communications in tumor progression. Mol Cancer 2019;18:50.
47. Quesenberry PJ, Aliotta JM. Cellular phenotype switching and microvesicles. Adv Drug Deliv Rev 2010;62:1141-8.
48. Nieri D, Neri T, Petrini S, Vagaggini B, Paggiaro P, Celi A. Cell-derived microparticles and the lung. Eur Respir Rev 2016;25:266-77.
49. Stępień EŁ, Durak-Kozica M, Kamińska A, et al. Circulating ectosomes: determination of angiogenic microRNAs in type 2 diabetes. Theranostics 2018;8:3874-90.
50. Wang Y, Chen LM, Liu ML. Microvesicles and diabetic complications-novel mediators, potential biomarkers and therapeutic targets. Acta Pharmacol Sin 2014;35:433-43.
51. Li C, Qiu S, Jin K, et al. Tumor-derived microparticles promote the progression of triple-negative breast cancer via PD-L1-associated immune suppression. Cancer Lett 2021;523:43-56.
52. Baj-Krzyworzeka M, Mytar B, Szatanek R, et al. Colorectal cancer-derived microvesicles modulate differentiation of human monocytes to macrophages. J Transl Med 2016;14:36.
53. Majno G, Joris I. Apoptosis, oncosis, and necrosis. An overview of cell death. Am J Pathol 1995;146:3-15.
54. Li C, Wang X, Chen T, et al. Huaier induces immunogenic cell death via CircCLASP1/PKR/eIF2α Signaling pathway in triple negative breast cancer. Front Cell Dev Biol 2022;10:913824.
55. Wen J, Creaven D, Luan X, Wang J. Comparison of immunotherapy mediated by apoptotic bodies, microvesicles and exosomes: apoptotic bodies’ unique anti-inflammatory potential. J Transl Med 2023;21:478.
56. van Dommelen SM, Vader P, Lakhal S, et al. Microvesicles and exosomes: opportunities for cell-derived membrane vesicles in drug delivery. J Control Release 2012;161:635-44.
57. Yin Q, Zhang J, Zhang H, et al. Cascade nanoreactor employs mitochondrial-directed chemodynamic and δ-ALA-mediated photodynamic synergy for deep-seated oral cancer therapy. Adv Healthc Mater 2024;13:e2304639.
58. Yang K, Ren D, Wang Z, et al. Apoptotic bodies encapsulating Ti2N nanosheets for synergistic chemo-photothermal therapy. Nanotechnology 2024;35:365703.
59. Eguchi T, Sheta M, Fujii M, Calderwood SK. Cancer extracellular vesicles, tumoroid models, and tumor microenvironment. Semin Cancer Biol 2022;86:112-26.
60. Hill BS, Sarnella A, D’Avino G, Zannetti A. Recruitment of stromal cells into tumour microenvironment promote the metastatic spread of breast cancer. Semin Cancer Biol 2020;60:202-13.
61. Liu Y, Gu Y, Han Y, et al. Tumor exosomal RNAs promote lung pre-metastatic niche formation by activating alveolar epithelial TLR3 to recruit neutrophils. Cancer Cell 2016;30:243-56.
62. Baxevanis CN, Fortis SP, Perez SA. The balance between breast cancer and the immune system: challenges for prognosis and clinical benefit from immunotherapies. Semin Cancer Biol 2021;72:76-89.
63. Wang X, Huang J, Chen W, Li G, Li Z, Lei J. The updated role of exosomal proteins in the diagnosis, prognosis, and treatment of cancer. Exp Mol Med 2022;54:1390-400.
64. Li Y, Liang Y, Sang Y, et al. MiR-770 suppresses the chemo-resistance and metastasis of triple negative breast cancer via direct targeting of STMN1. Cell Death Dis 2018;9:14.
65. Papi A, De Carolis S, Bertoni S, et al. PPARγ and RXR ligands disrupt the inflammatory cross-talk in the hypoxic breast cancer stem cells niche. J Cell Physiol 2014;229:1595-606.
66. Jung WH, Yam N, Chen CC, Elawad K, Hu B, Chen Y. Force-dependent extracellular matrix remodeling by early-stage cancer cells alters diffusion and induces carcinoma-associated fibroblasts. Biomaterials 2020;234:119756.
