Future of autologous breast reconstruction: a review of novel technological innovations
Abstract
The evolution of autologous breast reconstruction is marked by significant technological advancements aimed at enhancing surgical outcomes. This review explores the current limitations and inherent challenges in standard practices of autologous breast reconstruction and highlights the potential benefits of the latest technological innovations. It addresses key aspects that stand to gain from improvements in surgical training and perioperative patient care, with a particular focus on preoperative planning, intraoperative techniques, and postoperative monitoring. The transformative potential of these technologies is poised to significantly improve patient outcomes, optimize surgical efficiency, and advance surgical education.
Keywords
INTRODUCTION
Breast cancer is the most prevalent malignancy among women and remains the leading cause of
Among the various reconstruction techniques, autologous breast reconstruction is frequently preferred by patients, owing to its natural appearance without the use of prosthesis[4]. Autologous breast reconstruction is especially appreciated for its advantageous use in adjuvant radiation therapy and its ability to better match the contralateral native breast in unilateral reconstructions. This approach is also often preferred over implant-based reconstruction due to a better complication profile, including less capsular contracture, infection, and the potential future need for implant replacement[5].
Despite its benefits, however, autologous reconstruction is not without limitations: Autologous breast reconstruction-commonly performed with a deep inferior epigastric artery perforator (DIEP) flap-is a technically difficult and lengthy procedure associated with the risk of complications, such as hematoma, skin necrosis, and notably, donor site morbidity. Currently, there has been a trend toward
Recent technological advancements demonstrate remarkable applications in three crucial aspects of perioperative in autologous breast reconstruction, namely: (i) preoperative planning; (ii) intraoperative technique; and (iii) postoperative monitoring. Concurrently, the evolution of surgical techniques demands a corresponding adaptation in educational methods to address increasing surgical complexity. This article aims to review and discuss the latest advances in technology and their application in autologous breast reconstruction, highlighting recent developments and their utility in perioperative management and surgical education [Figure 1].
PREOPERATIVE PLANNING
Background
Autologous breast reconstruction most frequently utilizes abdominally-based flaps, such as the aforementioned DIEP flap. Variations in perforator anatomy increases the complexity of these procedures, increasing operative time as well as the risk of intraoperative errors and postoperative complications[9]. Preoperative evaluation of flap and perforator selection is, therefore, key to optimizing patient outcomes and efficiency in the operating room.
Inadequate perforator selection can lead to postoperative complications such as hematoma, thrombosis, fat necrosis, and complete flap loss. Current methods of preoperative evaluation herald MRI angiography (MRA), CTA, and Doppler ultrasound as the gold standard. However, postoperative complications still occur relatively frequently even with the use of these preoperative imaging modalities[7]. In addition, the use of standard preoperative evaluation methods results in changes in surgical plan intraoperatively in nearly 25% of cases, which prolongs operative time, increasing the risk to the patient and financial burden[10]. Recent advancements in 3D printing, augmented reality, and artificial intelligence have been applied to autologous breast reconstruction to address these limitations to improve complication risk and optimize efficiency in the operating room[11-13].
3D printing
While various preoperative techniques like CTA, MRA, and Doppler ultrasound are available for flap perforator mapping, CTA with 3D reconstruction remains the most commonly used[9]. Preoperative imaging has the advantage of meticulous perforator selection prior to incision, which can reduce flap harvesting and operative times while lowering complication rates. However, the utility of CTA 3D reconstructed imaging is somewhat limited as it is typically visualized on a two-dimensional monitor[9]. Recent advancements aim to address this limitation by enhancing the visualization of perforators in
Patient-specific 3D-printed models have shown potential in improving visuo-spatial understanding of flap anatomy. A study by DeFazio et al. compared 3D-printed models [Figure 2A] and interpretations of CTA imaging against operative findings. 3D-printed models accurately matched intraoperative findings in perforator number, source vessel origin, and branching patterns, while CTA interpretations were less accurate[9]. The precision of 3D-printed models, with a marginal error averaging less than 1 mm compared to over 8 mm in CTA, led to a 20 min reduction in flap harvesting time[9]. Another prospective study assessed the use of 3D-printed flap templates in DIEP and MS-TRAM flap breast reconstructions. These templates, designed with perforator location indicators, proved as accurate as conventional methods, and significantly reduced intraoperative perforator identification time by over 7 min[11].
In a retrospective study, Ogunleye et al. compared 3D-printed models with CTA scans for preoperative planning, reinforcing the notion that 3D models can shorten harvesting time and decrease intraoperative adjustments[10]. While no significant differences in clinical outcomes were detected due to inadequate power, reconstructions using 3D-printed models showed lower rates of flap take-back[10]. However, the impact of 3D-printed flap models on clinical outcomes is not well-documented and may be subject to bias due to the challenges in conducting blinded studies.
Despite their benefits, 3D-printed models for preoperative planning are not without limitations, including cost, the use of a single material, and the exclusion of certain anatomical structures in earlier models[9-11]. A proof-of-concept study by Jacobson et al. addressed this by combining voxel-based and mesh-based modeling to create a more complex and accurate 3D-printed model of DIEP flaps, incorporating relevant anatomical structures such as the rectus abdominis muscle, intra- and extra-muscular vessels, and a geometric grid oriented around the umbilicus for anatomic localization of perforators[14]. This hybrid model, which accurately represented perforator locations compared to intraoperative findings, provided insights that often differed from those based on interpretations of CTA imaging[14].
In summary, 3D-printed models offer significant added advantages over traditional preoperative imaging in autologous breast reconstruction. However, their application is still in its nascent stages and has not yet been widely adopted. Future research, including large-scale studies and cost analyses, is essential to establish these techniques as a standard of care. Furthermore, the utility of anatomically complete 3D-printed DIEP flaps in clinical practice remains an unexplored area[14,15].
