Exploring new therapeutics for Duchenne muscular dystrophy and related cardiomyopathy
Abstract
Duchenne muscular dystrophy (DMD) is a severe and progressively debilitating X-linked recessive disorder caused by mutations in the DMD gene, which encodes the dystrophin protein. This deficiency in dystrophin results in the progressive degeneration of both skeletal and cardiac muscles. Currently, there is no definitive cure for DMD, and treatment primarily aims to slow disease progression and manage symptoms. With the widespread application of respiratory support measures, DMD cardiomyopathy has emerged as the primary contributor to morbidity and mortality among DMD patients at present. There is an acute and pressing need to develop highly effective therapeutic strategies for treating DMD cardiomyopathy and to prevent the onset of heart failure. Various hypotheses have been proposed to explain the underlying mechanisms, including elevated levels of inflammatory cytokines, dysregulated HDAC activity, disruptions in ion balance, and mitochondrial dysfunction, which is also considered a potentially significant contributor. This review article aims to provide a comprehensive overview of various animal and human induced pluripotent stem cell-derived cardiomyocytes (hiPSC-CMs) models on DMD and DMD cardiomyopathy. It also provides a summary of current advancements and ongoing efforts in the treatment of DMD and DMD-related cardiomyopathy, with a focus on innovative treatment modalities, such as mitochondria transplantation or targeting ion homeostasis. This underscores the dynamic and evolving nature of research dedicated to developing effective treatments for DMD and DMD cardiomyopathy.
Keywords
INTRODUCTION
Genetics and role of dystrophin
DMD is an inheritable X-linked recessive muscular disorder caused by mutations in the DMD gene. The DMD gene, encoding for the dystrophin protein, is currently the largest known human gene (2.4Mb), containing 79 exons, four functional domains, and a wide variety of mutations reported[1]. Approximately 79% of DMD mutations involve large deletions or duplications (one or more exons), while the remaining 21% consist of nonsense mutations (about 11%), small deletions (about 5%), small insertions (about 2%), and splice site mutations (about 3%). Globally, one in every 3,500 newborn male infants is affected by this disease. Female carriers generally do not develop the disease, but some may exhibit mild symptoms[2,3].
Dystrophin is a rod-shaped protein positioned beneath the muscle fiber membrane. Wild-type dystrophin comprises four primary functional domains: (i) an N-terminal domain that binds to F-actin; (ii) a substantial central rod domain, which contains an additional actin-binding region and an nNOS (neuronal nitric oxide synthase) binding site; (iii) a cysteine-rich domain responsible for binding dystroglycan; and (iv) a C-terminal domain. Dystrophin is a fundamental component of the dystrophin-glycoprotein complex (DGC), which bridges the intracellular cytoskeleton with the extracellular matrix, thereby offering structural reinforcement to the sarcolemma[4]. Beyond its structural role, dystrophin also acts as a scaffold for proteins involved in diverse signaling cascades, encompassing sarcolemmal ion channels. Specifically in cardiac and skeletal muscle, the Dp427 (Dystrophin protein of 427 kDa) isoform of dystrophin is expressed and located at both the sarcolemma and within the T-tubules[5,6]. In DMD patients, dystrophin deficiency leads to sarcolemma membrane fragility and susceptibility to contraction-induced damage[7]. This causes progressive destruction of muscle cells, resulting in progressive skeletal muscle atrophy, gradual muscle weakness, and ultimate loss of mobility[8,9].
Clinical manifestations and current standard of care for DMD
Dystrophin deficiency causes structural instability in both muscles and cardiomyocytes, resulting in cellular damage during daily contraction and relaxation. Clinically, DMD typically manifests in early childhood with symptoms such as delayed motor development, frequent falls, and difficulty in walking and running. As the disease progresses, patients experience progressive muscle weakness and atrophy, leading to loss of mobility and the need for assistance with daily activities. Eventually, DMD affects the respiratory and cardiac muscles, leading to respiratory and cardiac failure. In addition to muscle weakness and atrophy, DMD patients may also experience other symptoms such as contractures (tightening of muscles and joints), scoliosis (curvature of the spine), and intellectual impairment in some cases[10,11]. In-frame mutations of the DMD gene, diagnosed as Becker muscular dystrophy (BMD), result in the formation of truncated dystrophin protein that shows milder symptoms and later disease onset[12,13].
Corticosteroids combined with multidisciplinary care are commonly used to delay the loss of motor function in DMD patients, although this approach cannot alter the disease’s outcome. Corticosteroids such as prednisone and Emflaza (deflazacort) are widely used in the treatment of DMD. These medications can slow the progression of muscle weakness and delay the loss of mobility, but long-term use may stimulate mineralocorticoid receptors (MR), potentially leading to detrimental effects such as decreased bone density, obesity, hypertension, adrenal insufficiency, and heightened muscle catabolism[14]. Vamorolone is a structurally unique dissociative steroidal anti-inflammatory drug. It effectively reduces muscle inflammation in DMD patients while causing fewer adverse reactions compared to traditional glucocorticoids, providing a better treatment option for DMD[15].
Patients born with this disease experience progressive muscle loss leading to muscle weakness and, by their late 20s, succumb to dilated cardiomyopathy and respiratory failure. DMD cardiomyopathy is progressive and the present cardiac phenotypes include: arrhythmia, ECG abnormalities, diastolic dysfunction, fibrosis, gradual ventricular dilation, systolic dysfunction, and end-stage heart failure[11]. With the implementation of respiratory support, DMD cardiomyopathy is currently the main cause of morbidity and mortality in DMD patients. There is an urgent need to develop efficacious therapies for treating DMD cardiomyopathy and prevent the onset of heart failure.
In this review, we focus on the current animal and cellular models used in DMD research and aim to provide an overview of emerging treatments being explored for treating DMD and DMD cardiomyopathy.
MODELS OF DMD
Animal models of DMD play an irreplaceable role in the field of DMD research and many models have been developed. Besides the classic mdx mouse model, larger animal models (dog and pig models) have been developed to better mimic physiology and zebrafish and Caenorhabditis elegans models have been created to enable high-throughput screenings. Despite these efforts, challenges remain. Differences in lifespan, lack of heart failure phenotype, and mild muscle loss have limited our ability to translate laboratory findings into the clinic. Thus, it is our intent to review available models and drug designs in our fight against DMD and DMD cardiomyopathy.