67. Song YH, Warncke C, Choi SJ, et al. Breast cancer-derived extracellular vesicles stimulate myofibroblast differentiation and pro-angiogenic behavior of adipose stem cells. Matrix Biol 2017;60-1:190-205.
68. González-Callejo P, Gener P, Díaz-Riascos ZV, et al. Extracellular vesicles secreted by triple-negative breast cancer stem cells trigger premetastatic niche remodeling and metastatic growth in the lungs. Int J Cancer 2023;152:2153-65.
69. Eckert RL, Kaartinen MT, Nurminskaya M, et al. Transglutaminase regulation of cell function. Physiol Rev 2014;94:383-417.
70. Antonyak MA, Li B, Boroughs LK, et al. Cancer cell-derived microvesicles induce transformation by transferring tissue transglutaminase and fibronectin to recipient cells. Proc Natl Acad Sci USA 2011;108:4852-7.
71. Schwager SC, Young KM, Hapach LA, et al. Weakly migratory metastatic breast cancer cells activate fibroblasts via microvesicle-Tg2 to facilitate dissemination and metastasis. Elife 2022;11:e74433.
72. Martínez-García D, Manero-Rupérez N, Quesada R, Korrodi-Gregório L, Soto-Cerrato V. Therapeutic strategies involving survivin inhibition in cancer. Med Res Rev 2019;39:887-909.
74. Li K, Liu T, Chen J, Ni H, Li W. Survivin in breast cancer-derived exosomes activates fibroblasts by up-regulating SOD1, whose feedback promotes cancer proliferation and metastasis. J Biol Chem 2020;295:13737-52.
75. D’Souza W, Kumar A. microRNAs in oral cancer: moving from bench to bed as next generation medicine. Oral Oncol 2020;111:104916.
76. Lee RC, Feinbaum RL, Ambros V. The C. elegans heterochronic gene lin-4 encodes small RNAs with antisense complementarity to lin-14. Cell 1993;75:843-54.
77. Pritchard CC, Cheng HH, Tewari M. MicroRNA profiling: approaches and considerations. Nat Rev Genet 2012;13:358-69.
78. Hamam R, Hamam D, Alsaleh KA, et al. Circulating microRNAs in breast cancer: novel diagnostic and prognostic biomarkers. Cell Death Dis 2017;8:e3045.
80. Krol J, Loedige I, Filipowicz W. The widespread regulation of microRNA biogenesis, function and decay. Nat Rev Genet 2010;11:597-610.
81. Nishihara T, Zekri L, Braun JE, Izaurralde E. miRISC recruits decapping factors to miRNA targets to enhance their degradation. Nucleic Acids Res 2013;41:8692-705.
82. Thomson DW, Bracken CP, Goodall GJ. Experimental strategies for microRNA target identification. Nucleic Acids Res 2011;39:6845-53.
83. Ren Z, Lv M, Yu Q, Bao J, Lou K, Li X. MicroRNA-370-3p shuttled by breast cancer cell-derived extracellular vesicles induces fibroblast activation through the CYLD/Nf-κB axis to promote breast cancer progression. FASEB J 2021;35:e21383.
84. Baroni S, Romero-Cordoba S, Plantamura I, et al. Exosome-mediated delivery of miR-9 induces cancer-associated fibroblast-like properties in human breast fibroblasts. Cell Death Dis 2016;7:e2312.
85. Ahn S, Kwon A, Huh YH, Rhee S, Song WK. Tumor-derived miR-130b-3p induces cancer-associated fibroblast activation by targeting SPIN90 in luminal A breast cancer. Oncogenesis 2022;11:47.
86. Yang SS, Ma S, Dou H, et al. Breast cancer-derived exosomes regulate cell invasion and metastasis in breast cancer via miR-146a to activate cancer associated fibroblasts in tumor microenvironment. Exp Cell Res 2020;391:111983.
87. Vu LT, Peng B, Zhang DX, et al. Tumor-secreted extracellular vesicles promote the activation of cancer-associated fibroblasts via the transfer of microRNA-125b. J Extracell Vesicles 2019;8:1599680.