Augmented reality
AR is a novel and expanding technology that overlays digital information onto the real-world environment. Applications of AR are becoming increasingly popular in surgery as they can improve the visualization of anatomical structures by superimposing radiographic imaging onto the patient in real time. AR also circumvents difficulties associated with 3D printing modalities and their need to be extrapolated to the patient on the table. AR’s direct perioperative applicability by surgeons marks a significant technological advancement.
To effectively integrate AR into clinical practice, its virtual projections must closely align with the patient's anatomy. Cholok et al. validated the spatial congruence of AR projections with 3D-printed anatomical models using the Microsoft HoloLens (Redmond, WA) technology[16]. While they observed minor statistical differences in translational displacement and axial rotation between the 3D models and virtual projections, the overall scale of projection was accurate. This suggests that AR can reliably localize complex,
Provided that AR projections can match spatial fidelity with patient anatomy, it is essential to determine their feasibility and practicability in clinical practice. In a Danish case series involving five patients, AR was used preoperatively to superimpose virtual projections over patients’ abdomen, identifying and mapping perforators in comparison to ultrasound localization[12]. Using the HoloLens, these projections were then tagged, enalbing freedom of movement during the dissection. In four out of five cases, the point for which the DIEA perforated the anterior fascia matched perfectly with the virtual overlays. The time required for perforator tracking with AR was comparable to Doppler examinations, underscoring AR's potential utility in surgical planning[12].
Assessing AR's clinical utility, Hummelink et al. found that AR projections [Figure 2B] significantly reduced operative time and improved the accuracy of perforator identification compared to Doppler ultrasound[17]. The study reported a reduction of over 17 min for perforator mapping and 19 min in flap harvesting time in the AR group. Additionally, the AR model correctly identified 61.7% of perforators, compared to 41.2% in the Doppler group. However, the study lacked sufficient power to detect differences in complication rates[17].
Current research demonstrates the proof-of-concept and non-inferiority of AR-assisted perforator identification and selection in autologous breast reconstruction[12,16,17]. Future studies are imperative to further explore AR's impact on clinical outcomes and assess its cost-effectiveness for broader implementation in routine breast microsurgical practice.
Artificial intelligence
Artificial Intelligence (AI) in surgical planning is a rapidly evolving and promising technological advancement in breast reconstruction. One example of its powerful application is its use in preoperative imaging. Given that imaging analysis for perforator selection is both labor-intensive and time-consuming, integrating AI and machine learning processes such as convolutional or deep neural networks into this process presents a viable solution. This integration could enhance time and cost efficiency while minimizing observer bias and human error in image interpretation.
Traditionally, MRA and CTA analyses for perforator selection rely heavily on the expertise of technicians and radiologists. The incorporation of AI into this process could streamline preoperative planning and reduce the likelihood of error. A prospective study involving 40 patients undergoing DIEP flap reconstruction demonstrated that semi-automatic identification of perforators from CTA images, facilitated by computer vision AI and machine learning, saved approximately two h of preoperative planning time compared to manual analysis[18]. While the number of localized perforators was similar in both approaches, the AI technique showed reduced error in perforators larger than 1.5 mm in caliber. However, it also indicated a slight increase in error for smaller perforators, though these discrepancies were not clinically significant and did not impact surgical technique[18].
The utility of AI extends beyond perforator selection, offering the potential for automated, preemptive screening of patients for significant postoperative complications. Flap failure, while rare, is a significant complication of autologous breast reconstruction and is challenging to predict due to the absence of clear individual risk factors[19]. O’Neill et al. developed a multifactorial ML algorithm that stratifies various patient and treatment factors into risk categories for flap failure. Analyzing a retrospective cohort of 1,012 DIEP flap breast reconstructions, the model identified four high-risk subgroups based on BMI, comorbidities, and surgical history with high specificity for predicting flap failure[19]. While the study size was smaller than typically recommended for high predictive power in ML, resulting in lower-than-expected sensitivity, it lays the groundwork for future research in applications of AI[19]. This represents a proof-of-concept for an ML program that could screen high-risk patients for flap failure, which in turn could allow surgeons to
Looking ahead, with adequate preoperative and postoperative data, the combination of established methods with ML and computer vision AI could be harnessed to analyze the characteristics of perforators and associated complications[18,19]. This approach could enable the learning and selection of perforators that optimize clinical outcomes[13].
INTRAOPERATIVE INNOVATIONS
Background
There are various intraoperative targets for optimizing outcomes in breast microsurgical reconstruction, including advancing surgical techniques and improving methods for evaluating successful flap perfusion.
DIEP flap reconstruction has been associated with significant donor site morbidity, with potential complications such as abdominal wall herniation, chronic pain, and weakness. This is in part due to the large incision through the rectus fascia to dissect the vascular pedicle, which can potentially be minimized using minimally-invasive techniques[20]. Previous attempts employed technologies like the Da Vinci robotic system, but fell short due to lack of suitability for meticulous microsurgical procedures. As the realm of biotechnology expands, robotic platforms specific to microsurgery will enable optimization of techniques, providing new opportunities to improve breast reconstruction procedures[21,22].
Autologous breast reconstruction also carries the risk of fat necrosis and flap failure, which, despite recent advances, still remains high in up to 14% of patients[23]. To prevent these complications, flap viability must be accurately identified in the operating room. Currently, this is mainly accomplished using clinical judgment and subjective evaluation of the flap, such as color, temperature, capillary refill, and bleeding. While significant clinical experience confers some accuracy, clinical judgment alone falls short at times in identifying inadequate flap perfusion[24]. Recent technologies to be implemented for flap perfusion assessment include indocyanine green (ICG) fluorescence angiography and hyperspectral imaging (HSI)[21,25,26].