Animal models
Mouse
The mdx4cv mouse is the most commonly used DMD model, displaying metabolic changes and mild cardiomyopathy, though it compensates for dystrophin deficiency with utrophin, resulting in a milder phenotype than seen in humans. To enhance clinical relevance, the mdx4cv/utrn-/- double knockout model[16,17] and humanized strains, such as hDMDdel45/mdx[18], have been developed, each showing stronger muscle degeneration phenotype that mimicked human pathology. Previously, a series of studies from Dr. Helen Blau’s group demonstrated that long murine telomeres mask cardiac phenotype in mdx4cv mice. Blau’s group demonstrated that humanized mouse telomere by cross breeding mTR-/- mouse, Terc knockout model, with mdx4cv mouse can better mimic DMD patients’ muscle phenotype[19] and heart failure[20,21]. Recently, a study performed a megabase-scale deletion of one of the duplicated DMD transgenes in Tg (DMD)72Thoen/J (hDMDTg) mouse[22]. Utilizing CRISPR zygotic microinjection, Chey et al. generated a single-copy, full-length, humanized DMD transgenic mouse model (hDMDTgSc), which simulates the haploid nature of the DMD gene in patients. They showed that the single remaining transgene copy is functional and rescues the phenotype associated with the endogenous DMD knockout mouse[22].
Rat and rabbit
Dystrophin-deficient rats, larger and with more pronounced cardiomyopathy than mice, show significant muscle fibrosis and cardiac dysfunction by 7-10 months, aligning closer with human DMD disease progression[23]. CRISPR/Cas9-engineered rabbits, using a pair of sgRNAs targeting exon 51, display hallmark DMD symptoms, including muscle atrophy and significant cardiac involvement, within months[24].
Dog
Transitioning to larger animals, the golden retriever muscular dystrophy (GRMD) dog model mirrors the human condition with skeletal and cardiac dysfunctions and can now be assessed through advanced imaging techniques[25,26]. Although costly and complex to maintain, GRMD is a valuable model for cardiomyopathy research given the size of canine hearts and contraction rates are more relevant to DMD patients compared to murine hearts. However, the late onset nature of GRMD cardiomyopathy and lack of molecular reagents make it difficult to study mechanistic progression, thus limiting its preclinical usefulness[27].
Swine
Due to strong anatomical and physiological resemblance to humans, exon 52-deleted DMD swine model has also been generated that mimicked severe myopathy and impaired mobility[28]. In this model, a significant reduction of left ventricular ejection fraction was observed as early as in 4-month DMDY/− pig hearts, making them a good model for testing gene editing and new diagnostic techniques, including multispectral optoacoustic tomography (MSOT), which allows for non-invasive monitoring of disease progression[29].
Simian
Simian models in rhesus monkeys, generated by mutating DMD exons, offer a closer physiological and genetic approximation to humans[30,31]. DMD monkey models recapitulate the pathogenesis of human patients, displaying progressive muscle deterioration and impaired motor function. Using this model, it has been shown that immune cell landscape changes, stimulation of fibro-adipogenic progenitors (FAPs), TGF-β resistance in FAPs, and profound muscle stem cells (MuSCs) defects contributed to the muscle dysfunction of DMD[31].
Other animal models
Zebrafish model
The DMD gene in zebrafish shows a high degree of homology with human DMD. The zebrafish DMD model, established using gene editing technology, exhibits abnormal embryonic muscle development manifesting in muscle contraction dysfunction and aberrant muscle fiber morphology[32]. The zebrafish model is advantageous for high-throughput screens, given its rapid reproduction and transparency during embryo development[33,34]. However, due to their small size and relatively simple muscle structure, conclusions do not always translate fully to human cardiac and skeletal muscles.
Caenorhabditis elegans model
Although Caenorhabditis elegans are evolutionarily distant from humans, dystrophin homolog has been identified. The DMD model constructed using nematodes is mainly used to study muscle contraction mechanisms and apoptosis[35,36]. The nematode model is easy to operate, has a short life cycle, and can be used for large-scale genetic screening, but it cannot fully simulate the complex pathological process of human DMD.
Each model - from small rodents to large mammals-contributes unique insights into DMD pathogenesis and therapeutic developments, with larger models like dogs and pigs offering comprehensive physiological parallels for cardiac research[37]. The continued development of species-specific models aligned with human DMD pathology is vital for optimizing therapeutics, especially for cardiac complications. While further optimization and humanization of existing animal models are encouraged, how to integrate these tools (albeit making various animal models more available or more affordable) to accelerate clinical translation would also require more discussion with regulatory agencies. Further, with emerging human induced pluripotent stem cell technology, it is foreseeable that bridging human cellular data with animal in vivo data will facilitate clinical translation.
Human induced pluripotent stem cell-derived cardiomyocyte (hiPSC-CM) models
Although DMD skeletal muscle biopsies are accessible and can be used to isolate myoblasts, limited passaging capacity hinders its availability. Further, given cardiomyocytes do not proliferate and cardiac biopsies are almost impossible to obtain, human myocardial models are urgently needed. Luckily, human induced pluripotent stem cell (hiPSC) technology has allowed access to this research need. With more robust differentiation protocols becoming available, researchers now possess the ability to differentiate and study human skeletal and cardiac muscle cells in a dish.
Establishment and characterization of DMD hiPSC lines
With the emergence of reprogramming technology, it is now possible to generate mutation-containing hiPSCs and differentiate them into beating cardiomyocytes to study DMD cardiomyopathy[38]. To generate a relevant in vitro model for studying DMD cardiomyopathy, researchers isolated and reprogrammed hiPSCs from male DMD patients with well-characterized DMD mutations[39-43]. Given the substantial proportion of DMD mutations located within the exon 45-55 region, CRISPR/Cas9 gene editing strategies targeting this hotspot offer a robust approach for restoring the dystrophin reading frame. Utilizing specific guide RNAs (gRNAs), researchers achieved targeted deletion of exons 45-55 in DMD hiPSC lines, with requirements of 530 kb, 670 kb, and 725 kb deletions, respectively[44]. Stable DMD hiPSC lines with exon 45-55 deletions were established via clonal selection, and off-target effects were minimized, with no unintended mutations detected at any highly homologous sites analyzed using COSMID. Importantly, all corrected hiPSC lines retained normal karyotypes, indicating that CRISPR/Cas9 editing did not adversely impact overall chromosomal stability.
In addition to exon deletion, CRISPR-based reframing (RF) and exon skipping (ES) strategies were employed to restore dystrophin expression in DMD hiPSCs with disease-inducing deletions in exon 44[45,46]. In this approach, the correction focused on exon 45, creating either a RF or ES dystrophin transcript capable of producing a truncated, yet functional, dystrophin protein. These corrected hiPSC lines were differentiated into cardiac muscle cells (CMs) to evaluate dystrophin restoration and its effect on cellular morphology and function. RNA sequencing of differentiated CMs demonstrated that the truncated dystrophin proteins were biologically functional and successfully mitigated the transcriptional dysregulation associated with DMD. Notably, both RF and ES corrected lines prevented aberrant expression patterns commonly seen in dilated cardiomyopathy, underscoring the therapeutic potential of such corrections.