88. Fong MY, Yan W, Ghassemian M, et al. Cancer-secreted miRNAs regulate amino-acid-induced mTORC1 signaling and fibroblast protein synthesis. EMBO Rep 2021;22:e51239.
89. Yan W, Wu X, Zhou W, et al. Cancer-cell-secreted exosomal miR-105 promotes tumour growth through the MYC-dependent metabolic reprogramming of stromal cells. Nat Cell Biol 2018;20:597-609.
90. Scognamiglio I, Cocca L, Puoti I, et al. Exosomal microRNAs synergistically trigger stromal fibroblasts in breast cancer. Mol Ther Nucleic Acids 2022;28:17-31.
91. Tang S, Yang L, Tang X, Liu M. The role of oxidized ATM in the regulation of oxidative stress-induced energy metabolism reprogramming of CAFs. Cancer Lett 2014;353:133-44.
92. Sung JS, Kang CW, Kang S, et al. ITGB4-mediated metabolic reprogramming of cancer-associated fibroblasts. Oncogene 2020;39:664-76.
93. Fong MY, Zhou W, Liu L, et al. Breast-cancer-secreted miR-122 reprograms glucose metabolism in premetastatic niche to promote metastasis. Nat Cell Biol 2015;17:183-94.
94. Mátyási B, Petővári G, Dankó T, et al. Extracellular vesicle-mediated metastasis suppressors NME1 and NME2 modify lipid metabolism in fibroblasts. Cancers 2022;14:3913.
95. Piersma B, Hayward MK, Weaver VM. Fibrosis and cancer: a strained relationship. Biochim Biophys Acta Rev Cancer 2020;1873:188356.
96. Zhang Y, Fang Z, Pan D, et al. Dendritic polymer-based nanomedicines remodel the tumor stroma: improve drug penetration and enhance antitumor immune response. Adv Mater 2024;36:e2401304.
97. Luga V, Zhang L, Viloria-Petit AM, et al. Exosomes mediate stromal mobilization of autocrine Wnt-PCP signaling in breast cancer cell migration. Cell 2012;151:1542-56.
98. Chen Y, Zeng C, Zhan Y, Wang H, Jiang X, Li W. Aberrant low expression of p85α in stromal fibroblasts promotes breast cancer cell metastasis through exosome-mediated paracrine Wnt10b. Oncogene 2017;36:4692-705.
99. Xi L, Peng M, Liu S, et al. Hypoxia-stimulated ATM activation regulates autophagy-associated exosome release from cancer-associated fibroblasts to promote cancer cell invasion. J Extracell Vesicles 2021;10:e12146.
100. Shimoda M, Principe S, Jackson HW, et al. Loss of the timp gene family is sufficient for the acquisition of the CAF-like cell state. Nat Cell Biol 2014;16:889-901.
101. Kim JE, Kim BG, Jang Y, Kang S, Lee JH, Cho NH. The stromal loss of miR-4516 promotes the FOSL1-dependent proliferation and malignancy of triple negative breast cancer. Cancer Lett 2020;469:256-65.
102. Orso F, Virga F, Dettori D, et al. Stroma-derived miR-214 coordinates tumor dissemination. J Exp Clin Cancer Res 2023;42:20.
103. Wang H, Wei H, Wang J, Li L, Chen A, Li Z. MicroRNA-181d-5p-containing exosomes derived from CAFs promote EMT by regulating CDX2/HOXA5 in breast cancer. Mol Ther Nucleic Acids 2020;19:654-67.
104. Tao S, Li H, Ma X, et al. Elevating microRNA-1-3p shuttled by cancer-associated fibroblasts-derived extracellular vesicles suppresses breast cancer progression and metastasis by inhibiting GLIS1. Cancer Gene Ther 2021;28:634-48.
105. Wu HJ, Hao M, Yeo SK, Guan JL. FAK signaling in cancer-associated fibroblasts promotes breast cancer cell migration and metastasis by exosomal miRNAs-mediated intercellular communication. Oncogene 2020;39:2539-49.
106. Chen B, Sang Y, Song X, et al. Exosomal miR-500a-5p derived from cancer-associated fibroblasts promotes breast cancer cell proliferation and metastasis through targeting USP28. Theranostics 2021;11:3932-47.