Robotic-assisted microsurgery
Robotic-assisted surgery, initially popularized in visceral and urological surgeries, has now made significant inroads into plastic surgery, including autologous breast reconstruction[27-29]. Early adaptation of robotic systems focused on their utility in harvesting free flaps. Roy et al. conducted a systematic review identifying two primary robotic-assisted breast reconstruction procedures using the Da Vinci Robotic system (Sunnyvale, California): a total of 240 DIEP and latissimus dorsi (LD) flap dissections were completed between 2006-2022. They observed that the total operative time for both DIEP and LD robotic-assisted procedures was, on average, 49 min longer than the reference data. However, the re-operation rate in robotic-assisted surgeries was significantly lower. Additionally, preliminary data indicated reduced pain at the recipient site and higher patient satisfaction scores (BREAST-Q tool) following robotic-assisted harvest[30]. Paralleling these findings, Khan et al. reviewed 56 robotic-assisted DIEP flap harvest procedures and noted decreased postoperative pain, shorter hospital stays, and improved patient-reported outcomes, albeit with increased operative times and costs[20]. Multiple separate case series have reported successful outcomes using the da Vinci Xi robot for robotic-assisted DIEP flaps with no flap failures or abdominal wall donor site morbidity postoperatively[31,32]. Robotic harvesting techniques are safe, reproducible, and feasible in a daily hospital setting and offer benefits such as improved visualization, increased dexterity, greater precision, and reduced surgeon fatigue due to better ergonomics[32]. However, conventional robotic systems like the Da Vinci system were not specifically designed for microsurgery, particularly microvascular anastomoses, as they are too large and robust, with suboptimal optics and magnification, and inability to scale movements precisely[33,34].
Addressing the need for more specialized technology in microsurgery, two main robotic systems have come onto the market: MicroSure’s MUSA (MicroSure, Eindhoven, Netherlands) [Figure 3A] and the MMI Symani robotic platform (Medical Microinstruments, Pisa, Italy) [Figure 3B]. In a landmark procedure by Innocenti et al. in 2021, the Symani platform was used for the first-in-human free flap tissue reconstruction[21]. Both systems are designed to stabilize and scale down movements while filtering tremors. Initially, microvascular anastomoses performed through robotic microsurgery were slower compared to traditional methods. However, they allowed for precise micro-movements with minimal tissue manipulation and made hard-to-reach anatomy more accessible[33]. Barbon et al. demonstrated that despite a steep learning curve, the time taken for robotic anastomosis eventually became comparable to that of hand-sewn anastomosis after several procedures[35]. A significant advantage of robotic microsurgery is the ability to scale motions down from 7-20 x, allowing the robot to function at a slower, more controlled speed[33]. In addition, more recent advances have coupled the use of the MUSA system with a 4K 3D exoscope, in place of a conventional microscope, allowing for improved visualization and ergonomics. This is made possible using 3D polarized glasses and high-definition screens stationed around the surgical field to allow the surgeon to operate with the robotic system without looking down through the oculars of a microscope. Van Mulken et al. demonstrated the feasibility of this system in microsurgical procedures and commended the ease and comfort of use, greatly improving the surgeon experience[36]. However, due to the novelty of these technologies, there is a dearth of high-quality, large-scale studies comparing robotic surgical outcomes to conventional manual techniques[22].
It is evident that robotic-assisted autologous breast reconstruction has shown significant promise in reducing complication rates and enhancing aesthetic outcomes, with minimal impact on anastomosis time. Such advancements suggest that this approach may become a mainstay surgical modality in the future.
Indocyanine green-aided imaging and hyperspectral imaging
Indocyanine green (ICG)-aided imaging, though present for over a decade, continues to represent an emerging innovation in autologous breast reconstruction. Utilizing the fluorescent properties of ICG dye, this imaging technique assists in visualizing blood flow and tissue perfusion in autologous free flaps and mastectomy skin during breast reconstruction[37]. ICG-aided imaging has also shown promise in enhancing lymph node biopsy accuracy in melanoma, aiding lymphedema management, and monitoring various microsurgical procedures[38,39]. Recent advancements have focused on optimizing ICG imaging parameters, particularly in the near-infrared window II (NIR-II, 1,000-1,700 nm), to enable deeper and more detailed perfusion analysis[40]. For example, Proulx et al. introduced a novel ICG formulation optimized for imaging outcomes, specifically targeting lymphatic vessels, which could facilitate concurrent lymphatic reconstruction and reduce lymphedema risk during autologous breast reconstruction[26]. Building upon these findings, Pruimboom et al. worked on optimizing factors for enhanced ICG use in flap surgery, including standardizing working distance, sensor angle, and pharmacology[24]. However, a limitation of ICG is that the identification of poorly perfused areas relies on subjective, qualitative evaluation of fluorescence intensity. Further advancements are geared toward quantifying the data, with several studies illustrating time-related parameters for predicting perfusion in free flaps following microvascular anastomosis and the quality of mastectomy skin flaps[41-43].