DMD pathophysiology using hiPSC-CM models
To further investigate the role of dystrophin in DMD pathophysiology, researchers explored interactions between dystrophin and the long noncoding RNA H19, focusing on dystrophin’s C-terminal zinc finger (ZNF) domain. Cross-linking immunoprecipitation (CLIP) and RNA immunoprecipitation (RIP) assays demonstrated that dystrophin associates with H19 motifs in both human and mouse muscle tissues, with specific AT-rich binding motifs critical for this interaction. Knockout studies in C2C12 (mouse myoblasts) cells confirmed that loss of either DMD or H19 disrupts dystrophin stability and results in downregulated dystrophin protein levels. Importantly, the reintroduction of wild-type H19, but not loss-of-function (LoF) mutants, successfully restored DMD-H19 interactions and stabilized dystrophin protein in hiPSC-derived skeletal muscle cells (SkMCs) and CMs[47]. This finding suggests that the DMD-H19 interaction may play a regulatory role in dystrophin stability, opening potential avenues for therapeutic intervention targeting DMD RNA-binding complexes.
Single-nucleus RNA sequencing (snRNA-seq) was conducted on DMD hiPSC-derived CMs and on an in vivo model of aged ΔEx44 DMD mice to examine the impact of dystrophin correction on global transcriptional profiles. The snRNA-seq data revealed significant transcriptional normalization in corrected CMs and ΔEx44 mice compared to uncorrected controls, demonstrating the utility of gene-edited hiPSCs in reversing DMD-associated cardiac abnormalities[45].
In addition to gene-editing strategies, researchers have investigated cellular aging mechanisms in DMD, particularly the role of telomere shortening in cardiomyocyte dysfunction[21]. DMD-related mutations are linked to accelerated telomere attrition in hiPSC-CMs[43], suggesting a potential mechanistic pathway through which dystrophin deficiency exacerbates muscle and cardiac deterioration. Telomeres, repetitive DNA sequences that protect chromosome ends, become critically shortened in DMD hiPSC-CMs, paralleling disease severity and dysfunction. By upregulating telomeric repeat-binding factor 2 (TRF2), a telomere-binding protein, researchers demonstrated that telomere attrition could be mitigated, thus preserving cardiomyocyte size, structural integrity, and survival in DMD models[48]. These findings highlight telomere maintenance as an adjunctive therapeutic approach in DMD, complementing gene correction efforts and potentially delaying the progression of cardiomyopathy.
DMD patient-derived hiPSC models provide an invaluable platform for understanding disease mechanisms and models for screening interventions for DMD. Through a combination of gene correction, targeted exon deletion, and exon skipping, these models demonstrate that truncated dystrophin proteins can restore functional stability to DMD hiPSC-CMs, both in vitro and in animal models. Additionally, the association of dystrophin with H19 suggests a potential regulatory mechanism that could be leveraged to enhance dystrophin stability. Together, conclusions gained from hiPSC technology have yielded promising new biology and new targets for developing new treatments for DMD cardiomyopathy.
HiPSC-derived 3D models
The development of three-dimensional (3D) in vitro systems using hiPSCs enables us to study the crosstalk between various cell types and the microenvironment. The utilization of hiPSCs combined with bioengineered strategies allows access to study any dystrophic cell type[49,50]. Human skeletal muscle organoids (SMOs) and engineered skeletal muscles (ESMs) with a regeneration-competent satellite-like cell pool have been developed to study muscle development, maturation, disease, and repair. Myogenic progenitors produced by the organoids have been demonstrated to participate in muscle regeneration when transplanted into animals[51-53] and these human SMOs/ESMs afford the potential for high throughput drug screens.
Engineered heart tissues (EHTs) have emerged as a powerful tool in studying DMD cardiomyopathy. EHTs are created through a process that involves combining hiPSC-CMs, and in some cases with non-cardiomyocytes, with aqueous extracellular matrices. Given that hiPSC-CMs spontaneously beat, EHT allows functional characterization to better our understanding regarding the role of Ca²⁺ handling and transcriptomic dysregulation in dystrophic hearts[54,55].
These 3D models will permit the assessment of how DMD cells respond to mechanical, electrical, and chemical stimuli. This, in turn, will be instrumental in the exploration and development of novel and potentially effective pharmaceutical strategies for treating DMD cardiomyopathy.
THERAPEUTIC APPROACHES
DMD is a severe genetic disorder, and current treatment approaches focus on managing symptoms, slowing disease progression, and improving the quality of life for patients. General therapeutic designs for DMD can be categorized into pharmacological, cell and cell-derived biologics, and gene therapies [Figure 1].
Figure 1. Therapeutic approaches for DMD. General therapeutic designs currently available or under investigation for DMD, which can be broadly classified into pharmacological, cell and cell - derived biologics, and gene therapies. DMD: Duchenne muscular dystrophy.
Targeting dystrophin deficiency
Stop codon readthrough
Stop codon readthrough therapy is primarily used to treat DMD patients who carry nonsense mutations, applicable to approximately 10% of patients. Nonsense mutations result in premature stop codons that terminate translation prematurely and produce non-functional dystrophin. Stop codon readthrough employs drugs to alter the conformation of mRNA or the ribosome itself in DMD patients, inserting amino acids at the abnormal stop codon to allow the translation process to bypass the mutated stop codon, thereby producing partially functional dystrophin[56]. This therapy aims to restore or partially restore dystrophin function to improve symptoms in DMD patients. Ataluren, a small-molecule drug, is a codon readthrough therapy medication that binds weakly to multiple sites on the ribosome, inducing the ribosome to continue adding amino acids upon encountering a stop codon, thereby achieving readthrough. In July 2014, Ataluren was granted conditional approval by the European Union for use in patients with nonsense mutation DMD age 2 years and older. However, the drug was not approved in the United States, and its efficacy and safety profile vary greatly among DMD patients[57,58]. Additionally, some aminoglycoside antibiotics, such as gentamicin, also have readthrough effects. However, while gentamicin can increase dystrophin expression in mdx4cv mice, safety concerns such as nephrotoxicity and neurotoxicity remain a challenge[59,60]. Lastly, our tools are limiting to evaluate whether various truncated DMD proteins generated by readthrough therapy can confer efficacy.
Compared to cell and gene therapies, stop codon readthrough molecules are easier to synthesize, cheaper, and more stable. However, the efficacy of stop codon readthrough is often variable in DMD patients. First, the position of the stop codon may or may not allow the production of a good dystrophin protein product – this could be dependent on whether forced readthrough would still allow the production of a properly folded dystrophin protein. Second, the efficiency of forced ribosome readthrough may not be 100%; thus, whether sufficient dystrophin protein is produced is variable. Last, given that stop codon readthrough drugs are non-specific, it is inevitable that toxic side effects exist.