107. Liu Y, Yang Y, Du J, Lin D, Li F. MiR-3613-3p from carcinoma-associated fibroblasts exosomes promoted breast cancer cell proliferation and metastasis by regulating SOCS2 expression. IUBMB Life 2020;72:1705-14.
108. Dou D, Ren X, Han M, et al. Cancer-associated fibroblasts-derived exosomes suppress immune cell function in breast cancer via the miR-92/PD-L1 pathway. Front Immunol 2020;11:2026.
109. Sheng Z, Wang X, Ding X, et al. Exosomal miRNA-92a derived from cancer-associated fibroblasts promote invasion and metastasis in breast cancer by regulating G3BP2. Cell Signal 2024;119:111182.
110. Zhou WY, Cai ZR, Liu J, Wang DS, Ju HQ, Xu RH. Circular RNA: metabolism, functions and interactions with proteins. Mol Cancer 2020;19:172.
111. Chen T, Wang X, Li C, et al. CircHIF1A regulated by FUS accelerates triple-negative breast cancer progression by modulating NFIB expression and translocation. Oncogene 2021;40:2756-71.
112. Wang X, Chen T, Li C, et al. CircRNA-CREIT inhibits stress granule assembly and overcomes doxorubicin resistance in TNBC by destabilizing PKR. J Hematol Oncol 2022;15:122.
113. Ye F, Liang Y, Wang Y, et al. Cancer-associated fibroblasts facilitate breast cancer progression through exosomal circTBPL1-mediated intercellular communication. Cell Death Dis 2023;14:471.
114. Hauser S, Bacher G, Dobberstein B, Lütcke H. A complex of the signal sequence binding protein and the SRP RNA promotes translocation of nascent proteins. EMBO J 1995;14:5485-93.
115. Grotwinkel JT, Wild K, Segnitz B, Sinning I. SRP RNA remodeling by SRP68 explains its role in protein translocation. Science 2014;344:101-4.
116. Nabet BY, Qiu Y, Shabason JE, et al. Exosome RNA unshielding couples stromal activation to pattern recognition receptor signaling in cancer. Cell 2017;170:352-66.e13.
117. Young CD, Anderson SM. Sugar and fat - that’s where it’s at: metabolic changes in tumors. Breast Cancer Res 2008;10:202.
118. You M, Xie Z, Zhang N, et al. Signaling pathways in cancer metabolism: mechanisms and therapeutic targets. Signal Transduct Target Ther 2023;8:196.
119. Kansara S, Singh A, Badal AK, et al. The emerging regulatory roles of non-coding RNAs associated with glucose metabolism in breast cancer. Semin Cancer Biol 2023;95:1-12.
120. Dias AS, Almeida CR, Helguero LA, Duarte IF. Metabolic crosstalk in the breast cancer microenvironment. Eur J Cancer 2019;121:154-71.
121. Liu Y, Hua F, Zhan Y, et al. Carcinoma associated fibroblasts small extracellular vesicles with low miR-7641 promotes breast cancer stemness and glycolysis by HIF-1α. Cell Death Discov 2021;7:176.
122. Thomson DW, Dinger ME. Endogenous microRNA sponges: evidence and controversy. Nat Rev Genet 2016;17:272-83.
123. Li Y, Zhao Z, Liu W, Li X. SNHG3 functions as miRNA sponge to promote breast cancer cells growth through the metabolic reprogramming. Appl Biochem Biotechnol 2020;191:1084-99.
124. Mecca M, Sichetti M, Giuseffi M, et al. Synergic role of dietary bioactive compounds in breast cancer chemoprevention and combination therapies. Nutrients 2024;16:1883.
125. Sun WL, Chen J, Wang YP, Zheng H. Autophagy protects breast cancer cells from epirubicin-induced apoptosis and facilitates epirubicin-resistance development. Autophagy 2011;7:1035-44.
126. Qiu Y, Wang X, Sun Y, et al. TCF12 regulates exosome release from epirubicin-treated CAFs to promote ER+ breast cancer cell chemoresistance. Biochim Biophys Acta Mol Basis Dis 2023;1869:166727.