In addition to quantitative analysis, a recently described innovation integrates AI, specifically computer vision, into ICG perfusion studies. This specialized computer software is equipped with a video-tracking algorithm and designed to analyze ICG tissue data, offsetting variables such as user movements and patient artifacts, including respiration. The software captures and interprets various pixel ICG intensities over time and transforms this data to automatically label and characterize tissue quality[44,45]. This AI application could be further utilized in autologous breast reconstruction to assess perfusion and potentially detect complications. Additionally, a proof-of-concept study created a real-time continuous in vitro perfusion monitoring model with a high-frequency low-dose ICG regimen, potentially guiding clinical
With respect to hyperspectral imaging (HSI) system, this technology has advanced tissue visualization in various surgical procedures, such as oncological and transplant surgeries[47]. In broad terms, HSI captures a broad spectrum of light waves, providing a comprehensive tissue assessment beyond the standard red, green, and blue light waves. It can analyze tissue perfusion through parameters such as tissue oxygenation patterns, near-infrared, tissue hemoglobin, and water perfusion markers[48]. HSI offers an objective, reliable, and user-independent method to assess flap perfusion in autologous breast reconstructions[49]. Thiem et al. translated HSI into the field of microsurgery, conducting the first HSI-based measurements on free and pedicled flaps for head and neck reconstruction immediately after inset and postoperatively. Their findings suggested that HSI can accurately measure perfusion viability immediately after flap anastomosis, with specific cut-offs indicative of arterial/venous occlusion aiding decision-making on take-backs and intraoperative revisions[49]. Moreover, HSI detected signs of disturbed perfusion hours before clinical symptoms manifested, and in one case, HSI analysis showed clear signs of emergent venous congestion 36 h prior to clinical diagnosis[49]. In the context of autologous breast reconstruction, Pruimboom et al. demonstrated that HSI is superior in identifying postoperative mastectomy skin flap necrosis compared to conventional clinical evaluation in unilateral DIEP reconstruction[25].
Both HSI and ICG-aided imaging have significantly advanced as reliable intraoperative tools for autologous breast reconstructive surgery over the past decade. However, further research is necessary to establish standardized protocols that could potentially reduce operation time and cost. Such advancements highlight the evolving role of technology in enhancing surgical decision-making, traditionally reliant on clinical experience and judgment.
POSTOPERATIVE INNOVATIONS
Background
Postoperative monitoring of flap perfusion is just as crucial a stage in autologous breast reconstruction as it is intraoperatively. Complete flap loss is one of the most feared complications following this procedure, and flap viability hinges on the integrity of the vascular anastomosis and patency. As such, early identification of vascular compromise increases the likelihood of flap salvage[50].
Current methodologies rely on clinical evaluation of flap perfusion to identify flaps at risk for failure. This includes examining flap color, capillary refill, skin temperature, and tissue turgor, as well as handheld Doppler signals. However, much of this evaluation remains subjective, and its accuracy varies among different skin types, highlighting the role of a more objective evaluation method. In addition, clinical evaluation can be time-consuming, requiring frequent evaluations to allow for early intervention, resulting in substantial provider burden, especially in the immediate postoperative period[50].
Recent advances in the field of flap monitoring have been made to increase its objectivity and efficiency. This includes the development of remote patient monitoring platforms to decrease provider burden and the advancement of tissue oximetry technology to assess perfusion more accurately[50-52].
Remote patient monitoring platforms
Flap monitoring, especially critical within the first 72-h window, is a cornerstone of postoperative management in autologous reconstruction and has been strongly associated with the success of flap revisions[53]. Remote patient monitoring has emerged as a time-efficient and effective alternative to traditional in-person monitoring[50]. In the realm of autologous breast reconstruction, Hummelink et al. piloted a wireless monitoring sensor based on Imec’s Nightingale system (Eindhoven, the Netherlands) for DIEP flaps, featuring real-time data transmission capabilities to smartphones (although this function was disabled during the pilot study). The red and infrared sensors, equipped with two probes for placement on the flap and healthy skin tissue [Figure 4A], measured multiple parameters including temperature, tissue oxygenation, and delta muscle saturation every 90 seconds[51]. Similarly, Xie et al. developed an infrared wireless thermometer for flap perfusion monitoring, incorporating temperature and humidity modules along with a wireless component for remote monitoring. The device was able to recognize signs of decreased perfusion much earlier compared to conventional clinical techniques (30.5 vs. 41.7 h)[54]. Additionally, to counter the traditional cabled Cook Doppler (Bloomington, Indiana) monitoring device, Oda et al. created an implantable wireless and biodegradable sensor for real-time monitoring over the anastomosis site, lasting over a week. In a feasibility animal study in rats, the biosensor was implanted around the femoral artery, showing no significant differences in arterial lumen diameter, intima thickness, or tissue inflammation indicator compared to the control group. The sensor also detected changes in femoral arterial signals immediately following venous occlusions[55]. However, these promising results in animal models await validation in human trials.
Figure 4. Postoperative monitoring[17]. (A) Wireless monitoring sensor of tissue temperature, oxygenation, and delta muscle saturation; (B) Schematic of paintable phosphorescent bandage for assessing tissue oxygenation.
Remote patient monitoring presents significant potential to revolutionize postoperative monitoring strategies. Nevertheless, further research is essential to refine and standardize this innovative approach, ensuring its efficacy and reliability in clinical settings.
Advanced tissue oximetry technology
Tissue oxygenation has historically been identified as a key parameter for assessing postoperative flap perfusion[56]. While peripheral tissue oxygenation measurements have demonstrated poor accuracy when predicting flap failure, recent in vivo studies and human trials have introduced novel strategies to accurately measure and correlate tissue oximetry to tissue perfusion[52,57]. Currently, the most prevalent and reliable optical method for monitoring flap perfusion through tissue oximetry is the ViOptix (Fremont, Calif.) T. OX system, which utilizes near-infrared spectroscopy (NIRS). Nevertheless, this system's reliance on cabled leads connected to a control unit can be cumbersome, restrictive, and uncomfortable for patients[58]. These systems are also known to trigger false alarms due to many inevitable factors such as patient movement[59]. Addressing these challenges, the novel ViOptix Intra.Ox handheld device was developed for more accurate and portable assessments. Animal model studies demonstrated that this device could reliably identify at-risk tissues by correlating tissue oxygenation measurements with flap necrosis[52]. The Intra.ox device, with its handheld convenience and exclusion of intravenous dye injections, enables continuous perfusion assessments outside the operating room and proves to be more intuitive than thermography, especially beneficial in the perioperative period with fluctuating core body and ambient temperatures[52]. A further innovation in tissue oximetry, a paintable transparent phosphorescent bandage device [Figure 4B], has been developed and described to assess the partial oxygen pressure and perfusion in DIEP flaps[58]. This liquid bandage formulation contains novel metalloporphyrins structures, which detect light absorption and emission changes based on tissue oxygenation. These properties facilitate their integration into polymer matrices, enabling oxygen measurement in clinical settings with portable cameras. This permits
In summary, advancements in tissue oximetry show promise for enhancing postoperative monitoring in autologous breast reconstruction. Future studies are needed to fully understand the strengths and limitations of these innovative biotechnologies.