Exon skipping
Exon skipping is designed to generate truncated but in-frame dystrophin transcripts by skipping the mutant exon. It is reasoned that when an in-frame mutation occurs in the DMD gene, the resulting dystrophin protein, though internally truncated, retains partial functionality and clinically, treated DMD patients would transition into Becker Muscular Dystrophy (BMD). In support, it has been shown that frame-shift mutations that disrupt the ORF, which result in the loss of critical DMD functional domains, lead to the more severe DMD phenotype[61,62]. Exon skipping therapy employs pre-designed antisense oligonucleotides (ASOs) that specifically target pre-mRNA, inhibiting splicing enhancer sites to prevent specific exons from participating in splicing during the splicing of pre-mRNA[63]. Although theoretically applicable to all DMD patients except those with nonsense mutations, the specificity of ASOs necessitates multiple drugs to cover a large patient population[64]. Currently, exon skipping therapy has made significant progress. Notably, for mutations in exons 45-55 of the DMD gene, therapeutic drugs targeting exon 51 skipping, such as Eteplirsen by Sarepta Therapeutics, have been approved by the U.S. Food and Drug Administration (FDA), marking the first new treatment for DMD treatment[65]. Drugs targeting exon 53 skipping, like Sarepta’s Golodirsen[66] and Japan’s Viltolarsen[67], have also received relevant approvals. Additionally, drugs targeting other exons are under development, such as Sarepta’s Casimersen, which targets exon 45 skipping[68].
Exon skipping therapies enable the production of a partially functional dystrophin protein and have clinically conferred some improvements in muscle strength. Theoretically, oligonucleotide-based exon skipping designs can cover a wide range of DMD mutations. However, rare mutations often prohibit clinical trial designs and partial dystrophin expression does not always result in muscle function improvement. Limited muscle stem cell penetrance and drug dilution due to constant muscle repair cycles would require life-long treatment regimes.
Gene replacement therapy
Since DMD is caused by the deficiency or dysfunction of dystrophin, restoring dystrophin expression and function has become the key to treating DMD. Conventional gene therapy strategies struggle to deliver the full-length DMD gene to patients due to size constraints. To resolve this, researchers developed micro-dystrophin, a dystrophin with a truncated central rod domain, resulting in the absence of most spectrin-like repeat domains and retaining only DPAC domains that interact with the cytoskeleton to maintain core function[69]. This shortened dystrophin protein retains partial functions, thereby improving muscle function in patients. Viral vectors (such as AAV) were used to deliver the gene encoding micro-dystrophin into muscle tissue; non-viral vectors, such as liposomes and nanoparticles, have relatively low delivery efficiency, yet their advantage lies in the simplicity in preparation and ease of modification. The delivery of micro-dystrophin via AAV vectors has demonstrated the potential to ameliorate dystrophic pathology in various mouse and canine models of DMD[70-72], hinting that the introduction of micro-dystrophin genes could be sufficient to transition the DMD phenotype toward a less severe Becker-like clinical manifestation.
SRP-9001 (also known as AAVrh74.MHCK7. micro-Dystrophin) is a gene therapy developed by Sarepta Therapeutics in joint with Roche that has shown positive clinical trial results. Preliminary clinical trial results indicate that SRP-9001 gene therapy can significantly increase micro-dystrophin expression in patients’ muscles and improve their motor function[73]. For example, biopsy samples of the gastrocnemius muscle taken 12 weeks after infusion showed that an average of 81.2% of muscle fibers expressed micro-dystrophin, with an average expression intensity of 96% at the sarcolemma. An ongoing global multicenter Phase III clinical study (EMBARK) utilizes western blot to quantify micro-dystrophin expression at 12 weeks post injection as a secondary efficacy indicator. In June 2023, SRP-9001 (rAAVrh74.MHCK7.micro-dystrophin, ELEVIDYS, Sarepta) received conditional approval for use in boys aged 4 to 5 years with DMD who do not have deletions in exon 8 and/or 9, marking it as the first approved gene therapy product for DMD[74]. Although its Phase III confirmatory clinical study did not achieve the primary motor function endpoint of the North Star Ambulatory Assessment (NSAA), it did meet secondary endpoints and is now employed in treatment.
There is a possibility of achieving long-term expression of micro-dystrophin as AAV vectors can integrate into muscle cells without causing significant genotoxicity, providing a sustained source of the protein. However, delivering AAV vectors to all affected muscles in the body remains challenging and is limited by liver toxicity when AAVs are administered through intravenous injections. It is noteworthy that preexisting adaptive immunity to the viral vectors used prohibits these patients from receiving AAV treatments[75]. Additionally, there is the possibility of AAV genome dilution due to muscle regeneration, leading to a progressive decline in the expression of micro-dystrophin[76]. This may allow the pathological process of muscular dystrophy to recur, limiting the durability of the treatment effect.
Additionally, in various AAV clinical trials, the incidence of “Severe Adverse Events (SAEs)” has been a key area of concern[77]. Some trials have reported low incidences of SAEs directly related to the AAV-microdystrophin treatment, mainly involving immune-related responses and organ-specific toxicities[78-80]. In some cases, immune responses to the AAV vector or dystrophin have been observed[79-81]. Patient age, underlying health status, and genetic background may significantly influence the incidence of SAEs. In addition, researchers are working to improve the safety of gene therapy through vector optimization and dose and route of administration adjustments[82,83]. How to balance between efficacy and toxicity remains challenging.
Gene editing
Gene editing therapy is a cutting-edge treatment approach for DMD. It utilizes gene editing tools such as the CRISPR/Cas9 system to make precise modifications directly within the patient’s genome. These tools can identify and cut specific sequences in DNA, and then utilize the cell’s natural repair mechanisms to insert, delete, or replace gene fragments[84].
Strategies utilizing CRISPR/Cas9 technology to treat DMD are designed to either repair or bypass mutations that cause the disease, thereby restoring the function of dystrophin[85]. The initial demonstrations of CRISPR/Cas9-mediated correction in the context of DMD employed an exon excision approach. This method is conceptually akin to exon skipping, where two double-strand breaks (DSBs) are induced in intronic sequences adjacent to a target exon using a pair of single guide RNAs (sgRNAs). Subsequently, the resulting lesions are repaired through the Non-Homologous End Joining (NHEJ) pathway, removing the intervening DNA[86]. The first demonstration targeted exon 23 of DMD in the mdx mouse model[85]. In addition to directly repairing gene mutations, the CRISPR/Cas9 system can also affect the expression level of the dystrophin gene by editing adjacent DNA sequences (such as promoter or enhancer regions).
Engineered CRISPR technologies of base editing and prime editing have expanded the gene editing toolbox, enabling precise corrections of genetic mutations at individual nucleotides. Base editing that uses a nickase Cas9 (nCas9) enzyme fused to a base editing enzyme like adenine base editors (ABEs) can target single nucleotide variants. This system minimizes the risk of indel formation and chromosomal rearrangements[87]. Base editing using the nCas9-ABE system has been used to correct a nonsense mutation in mdx mice and achieved exon skipping in dystrophic ∆Ex51 mice by disrupting splice sites[88,89]. However, using nCas9-ABE system in vivo requires special delivery methods due to its large size[90]. The NG-targeting adenine base editor (iABE-NGA) demonstrates high efficiency in precisely editing a DMD mutation in adult mice[91]. Prime editing is an alternative system that can install specific edits directly into target genomes using a catalytically impaired Cas9 fused to reverse transcriptase and a specialized guide RNA[92]. Prime editing has been used to reframe dystrophin ORFs in hiPSCs but has yet to be demonstrated in vivo[88].