127. Chowdhury P, Nagesh PKB, Hatami E, et al. Tannic acid-inspired paclitaxel nanoparticles for enhanced anticancer effects in breast cancer cells. J Colloid Interface Sci 2019;535:133-48.
128. Tao S, Wang J, Li F, et al. Extracellular vesicles released by hypoxia-induced tumor-associated fibroblasts impart chemoresistance to breast cancer cells via long noncoding RNA H19 delivery. FASEB J 2024;38:e23165.
130. Clark GC, Hampton JD, Koblinski JE, et al. Radiation induces ESCRT pathway dependent CD44v3+ extracellular vesicle production stimulating pro-tumor fibroblast activity in breast cancer. Front Oncol 2022;12:913656.
131. Zelnak AB, O’Regan RM. Optimizing endocrine therapy for breast cancer. J Natl Compr Canc Netw 2015;13:e56-64.
132. Shah SH, Miller P, Garcia-Contreras M, et al. Hierarchical paracrine interaction of breast cancer associated fibroblasts with cancer cells via hMAPK-microRNAs to drive ER-negative breast cancer phenotype. Cancer Biol Ther 2015;16:1671-81.
133. Sansone P, Berishaj M, Rajasekhar VK, et al. Evolution of cancer stem-like cells in endocrine-resistant metastatic breast cancers is mediated by stromal microvesicles. Cancer Res 2017;77:1927-41.
134. Gao Y, Li X, Zeng C, et al. CD63+ cancer-associated fibroblasts confer tamoxifen resistance to breast cancer cells through exosomal miR-22. Adv Sci 2020;7:2002518.
135. Sansone P, Savini C, Kurelac I, et al. Packaging and transfer of mitochondrial DNA via exosomes regulate escape from dormancy in hormonal therapy-resistant breast cancer. Proc Natl Acad Sci USA 2017;114:E9066-75.
136. Chapman NM, Chi H. Metabolic adaptation of lymphocytes in immunity and disease. Immunity 2022;55:14-30.
137. Lin Z, Hua G, Hu X. Lipid metabolism associated crosstalk: the bidirectional interaction between cancer cells and immune/stromal cells within the tumor microenvironment for prognostic insight. Cancer Cell Int 2024;24:295.
138. Lundholm M, Schröder M, Nagaeva O, et al. Prostate tumor-derived exosomes down-regulate NKG2D expression on natural killer cells and CD8+ T cells: mechanism of immune evasion. PLoS One 2014;9:e108925.
139. Wang Y, Goliwas KF, Severino PE, et al. Mechanical strain induces phenotypic changes in breast cancer cells and promotes immunosuppression in the tumor microenvironment. Lab Invest 2020;100:1503-16.
140. Xie F, Zhou X, Su P, et al. Breast cancer cell-derived extracellular vesicles promote CD8+ T cell exhaustion via TGF-β type II receptor signaling. Nat Commun 2022;13:4461.
141. Cao J, Lv G, Wei F. Engineering exosomes to reshape the immune microenvironment in breast cancer: molecular insights and therapeutic opportunities. Clin Transl Med 2024;14:e1645.
142. Yao H, Huang C, Zou J, et al. Extracellular vesicle-packaged lncRNA from cancer-associated fibroblasts promotes immune evasion by downregulating HLA-A in pancreatic cancer. J Extracell Vesicles 2024;13:e12484.
143. Shang H, Lu L, Fan M, Lu Y, Shi X, Lu H. Exosomal circHIF1A derived from hypoxic-induced carcinoma-associated fibroblasts promotes hepatocellular carcinoma cell malignant phenotypes and immune escape. Int Immunopharmacol 2024;138:112282.
144. Liao D, Luo Y, Markowitz D, Xiang R, Reisfeld RA. Cancer associated fibroblasts promote tumor growth and metastasis by modulating the tumor immune microenvironment in a 4T1 murine breast cancer model. PLoS One 2009;4:e7965.
145. Costa A, Kieffer Y, Scholer-Dahirel A, et al. Fibroblast heterogeneity and immunosuppressive environment in human breast cancer. Cancer Cell 2018;33:463-79.e10.