EDUCATION
Background
As autologous breast reconstruction techniques and technologies evolve, there is a pressing need for surgical training to adapt accordingly. Autologous breast reconstruction is a technically complex procedure requiring careful perforator dissection and precise vessel anastomosis within the confined chest anatomy. Traditional training methodologies encompass a range of approaches, including the Halsted apprenticeship model from the late 1,800s, didactic learning, instructional videos, and simulations using both living and non-living animal models[60,61].
While the apprenticeship model is deemed the hallmark of surgical training, it imposes a considerable time demand on faculty, and therefore faces practical challenges. These constraints are further heightened by the need to minimize experimental teaching, underscored by the importance of reducing anesthesia time, coupled with the inherently low margin of error in microsurgery. In autologous breast reconstruction, specific challenges include understanding the complex spatial and three-dimensional anatomy of the
Consequently, there is a role for surgical education to pivot toward other effective and efficient teaching strategies that can mirror the true complexity and subtleties of microsurgical techniques in breast reconstruction.
Three-Dimensional (3D) printing
The application of 3D printing in surgical education transcends operative planning and offers a significant educational advantage. Often, early-stage trainees struggle with the visuo-spatial skills required to interpret CT angiography (CTA) imaging in the context of a patient's three-dimensional anatomy for perforator dissection. In a pioneering effort, Mehta et al. utilized patient-specific 3D-printed models to replicate the rectus abdominis muscle and the intricate intramuscular course of DIEA perforators. This model was designed to assist trainees in evaluating patients’ three-dimensional surgical anatomy for effective application in the operating room[15]. Surgical residents were able to study the model preoperatively and intraoperatively to help with perforator dissection. Anecdotal evidence indicated that these 3D models enhanced clarity in dissection, offered superior visualization of the perforator’s course, and provided a clearer understanding of perforator depth in comparison to traditional CTA imaging[15]. This evidence is supported by the success of previous 3D models used for education in skull base anatomy, complex orbital anatomy, and even pathologic anatomy of craniofacial anomalies[62-64].
As trainees progress in their understanding of perforator anatomy, they require consistent and realistic methods for tactile practice outside the operating room. While the chicken thigh model is frequently used given its similar vessel caliber to that of humans, it falls short in mimicking the actual clinical environment and anatomical precision needed for successful technical execution[60]. For instance, during chest wall dissection and microvascular anastomosis, the depth at which the internal mammary vessels are located poses significant training challenges in accessing and visualizing the anastomosis site, a complexity not adequately represented by standard animal and simulation models. Papavisiliou et al. addressed this limitation by creating a 3D-printed chest wall that could be integrated with the chicken thigh model
3D printing provides surgical trainees with the opportunity to provide realistic hands-on surgical simulation much earlier in their career than the standard apprenticeship method alone. It also serves as an excellent tool for improving their visuo-spatial understanding. As 3D printing technology continues to evolve, its role and impact in surgical education are expected to further expand.
Augmented reality
Augmented reality (AR) technology has untapped potential as an educational tool in autologous breast reconstruction[65-68]. A notable example of AR's application in surgical education is the Microsoft HoloLens (Redmond, WA), which is becoming increasingly popular. In combination with specialized software, Cholok et al. had users visualize precise projections of patient soft tissue anatomy, including intra-muscular perforator artery course on corresponding 3D models, allowing trainees to engage in step-by-step simulated procedures and possibly guiding trainees in real time during the procedure[16,69]. Its anatomical accuracy in autologous breast reconstruction has been well documented, suggesting significant potential for trainee education with appropriately designed patient models and simulations[16].
Additionally, the integration of the HoloLens with Touch Surgery (Minneapolis, MN) [Figure 5B] - a free, device-based application featuring a comprehensive library of surgical procedures for interactive learning-is underway[69]. This integration aims to create a mixed reality platform that enables learning surgical procedures through programmed hand movements[69].
Despite the subjective endorsements by many plastic surgeons regarding the application of these technologies in clinical practice and education, their practical feasibility and utility require more objective assessment. A pilot study addressing this gap explored surgical trainee perspectives on AR's effectiveness in learning anatomy and surgical skills[61]. Remarkably, 78% of residents found holographic learning comparable to traditional apprenticeship-based methods, with a unanimous preference for AR over
Although the initial adoption of AR may pose a financial challenge, ongoing technological advancements and expected market price reductions could render it a viable training modality in the near future.
CONCLUSION
Autologous breast reconstruction is undergoing a transformative phase marked by significant technological advancements. These innovations span various aspects of the patient’s surgical experience, including preoperative planning, surgical techniques, and postoperative care. The integration of 3D printing has not only enhanced trainee education and preoperative planning but also paved the way for more precise and personalized surgical approaches. The incorporation of AR and AI into surgical decision-making will only revolutionize the field by providing more advanced and immediate analytical capabilities. Furthermore, the emergence of robotic-assisted microsurgery and cutting-edge imaging modalities like hyperspectral imaging is refining intraoperative techniques and capabilities, leading to greater surgical precision and hopefully enhanced safety. In the postoperative realm, the development of remote patient monitoring and advanced tissue oximetry technologies shows great promise in improving patient outcomes and elevating the quality of care while decreasing provider burden.