The clinical trials of CRISPR/Cas9 gene editing therapies for DMD are still in the exploratory stage. The CRD-TMH-001 therapy, developed by Cure Rare Disease, encountered setbacks in clinical trials, with the only subject, unfortunately, passing away for unclear reasons[93]. Although the first clinical trial had an unexpected outcome, it also provided valuable experience and lessons for the development of this technology. A preclinical trial designed to investigate the efficacy and safety of GEN6050X, cytosine base editing therapy using dual AAV9 vectors, in DMD patients was also launched by GenAssist Ltd for DMD patients amenable to exon 50 skipping (NCT06392724). On top of AAV toxicity, gene editing therapies are also challenged with off-target side effects. The long-term effects of gene editing therapies in DMD patients remain to be determined.
Cell therapy
Historically, myoblast transplantation has been of great interest for treating muscle loss for DMD patients. However, low cell survival and poor integration of the transplanted myoblasts make this strategy difficult. Further, immune activation against foreign myoblasts results in quick elimination and inflammatory damage. Although researchers have turned to mesenchymal stem cells for their immunomodulatory and growth factor secretory properties[94], how to overcome and repair inflammatory dystrophic tissue remains challenging.
HiPSCs have emerged as ideal autologous seed cells for cellular therapy due to the superior regenerative capacity of their derived myogenic progenitor cells. Using CRISPR/Cas9, corrected hiPSCs are differentiated into skeletal and cardiac myocytes, both capable of restoring dystrophin expression[95]. In comparison with allogeneic cell transplantation therapy, autologous transplantation can greatly reduce immune rejection, making it a promising strategy for the treatment of DMD. Preclinical studies using hiPSC-derived muscle cells in animal models demonstrated the potential of this approach; the transplanted hiPSC-derived cells were able to contribute to muscle regeneration and showed improved muscle function[96,97]. However, challenges remain, including long-term stability and the degree of integration of transplanted cells into the dystrophic muscle.
The use of allogeneic cardiosphere-derived cells (CDCs)-secreted extracellular vesicles (EVs) have been shown to mediate regenerative and repair capabilities[98,99]. Alternatively, dystrophin-expressing chimeric cells (DECs) are genetically engineered to express dystrophin and current clinical results indicate improvements in muscle strength and motor function in DMD patients[100].
Utrophin modulation
Utrophin (UTRN) is a gene related to dystrophin (DMD) located on chromosome 6q24. UTRN is expressed during fetal development, at the neuromuscular and myotendinous junctions, and during muscle regeneration. So far, five full-length utrophin first exons have been identified in humans (A, A’, B, B’, and F). Utrophin shares a comparable structure and function with dystrophin, but it lacks two spectrin-like repeats and the crucial nNOS-binding site[101-103] and overexpression does not fully rescue the disordered microtubule network pattern observed in dystrophic myofibers[104]. In DMD patients who lack dystrophin protein, utrophin protein has been found to be present in sarcomeres and co-localized with acetylcholine receptors, playing a compensatory role in stabilizing the synaptic cytoskeleton[105-106]. Since utrophin may functionally compensate for the absence of dystrophin, increasing its expression in DMD muscle could functionally compensate for the absence of dystrophin and ameliorate muscle pathology. This is further supported by the observation that dystrophic pathology is more severe in dystrophin and utrophin double knockout (mdx4cv/utrn-/-) mice and that genetic overexpression of full-length utrophin on an mdx4cv background can prevent the development of dystrophic pathological features[16,107]. Therefore, upregulating utrophin is a promising therapeutic strategy for DMD regardless of their mutation type.
The use of small-molecule modulators that can bind to specific targets within cells is currently one of the leading strategies for upregulating utrophin[108,109]. Utrophin minigene variants have shown promise in improving histopathology and reducing serum creatine kinase (CK) levels in various models of DMD[110]. Furthermore, adeno-associated virus (AAV)-delivered micro-utrophin constructs have been shown to improve muscle function and increase lifespan in mdx4cv/utrn-/- knockout mice[111]. New approaches to upregulate utrophin have also been demonstrated in DMD animals, including using CRISPR activation (CRISPRa)[112] and deletion of miRNA target sites in the UTRN 5′ untranslated region (UTR)[113].
There are several clinical trials investigating the efficacy and safety of utrophin upregulation therapies in DMD patients, including a phase 2 clinical trial assessing the ability of Ezutromid to increase utrophin expression and improve muscle function in DMD patients[108]. Whether utrophin upregulation can alleviate DMD muscle function regardless of mutation type remains to be determined.
EMERGING THERAPEUTICS
As new signaling pathways involved in DMD pathogenesis are being uncovered, new therapeutic strategies and new delivery modalities are also emerging.
Inflammatory signaling and macrophage behavior modulation
The corticosteroids prednisone/prednisolone and deflazacort are current gold standards of pharmacological care in DMD due to their potent anti-inflammatory effects. Both drugs are used to stabilize motor function, control symptoms, and slow disease progression. However, long-term corticosteroid use is associated with significant systemic adverse effects. To counter corticosteroid side effects, Vamorolone (AGAMREE®) was developed by ReveraGen BioPharma and Santhera Pharmaceuticals. It lacks the 11β-hydroxyl/carbonyl moiety on the steroidal C ring, which is known to drive corticosteroid-associated adverse effects on bone in mouse models[15,114,115]. Vamorolone was approved in October 2023 for the treatment of DMD in patients 2 years of age and older in the USA and for the treatment of DMD in patients 4 years of age and older in the EU[116].