146. Shen L, Huang H, Wei Z, et al. Integrated transcriptomics, proteomics, and functional analysis to characterize the tissue-specific small extracellular vesicle network of breast cancer. MedComm 2023;4:e433.
147. Yan Q, Deng Y, Zhang Q. A comprehensive overview of metaplastic breast cancer: features and treatments. Cancer Sci 2024;115:2506-14.
148. Chen X, Song E. Turning foes to friends: targeting cancer-associated fibroblasts. Nat Rev Drug Discov 2019;18:99-115.
149. Li W, Wang X, Li C, Chen T, Yang Q. Exosomal non-coding RNAs: emerging roles in bilateral communication between cancer cells and macrophages. Mol Ther 2022;30:1036-53.
150. Calon A, Tauriello DV, Batlle E. TGF-beta in CAF-mediated tumor growth and metastasis. Semin Cancer Biol 2014;25:15-22.
151. Turner N, Grose R. Fibroblast growth factor signalling: from development to cancer. Nat Rev Cancer 2010;10:116-29.
152. Suh J, Kim DH, Kim SJ, et al. Nuclear localization of fibroblast growth factor receptor 1 in breast cancer cells interacting with cancer associated fibroblasts. J Cancer Prev 2022;27:68-76.
153. Tomlinson DC, Knowles MA, Speirs V. Mechanisms of FGFR3 actions in endocrine resistant breast cancer. Int J Cancer 2012;130:2857-66.
154. Liu C, Wang Y, Li L, et al. Engineered extracellular vesicles and their mimetics for cancer immunotherapy. J Control Release 2022;349:679-98.
155. Wu K, Xing F, Wu SY, Watabe K. Extracellular vesicles as emerging targets in cancer: recent development from bench to bedside. Biochim Biophys Acta Rev Cancer 2017;1868:538-63.
156. Bister N, Pistono C, Huremagic B, Jolkkonen J, Giugno R, Malm T. Hypoxia and extracellular vesicles: a review on methods, vesicular cargo and functions. J Extracell Vesicles 2020;10:e12002.
157. Wu F, Yang J, Liu J, et al. Signaling pathways in cancer-associated fibroblasts and targeted therapy for cancer. Signal Transduct Target Ther 2021;6:218.
158. Wong VW, Rustad KC, Akaishi S, et al. Focal adhesion kinase links mechanical force to skin fibrosis via inflammatory signaling. Nat Med 2011;18:148-52.
160. Quail DF, Joyce JA. Microenvironmental regulation of tumor progression and metastasis. Nat Med 2013;19:1423-37.
161. Zhang DX, Dang XTT, Vu LT, et al. αvβ1 integrin is enriched in extracellular vesicles of metastatic breast cancer cells: A mechanism mediated by galectin-3. J Extracell Vesicles 2022;11:e12234.
162. Xu Z, Zeng S, Gong Z, Yan Y. Exosome-based immunotherapy: a promising approach for cancer treatment. Mol Cancer 2020;19:160.
163. Logozzi M, Di Raimo R, Mizzoni D, Fais S. What we know on the potential use of exosomes for nanodelivery. Semin Cancer Biol 2022;86:13-25.
164. Zhang M, Hu S, Liu L, et al. Engineered exosomes from different sources for cancer-targeted therapy. Signal Transduct Target Ther 2023;8:124.
Cite This Article
How to Cite
Download Citation
Export Citation File:
Type of Import
Tips on Downloading Citation
Citation Manager File Format
Type of Import
Direct Import: When the Direct Import option is selected (the default state), a dialogue box will give you the option to Save or Open the downloaded citation data. Choosing Open will either launch your citation manager or give you a choice of applications with which to use the metadata. The Save option saves the file locally for later use.
Indirect Import: When the Indirect Import option is selected, the metadata is displayed and may be copied and pasted as needed.
About This Article
Copyright
Data & Comments
Data
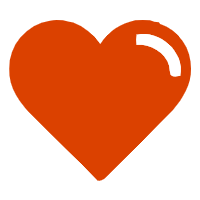
Comments
Comments must be written in English. Spam, offensive content, impersonation, and private information will not be permitted. If any comment is reported and identified as inappropriate content by OAE staff, the comment will be removed without notice. If you have any queries or need any help, please contact us at [email protected].