Collectively, these technological breakthroughs signify a paradigm shift toward more tailored, efficient, and effective methods in autologous breast reconstruction. These advancements aim to not only enhance patient care but also offer significant benefits to surgeons. As the field continues to advance, the ongoing research and clinical integration of these innovations will further refine their roles, solidifying their place in the future of autologous breast reconstruction surgery.
DECLARATIONS
Author contributions
Background research, writing, and editing: Allam O, Foster C, Knoedler L, Knoedler S, Oh SJ, Pomahac B, Ayyala HS
Availability of data and materials
Not applicable.
Financial support and sponsorship
The authors received no funding for data collection or preparation of the manuscript.
Conflicts of interest
All authors declare that there are no conflicts of interest.
Ethical approval and consent to participate
Not applicable.
Consent for publication
Not applicable.
Copyright
© The Author(s) 2024.
REFERENCES
1. Nolan E, Lindeman GJ, Visvader JE. Deciphering breast cancer: from biology to the clinic. Cell 2023;186:1708-28.
2. Nardin S, Mora E, Varughese FM, et al. Breast cancer survivorship, quality of life, and late toxicities. Front Oncol 2020;10:864.
3. Al-Ghazal SK, Fallowfield L, Blamey RW. Comparison of psychological aspects and patient satisfaction following breast conserving surgery, simple mastectomy and breast reconstruction. Eur J Cancer 2000;36:1938-43.
4. Yueh JH, Slavin SA, Adesiyun T, et al. Patient satisfaction in postmastectomy breast reconstruction: a comparative evaluation of DIEP, TRAM, latissimus flap, and implant techniques. Plast Reconstr Surg 2010;125:1585-95.
5. Yun JH, Diaz R, Orman AG. Breast reconstruction and radiation therapy. Cancer Control 2018;25:1073274818795489.
6. Pien I, Caccavale S, Cheung MC, et al. Evolving trends in autologous breast reconstruction: is the deep inferior epigastric artery perforator flap taking over? Ann Plast Surg 2016;76:489-93.
7. Wade RG, Razzano S, Sassoon EM, Haywood RM, Ali RS, Figus A. Complications in DIEP flap breast reconstruction after mastectomy for breast cancer: a prospective cohort study comparing unilateral versus bilateral reconstructions. Ann Surg Oncol 2017;24:1465-74.
8. Knoedler S, Kauke-Navarro M, Knoedler L, et al. The significance of timing in breast reconstruction after mastectomy: an ACS-NSQIP analysis. J Plast Reconstr Aesthet Surg 2024;89:40-50.
9. DeFazio MV, Arribas EM, Ahmad FI, et al. Application of three-dimensional printed vascular modeling as a perioperative guide to perforator mapping and pedicle dissection during abdominal flap harvest for breast reconstruction. J Reconstr Microsurg 2020;36:325-38.
10. Ogunleye AA, Deptula PL, Inchauste SM, et al. The utility of three-dimensional models in complex microsurgical reconstruction. Arch Plast Surg 2020;47:428-34.
11. Chae MP, Hunter-Smith DJ, Chung RD, Smith JA, Rozen WM. 3D-printed, patient-specific DIEP flap templates for preoperative planning in breast reconstruction: a prospective case series. Gland Surg 2021;10:2192-9.
12. Seth I, Lindhardt J, Jakobsen A, et al. Improving visualization of intramuscular perforator course: augmented reality headsets for DIEP flap breast reconstruction. Plast Reconstr Surg Glob Open 2023;11:e5282.
13. Cevik J, Seth I, Rozen WM. Transforming breast reconstruction: the pioneering role of artificial intelligence in preoperative planning. Gland Surg 2023;12:1271-5.
14. Jacobson NM, Carerra E, Treat A, McDonnell M, Mathes D, Kaoutzanis C. Hybrid modeling techniques for 3D printed deep inferior epigastric perforator flap models. 3D Print Med 2023;9:26.
15. Mehta S, Byrne N, Karunanithy N, Farhadi J. 3D printing provides unrivalled bespoke teaching tools for autologous free flap breast reconstruction. J Plast Reconstr Aesthet Surg 2016;69:578-80.
16. Cholok DJ, Fischer MJ, Leuze CW, Januszyk M, Daniel BL, Momeni A. Spatial fidelity of microvascular perforating vessels as perceived by augmented reality virtual projections. Plast Reconstr Surg 2024;153:524-34.
17. Hummelink S, Hoogeveen YL, Schultze Kool LJ, Ulrich DJO. A new and innovative method of preoperatively planning and projecting vascular anatomy in DIEP flap breast reconstruction: a randomized controlled trial. Plast Reconstr Surg 2019;143:1151e-8e.
18. Mavioso C, Araújo RJ, Oliveira HP, et al. Automatic detection of perforators for microsurgical reconstruction. Breast 2020;50:19-24.
19. O'Neill AC, Yang D, Roy M, Sebastiampillai S, Hofer SOP, Xu W. Development and evaluation of a machine learning prediction model for flap failure in microvascular breast reconstruction. Ann Surg Oncol 2020;27:3466-75.
20. Khan MTA, Won BW, Baumgardner K, et al. Literature review: robotic-assisted harvest of deep inferior epigastric flap for breast reconstruction. Ann Plast Surg 2022;89:703-8.
21. Innocenti M, Malzone G, Menichini G. First-in-human free flap tissue reconstruction using a dedicated microsurgical robotic platform. Plast Reconstr Surg 2023;151:1078-82.