Macrophages play a crucial role in the context of muscle regeneration and DMD. They serve as key regulators during muscle regeneration by modulating the inflammatory response and facilitating tissue repair and regeneration. Following muscle injury, macrophages rapidly infiltrate the damaged area and initiate the regulation of the inflammatory response by releasing a series of mediators. With their powerful phagocytic capacity, macrophages clear damaged muscle cells and cellular debris, creating space for the growth of new muscle fibers. By secreting signaling molecules such as growth factors and cytokines, macrophages promote the proliferation, differentiation, and fusion of myoblasts, thereby accelerating the muscle regeneration process. Moreover, macrophages exhibit phenotypic switching during muscle regeneration, transitioning from proinflammatory (M1) to anti-inflammatory (M2)[117]. In the mdx mouse model, M1 macrophages significantly exacerbate muscle damage[118,119]. Studies have shown that the number of M1 macrophages increases during the early acute phase of mdx muscle pathology. These macrophages are primarily responsible for causing inflammatory damage to dystrophic muscle fibers, through the production of nitric oxide by inducible nitric oxide synthase (iNOS)[120]. This phenotypic switching plays a crucial role in the regulation of inflammatory response, promotion of proliferation and differentiation of muscle cells, and prevention of excessive fibrosis. Mutations in the DMD gene result in defective muscle proteins, making muscle cells susceptible to damage and degeneration[121]. Prior research established NFIX as a key factor connecting RhoA-ROCK1-dependent phagocytosis with the transition of macrophages from a proinflammatory to an anti-inflammatory state[122]. Expression of Nfix by macrophages during the progression of dystrophy plays a role in accelerating disease progression, affecting both myogenic cells and fibro-adipogenic progenitors (FAPs) in mice. Removing Nfix from the macrophages of dystrophic mice results in a delayed onset of fibrosis and muscle wasting, while also enhancing grasp force[123,124]. Babaeijandaghi et al. have demonstrated that PLX73086, a small-molecule inhibitor of CSF1R currently being clinically investigated for the treatment of rare cancers, is capable of eliminating both TIMD4+ and TIMD4- muscle-resident macrophage cells[125]. Their study revealed that administering CSF1R inhibitors to mdx mice protected dystrophic muscles from eccentric contraction-induced injury, both in vivo and ex vivo settings. The observed protective effect is likely due to a shift in the balance between damage-sensitive glycolytic fibers and more resilient glycolytic-oxidative fibers. Although new ideas for targeting macrophages are emerging - macrophage phenotypic switching, enhancing phagocytic capacity, and promoting the secretion of growth factors - more work is warranted before this idea migrates to clinical testing.
Histone modifications
DMD muscles are observed to have abnormal HDAC activity due to impaired nitric oxide (NO) signaling. NO is an important signaling molecule that plays a crucial role in regulating cell function, vascular tone, and immune response. Impaired NO signaling may fail to effectively inhibit HDAC activity, leading to an irregular increase in HDAC activity. Elevated HDAC activity induces widespread change in gene expression by altered chromatin structure and the accessibility of DNA, contributing to the pathological development of DMD[126]. Givinostat is an HDAC inhibitor that has been shown to have a therapeutic effect on muscle histopathology. In studies on mdx mice, Givinostat significantly improved muscle histopathology and reduced inflammation and muscle loss. Additionally, in a Phase I/II DMD clinical trial (NCT01761292), Givinostat was found to significantly improve muscle function and quality of life for patients. By inhibiting HDAC, Givinostat inhibits inflammation, promotes muscle regeneration, and reduces muscle loss. Givinostat offers new hope for the treatment of muscular dystrophy diseases including DMD[127]. Further research and exploration are underway for HDAC inhibitors to determine optimal dosage, treatment duration, and long-term efficacy.
Gut microbiota as a regulator
The relationship between gut microbiota and DMD pathogenesis is a complex and emerging field of interest. While direct links between gut microbiota and DMD have not been fully elucidated, there is growing evidence suggesting that the gut microbiome may play a role in the modulation of inflammatory processes, metabolic pathways, autophagy, and neuromuscular functions relevant to DMD pathogenesis. Farini et al. found a significant correlation between the features of DMD and the abundance of Prevotella bacteria[128]. The absence of gut microorganisms was found to impact muscle immunity and fibrosis and mdx mice showed decreased inflammation and improved muscle pathology and function when Prevotella was re-introduced. In a separate study, the plasma of mdx mice exhibited decreased levels of gut microbiota-derived metabolites, particularly short-chain fatty acids (SCFAs), and increased levels of endocannabinoids[129]. Administration of the SCFA and sodium butyrate (NaB) effectively restored muscle strength and autophagy, and inhibited inflammation linked to heightened endocannabinoid signaling at CB1 receptors. These results suggest a possible role for gut microbiota in the development of DMD.
Emerging treatments for DMD cardiomyopathy
Mitochondrial therapeutics
Mitochondria transplantation
Mitochondrial transplantation refers to the technique of injecting isolated healthy mitochondria into damaged tissues or into the circulatory system to exert therapeutic effects. Mitochondria are known as the “powerplant” of cells. They are crucial to the production of adenosine triphosphate (ATP), the energy source for biological functions. Mitochondrial dysfunction can lead to a series of diseases such as mitochondrial disorders, myocardial ischemic injury, and brain injury. The principle of mitochondrial transplantation is to improve the overall mitochondrial function in recipient cells. Mitochondrial transplantation has been validated in multiple studies to restore energy metabolism and normal function in damaged cells[130,131]. Mitochondrial transplantation shows potential in the treatment of ischemic injury[132-134]. The first report of mitochondrial transplantation technology in the treatment of the ischemic heart of rabbits was published in 2009, showing that mitochondrial transplantation can help the damaged heart recover from ischemic injury[135]. Mitochondria-rich extracellular vesicles could rescue patient-specific cardiomyocytes from Doxorubicin injury, showing improved contractility, reactive oxygen species production, ATP production, and mitochondrial biogenesis[136]. Artificially transplanted mitochondria in endothelial cells (ECs) could promote mitophagy, transiently enhance EC bioenergetics, and enable them to form functional vessels in ischemic tissues without the support of MSCs[137]. Studies have also shown that mitochondrial transplantation can protect neuronal survival and promote the secretion of brain-derived neurotrophic factor (BDNF), hence improving neurological function[138]. By transplanting healthy mitochondria, the mitochondrial function and vitality of damaged neurons can be restored, thereby slowing disease progression[139].
By combining dystrophin-expressing chimeric cells (DECs) and mitochondrial transfer, human myoblast-mitochondria fusion products were shown to mitigate DMD symptoms[140]. Our previous work also demonstrated that healthy hiPSC-CMs isolated from mitochondria can restore contractile function in DMD hiPSC-CMs[141]. Since mitochondria are crucial for the function of both skeletal and cardiac myocytes, mitochondrial transplantation opens a new avenue for treating DMD[142-144]. Invasive delivery methods include oral mitochondrial transplantation using nanomotors[145], nasal administration[146], and direct injections.
However, mitochondrial transplantation is not without challenges. Issues including mitochondria targeting, long- versus short-term efficacy, immune response, residual mitochondrial DNA, and ethical guidelines regarding the source of mitochondria need to be addressed before this new technology can be translated into the clinics.
Targeting cellular/mitochondrial redox imbalance
Past studies have shown that mitochondrial hydrogen peroxide (mH2O2) levels are elevated during the early stages of disease progression in the cardiac of DMD patients[147], leading to the development of mitochondrial-targeted antioxidant therapies, treatments that directly combat oxidative stress within mitochondria. Two notable antioxidants, MitoQ and SkQ1, have been developed to specifically target mitochondria. These antioxidants are conjugated with a lipophilic triphenylphosphonium (TTP) cation, which facilitates their entry into mitochondria to mitigate oxidative damage[148]. Small peptides known as ‘SS’ peptides were demonstrated to preserve mitochondrial function. These peptides are permeable to mitochondria and capable of binding to cardiolipin, a phospholipid found in the inner mitochondrial membrane (IMM). By interacting with cardiolipin, SS peptides take part in regulating oxidative phosphorylation and preventing oxidative damage[149]. Using DMD hiPSC-CMs, we also demonstrated that data mining from clinical Chinese herbal medicine (CHM) usage shows that targeting Catalase (CAT) (targeted by quercetin, kaempferol, and vitamin C) confers ROS protection and improved contraction in DMD hiPSC-CMs[150]. Although antioxidants can alleviate DMD, as do with most small molecules, more studies in optimizing targeting and pharmacokinetics are needed before these compounds can be tested clinically.
Degeneration of mitochondrial structures was identified in dystrophic cardiomyocytes isolated from the hearts of 1-month-old and 3- to 4-month-old mdx4cv mice, characterized by swelling and loss of cristae prior to the onset of clinical symptoms of DMD[151]. Abnormal mitochondrial ultrastructure was also observed in the hearts of 12-month-old and older C57BL/10 mdx mice after the development of dilated cardiomyopathy (DCM), suggesting that mitochondrial abnormalities may be an early biomarker of DMD-related cardiomyopathy[152]. DMD hiPSC-CMs exhibit an increased number of morphologically abnormal mitochondria, hinting at the underlying mitochondrial dysfunction at a cellular level[153]. Autophagy has emerged as a pivotal process in sustaining skeletal muscle function by preserving homeostasis through the degradation of altered or damaged organelles. A study demonstrated a significant increase in the co-localization of LC3 dots with fragmented mitochondria in the hearts of 22-week-old C57BL/10 mdx mice in comparison to wild-type controls[154], suggesting altered mitophagy may play a role in mitochondrial dysfunction. The AMP-activated protein kinase (AMPK) and peroxisome proliferator-activated receptor coactivator-1α (PGC-1α) signaling pathways promote cellular autophagy and suppress inflammation[155]; however, activation of AMPK and PGC-1α diminishes with age and can lead to skeletal muscle function decline[156]. Moreover, blocked autophagy leads to the accumulation of dysfunctional mitochondria, drives up reactive oxygen species (ROS), and activates the formation of Nod-like receptor family pyrin domain-containing 3 (NLRP3) inflammasomes. The NF-κB signaling pathway fuels the production of NLRP3, creating a complex interplay between autophagy, inflammation, and the decline in skeletal muscle function. Resveratrol and quercetin have been shown to induce PGC-1a expression, increasing cell survival and reducing necrosis and levels of oxidative stress[157-159].
Targeting cellular/mitochondrial ion homeostasis
Targeting calcium handling
Under normal physiological conditions, depolarization of the sarcolemma triggers the interaction between the L-type voltage-gated calcium channel/dihydropyridine receptor located in the T tubule and the ryanodine receptor (RyR) on the sarcoplasmic reticulum (SR). This interaction releases calcium ions (Ca2+) from the SR into the cytoplasm, essential for muscle contraction. Following contraction, Ca2+ is actively transported back into the SR through the sarco/endoplasmic reticulum calcium ATPase (SERCA) to restore the cytosolic Ca2+ concentration necessary for muscle relaxation[160]. In contrast, RyR undergoes nitrosylation and phosphorylation due to oxidative stress and abnormal signaling (such as through the DAPC member protein kinase A) in DMD[161]. Leakage of Ca2+ from SR due to RyR abnormalities disrupts physiological [Ca2+] and triggers muscle injury[162,163]. Prolonged elevation of cytosolic [Ca2+] causes overactivated calcium-dependent enzymes, including phospholipase A2 and calpain, to attack mitochondria and other membrane phospholipids. Membrane damage generates lysophospholipids and free fatty acids, which induce mitochondrial ROS production[164]. Simultaneously, elevated cytosolic [Ca2+] can also drive mitochondrial calcium overload. Calcium overload can lead to mitochondrial permeability transition pore (MPTP) opening, leading to depolarized mitochondrial potential, reduced ATP production, and impaired cellular energy metabolism[165]. Moreover, the instability of mitochondrial membrane and channel proteins generates massive efflux of Ca2+ from mitochondria, further increasing the cytosolic Ca2+ concentration, initiating a vicious cycle that ultimately leads to apoptosis and muscle dysfunction[166]. RyR stabilizers have been found to significantly reduce muscle pathology in the mdx mouse model[167]. Studies have shown that the expression of sarcolipin, a small peptide inhibitor of SERCA activity, is significantly increased in dystrophic muscle. Overexpression of SERCA[168,169] or depletion of sarcolipin[170] can ameliorate skeletal muscle and cardiomyopathy in symptomatic DMD mouse models, suggesting the potential of treating DMD muscle dystrophy by targeting calcium homeostasis.
Dysregulation of potassium channels
Potassium ions (K+) play vital roles in cardiomyocytes by maintaining the resting membrane potential, repolarizing the action potential, and regulating cellular excitability. Cardiomyocytes facilitate the transmembrane transport of potassium ions through potassium ion channels. The KATP channel is composed of Kir6.2 and sulfonylurea receptor (SUR) subunits, with the Kir6.2 subunit interacting with dystrophin[171]. In DMD, due to the deletion or mutation of dystrophin, this interaction is lost, resulting in decreased KATP channel activity and reduced KATP currents[172]. KATP channels play an important role in regulating cardiomyocyte metabolism and responding to stress conditions such as ischemia and hypoxia, and their functional decline may exacerbate myocardial damage[173-174]. In DMD murine cardiomyocytes, inward rectifier potassium currents primarily mediated by Kir2.1 channels are significantly reduced[175]; in DMD canine cardiomyocytes, a decrease in transient outward potassium currents was reported[176]. These potassium currents play a pivotal role in the initial repolarization phase of the action potential, and their reduction may prolong the repolarization process of the action potential.
Iron overload in DMD
Heart iron overload is often found in heart failure and iron overload is usually caused by dysregulated iron homeostasis such as excessive intestinal iron absorption and/or aberrant iron storage and export[177]. In DMD patients, iron overload in the heart is hard to detect in the early stages. This is due to the fact that the accumulation of iron occurs gradually first in the epicardium, while later it is also observed in the myocardium and endocardium[178]. Iron accumulation studies have demonstrated slow iron accumulation in DMD patient hearts using cardiac MRI, which is marked by a shortening of the T2 relaxation time[179]. Impaired iron metabolism in DMD hiPSC-CMs provoked an increase in oxidative stress and mitochondria damage, which could be reversed with deferoxamine (iron chelator) and pioglitazone (mitoNEET stabilizing compound)[180,181]. Further, iron overload was also observed in muscle samples from mdx4cv and mdx4cv/utrn-/- knockout animals and iron chelation treatment with deferiprone ameliorated the muscle pathology, decreased ROS production, and improved mitochondrial function[182]. These findings provide support for targeting iron homeostasis for treating DMD.