22. Ghandourah HSH, Schols RM, Wolfs JAGN, Altaweel F, van Mulken TJM. Robotic microsurgery in plastic and reconstructive surgery: a literature review. Surg Innov 2023;30:607-14.
23. Bhullar H, Hunter-Smith DJ, Rozen WM. Fat necrosis after DIEP flap breast reconstruction: a review of perfusion-related causes. Aesthetic Plast Surg 2020;44:1454-61.
24. Pruimboom T, van Kuijk SMJ, Qiu SS, et al. Optimizing indocyanine green fluorescence angiography in reconstructive flap surgery: a systematic review and ex vivo experiments. Surg Innov 2020;27:103-19.
25. Pruimboom T, Lindelauf AAMA, Felli E, et al. Perioperative hyperspectral imaging to assess mastectomy skin flap and DIEP flap perfusion in immediate autologous breast reconstruction: a pilot study. Diagnostics 2022;12:184.
26. Proulx ST, Luciani P, Derzsi S, et al. Quantitative imaging of lymphatic function with liposomal indocyanine green. Cancer Res 2010;70:7053-62.
27. Prabhu AS, Carbonell A, Hope W, et al. Robotic inguinal vs transabdominal laparoscopic inguinal hernia repair: the RIVAL randomized clinical trial. JAMA Surg 2020;155:380-7.
28. Brassetti A, Ragusa A, Tedesco F, et al. Robotic surgery in urology: history from PROBOT® to HUGOTM. Sensors 2023;23:7104.
29. Aitzetmüller MM, Klietz ML, Dermietzel AF, Hirsch T, Kückelhaus M. Robotic-assisted microsurgery and its future in plastic surgery. J Clin Med 2022;11:3378.
30. Roy N, Alessandro CJ, Ibelli TJ, et al. The expanding utility of robotic-assisted flap harvest in autologous breast reconstruction: a systematic review. J Clin Med 2023;12:4951.
31. Daar DA, Anzai LM, Vranis NM, et al. Robotic deep inferior epigastric perforator flap harvest in breast reconstruction. Microsurgery 2022;42:319-25.
32. Wittesaele W, Vandevoort M. Implementing the robotic deep inferior epigastric perforator flap in daily practice: a series of 10 cases. J Plast Reconstr Aesthet Surg 2022;75:2577-83.
33. Besmens IS, Politikou O, Giovanoli P, Calcagni M, Lindenblatt N. Robotic microsurgery in extremity reconstruction - experience with a novel robotic system. Surg Innov 2024;31:42-7.
34. Wolfs JA, Schols RM, van Mulken TJ. Robotic microvascular and free flap surgery: overview of current robotic applications and introduction of a dedicated robot for microsurgery. In: Nikkhah, D., Rawlins, J., Pafitanis, G. (eds) Core Techniques in Flap Reconstructive Microsurgery. Springer, Cham; 2023.p.77-86.
35. Barbon C, Grünherz L, Uyulmaz S, Giovanoli P, Lindenblatt N. Exploring the learning curve of a new robotic microsurgical system for microsurgery. JPRAS Open 2022;34:126-33.
36. van Mulken TJM, Qiu SS, Jonis Y, et al. First-in-human integrated use of a dedicated microsurgical robot with a 4K 3D exoscope: the future of microsurgery. Life 2023;13:692.
37. Abdelrahman H, El-Menyar A, Peralta R, Al-Thani H. Application of indocyanine green in surgery: a review of current evidence and implementation in trauma patients. World J Gastrointest Surg 2023;15:757-75.
38. Burnier P, Niddam J, Bosc R, Hersant B, Meningaud JP. Indocyanine green applications in plastic surgery: a review of the literature. J Plast Reconstr Aesthet Surg 2017;70:814-27.
39. Bigdeli AK, Gazyakan E, Schmidt VJ, et al. Indocyanine green fluorescence for free-flap perfusion imaging revisited: advanced decision making by virtual perfusion reality in visionsense fusion imaging angiography. Surg Innov 2016;23:249-60.
40. Wu Y, Suo Y, Wang Z, et al. First clinical applications for the NIR-II imaging with ICG in microsurgery. Front Bioeng Biotechnol 2022;10:1042546.
41. Van Den Hoven P, Verduijn PS, Van Capelle L, et al. Quantification of near-infrared fluorescence imaging with indocyanine green in free flap breast reconstruction. J Plast Reconstr Aesthet Surg 2022;75:1820-5.
42. Moyer HR, Losken A. Predicting mastectomy skin flap necrosis with indocyanine green angiography: the gray area defined. Plast Reconstr Surg 2012;129:1043-8.
43. Phillips BT, Lanier ST, Conkling N, et al. Intraoperative perfusion techniques can accurately predict mastectomy skin flap necrosis in breast reconstruction: results of a prospective trial. Plast Reconstr Surg 2012;129:778e-88e.
44. Cahill RA, O'Shea DF, Khan MF, et al. Artificial intelligence indocyanine green (ICG) perfusion for colorectal cancer intra-operative tissue classification. Br J Surg 2021;108:5-9.
45. Poplack SP, Park EY, Ferrara KW. Optical breast imaging: a review of physical principles, technologies, and clinical applications. J Breast Imaging 2023;5:520-37.
46. Oppermann C, Dohrn N, Yikilmaz H, Falk Klein M, Eriksen T, Gögenur I. Continuous organ perfusion monitoring using indocyanine green in a piglet model. Surg Endosc 2023;37:1601-10.
47. Karim S, Qadir A, Farooq U, Shakir M, Laghari AA. Hyperspectral imaging: a review and trends towards medical imaging. Curr Med Imaging 2022;19:417-27.
48. Studier-Fischer A, Seidlitz S, Sellner J, et al. Spectral organ fingerprints for machine learning-based intraoperative tissue classification with hyperspectral imaging in a porcine model. Sci Rep 2022;12:11028.
49. Thiem DGE, Frick RW, Goetze E, Gielisch M, Al-Nawas B, Kämmerer PW. Hyperspectral analysis for perioperative perfusion monitoring-a clinical feasibility study on free and pedicled flaps. Clin Oral Investig 2021;25:933-45.
50. Knoedler S, Hoch CC, Huelsboemer L, et al. Postoperative free flap monitoring in reconstructive surgery-man or machine? Front Surg 2023;10:1130566.
51. Hummelink SLM, Paulus VAA, Wentink EC, Ulrich DJO. Development and evaluation of a remote patient monitoring system in autologous breast reconstruction. Plast Reconstr Surg Glob Open 2022;10:e4008.
52. Khavanin N, Darrach H, Kraenzlin F, Yesantharao PS, Sacks JM. The intra.Ox near-infrared spectrometer measures variations in flap oxygenation that correlate to flap necrosis in a preclinical rodent model. Plast Reconstr Surg 2021;147:1097-104.
53. Largo RD, Selber JC, Garvey PB, et al. Outcome analysis of free flap salvage in outpatients presenting with microvascular compromise. Plast Reconstr Surg 2018;141:20e-7e.
54. Xie R, Zhang Y, Liu Q, Huang X, Liu M. A wireless infrared thermometry device for postoperative flap monitoring: proof of concept in patients. Surg Innov 2023;30:636-9.
55. Oda H, Beker L, Kaizawa Y, et al. A novel technology for free flap monitoring: pilot study of a wireless, biodegradable sensor. J Reconstr Microsurg 2020;36:182-90.
56. Halani SH, Hembd AS, Li X, et al. Flap monitoring using transcutaneous oxygen or carbon dioxide measurements. J Hand Microsurg 2022;14:10-8.
57. Guye ML, Motamed C, Chemam S, Leymarie N, Suria S, Weil G. Remote peripheral tissue oxygenation does not predict postoperative free flap complications in complex head and neck cancer surgery: a prospective cohort study. Anaesth Crit Care Pain Med 2017;36:27-31.
58. Marks H, Bucknor A, Roussakis E, et al. A paintable phosphorescent bandage for postoperative tissue oxygen assessment in DIEP flap reconstruction. Sci Adv 2020;6:eabd1061.
59. Khanna AK, Ahuja S, Weller RS, Harwood TN. Postoperative ward monitoring - why and what now? Best Pract Res Clin Anaesthesiol 2019;33:229-45.
60. Papavasiliou T, Ubong S, Khajuria A, Chatzimichail S, Chan JCY. 3D printed chest wall: a tool for advanced microsurgical training simulating depth and limited view. Plast Reconstr Surg Glob Open 2021;9:e3817.
61. Leung R, Shi G. Building your future holographic mentor: can we use mixed reality holograms for visual spatial motor skills acquisition in surgical education? Surg Innov 2024;31:82-91.
62. Favier V, Zemiti N, Caravaca Mora O, et al. Geometric and mechanical evaluation of 3D-printing materials for skull base anatomical education and endoscopic surgery simulation - a first step to create reliable customized simulators. PLoS One 2017;12:e0189486.
63. Lichtenstein JT, Zeller AN, Lemound J, et al. 3D-printed simulation device for orbital surgery. J Surg Educ 2017;74:2-8.
64. Lobb DC, Cottler P, Dart D, Black JS. The use of patient-specific three-dimensional printed surgical models enhances plastic surgery resident education in craniofacial surgery. J Craniofac Surg 2019;30:339-41.
65. Smith DM, Aston SJ, Cutting CB, Oliker A. Applications of virtual reality in aesthetic surgery. Plast Reconstr Surg 2005;116:898-904; discussion 905-6.
66. Smith DM, Oliker A, Carter CR, Kirov M, McCarthy JG, Cutting CB. A virtual reality atlas of craniofacial anatomy. Plast Reconstr Surg 2007;120:1641-6.
67. Tolsdorff B, Pommert A, Höhne KH, et al. Virtual reality: a new paranasal sinus surgery simulator. Laryngoscope 2010;120:420-6.
68. de Runz A, Boccara D, Bertheuil N, Claudot F, Brix M, Simon E. Three-dimensional imaging, an important factor of decision in breast augmentation. Ann Chir Plast Esthet 2018;63:134-9.
Cite This Article
How to Cite
Allam, O.; Foster, C.; Knoedler, L.; Knoedler, S.; Oh, S. J.; Pomahac, B.; Ayyala, H. S. Future of autologous breast reconstruction: a review of novel technological innovations. Plast. Aesthet. Res. 2024, 11, 19. http://dx.doi.org/10.20517/2347-9264.2024.21
Download Citation
Export Citation File:
Type of Import
Tips on Downloading Citation
Citation Manager File Format
Type of Import
Direct Import: When the Direct Import option is selected (the default state), a dialogue box will give you the option to Save or Open the downloaded citation data. Choosing Open will either launch your citation manager or give you a choice of applications with which to use the metadata. The Save option saves the file locally for later use.
Indirect Import: When the Indirect Import option is selected, the metadata is displayed and may be copied and pasted as needed.
About This Article
Special Issue
Copyright
Data & Comments
Data
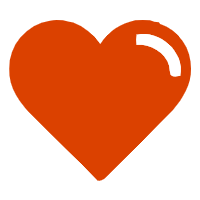
Comments
Comments must be written in English. Spam, offensive content, impersonation, and private information will not be permitted. If any comment is reported and identified as inappropriate content by OAE staff, the comment will be removed without notice. If you have any queries or need any help, please contact us at support@oaepublish.com.