COMBINATORIAL THERAPY
As more discussions are happening around combinatorial therapy for DMD, one would have to consider some of the key fundamental assumptions we, as a field, are making. Is restoration of dystrophin protein or its truncated form sufficient to arrest or reverse muscle dysfunction? If we assume this assumption is true, then one should consider ways that can boost the efficiency of available and developing treatments. In keeping with this logic, the co-administration of dantrolene, an exon skipping enhancer drug, with histone deacetylase (HDAC) inhibitors has been explored. It has been demonstrated that HDAC inhibitors can potentially modify the DNA to be more accessible for exon skipping[183]. Moreover, dantrolene shows synergistic enhancement with antisense-mediated exon skipping treatment in reprogrammed DMD myotubes and AO-mediated exon skipping treatment in DMD hiPSC-CMs[184,185]. Combination of cell therapy and AAV-micro-dystrophin therapy has also been explored. Co-administration of bone marrow-derived MSCs with AAV-micro-dystrophin resulted in muscle improvement in dystrophic dogs[186], likely through immune modulation, enabling more efficient AAV delivery. Another strategy involves pairing dystrophin restoration therapy with microRNA (miRNA) inhibition. By inhibiting miRNAs, upregulated by dystrophin deficiency, increased dystrophin restoration efficacy has been observed[187,188]. Dystrophin restoration using adeno-associated virus (AAV)-U7-mediated exon skipping therapy has improved drastically in treated muscles. The PPMO (peptide-phosphorodiamidate morpholino) pre-treatment allowed efficient maintenance of AAV genomes in mdx muscles and enhanced the AAV-U7 therapy effect with a ten-fold increase in protein levels after 6 months[189]. In addition, many other combinatorial treatments have also been explored with dystrophin restoration strategies to enhance muscle function[190]. Small molecules that inhibit muscle degeneration, modulate inflammation, neutralize oxidative stress, block fibrosis, or enhance muscle regeneration are considered[33,191].
In conclusion, the complexity of DMD disease calls for a multifaceted approach. Combinatorial therapies offer the potential to synergistically expand our therapeutic toolbox. Of course, new combinations need to be developed and refined before clinical translation.
LIMITATIONS AND FUTURE DIRECTIONS
This review article is an attempt to cover as much ground as possible regarding therapeutic strategies in treating DMD. It is clear that most therapeutic strategies that have entered clinical trials aim to restore the full or parts of the dystrophin protein through gene therapy. The limitations of these gene editing and gene therapies also warrant caution. First, to treat all the muscles, delivery modalities are often used at high doses. This presents not only a technological challenge on the manufacturing side but also increased risks for DMD patients. Second, a large dosage also entails high costs, not only in manufacturing but also in low-temperature logistics. The economic burden on healthcare systems, particularly in developing countries, needs careful evaluation. Third, by the nature of AAV gene therapy design, the treatment is typically administered only once. As a result, issues related to targeting efficiency arise, and patients can only commit to one gene therapy trial. Additionally, the risk of off-targets in gene editing remains to be clinically determined. So far, we have not seen a breakthrough success like Zolgensma for SMA in DMD gene therapy pipelines. Earlier gene therapy intervention will require more clinical input. Fourth, whether restoration of dystrophin protein (or truncated form) is sufficient to reverse muscular and cardiac progression directly remains to be determined, not to mention that AAV capsids often cannot target muscle satellite stem cells.
In response to these challenges, we propose the following suggestions. Without changing existing genetic cargo, there is an urgent need for a gene delivery system that is affordable and allows multiple dosing. In the scenario where dystrophin protein restoration is insufficient to reverse phenotype due to compensatory mechanisms, identifying downstream pathways that are universal to all DMD patients is highly desirable. Biologics (mmRNAs) or new delivery modalities (exosomes and mitochondria) may enable repetitive dosing regimens for existing drug designs. Additionally, advancements in nanotechnology and non-viral drug delivery systems may enable more efficient and targeted delivery of therapeutic agents[192,193].
In all, there are many molecular medicine approaches that are on the horizon, in clinical trials, or approved for use in patients with DMD. However, little advancement has been seen in tackling DMD cardiomyopathy. In light of advances in cell and animal models, new delivery modalities/drug designs and knowledge gained from DMD trials, we are optimistic that more scientists will join the DMD community in finding a cure.
DECLARATIONS
Authors’ contributions
Wrote the first draft of the paper, performed literature search and data interpretation: Li B, Xiong WY, Chang CYA
Reviewed the paper and finalized the manuscript: Chang CHA, Chang, CYA
Made substantial contributions to the conception and content of the review: Li, B, Xiong WY, Chang CHA, Chang CYA
Figures were partially created with materials downloaded from Servier Medical Art (https://smart.servier.com/) by Xiong WY using Adobe Illustrator.
Availability of data and materials
Not applicable.
Financial support and sponsorship
None.
Conflicts of interest
All authors declared that there are no conflicts of interest.
Ethical approval and consent to participate
Not applicable.
Consent for publication
Not applicable.
Copyright
© The Author(s) 2025.
REFERENCES
1. Duan D, Goemans N, Takeda S, Mercuri E, Aartsma-Rus A. Duchenne muscular dystrophy. Nat Rev Dis Primers. 2021;7:13.
2. Petrof BJ, Shrager JB, Stedman HH, Kelly AM, Sweeney HL. Dystrophin protects the sarcolemma from stresses developed during muscle contraction. Proc Natl Acad Sci U S A. 1993;90:3710-4.
3. Culligan KG, Mackey AJ, Finn DM, Maguire PB, Ohlendieck K. Role of dystrophin isoforms and associated proteins in muscular dystrophy (review). Int J Mol Med. 1998;2:639-48.
4. Ahn AH, Kunkel LM. The structural and functional diversity of dystrophin. Nat Genet. 1993;3:283-91.
5. Górecki DC, Monaco AP, Derry JM, Walker AP, Barnard EA, Barnard PJ. Expression of four alternative dystrophin transcripts in brain regions regulated by different promoters. Hum Mol Genet. 1992;1:505-10.
6. Klietsch R, Ervasti JM, Arnold W, Campbell KP, Jorgensen AO. Dystrophin-glycoprotein complex and laminin colocalize to the sarcolemma and transverse tubules of cardiac muscle. Circ Res. 1993;72:349-60.
7. Moser H. Duchenne muscular dystrophy: pathogenetic aspects and genetic prevention. Hum Genet. 1984;66:17-40.
8. Blake DJ, Weir A, Newey SE, Davies KE. Function and genetics of dystrophin and dystrophin-related proteins in muscle. Physiol Rev. 2002;82:291-329.
9. Hoffman EP, Brown RH Jr, Kunkel LM. Dystrophin: the protein product of the Duchenne muscular dystrophy locus. Cell. 1987;51:919-28.
10. Birnkrant DJ, Bushby K, Bann CM, et al. DMD care considerations working group. Diagnosis and management of Duchenne muscular dystrophy, part 1: diagnosis, and neuromuscular, rehabilitation, endocrine, and gastrointestinal and nutritional management. Lancet Neurol. 2018;17:251-67.
11. McNally EM, Kaltman JR, Benson DW, et al. Working group of the national heart; lung; and blood institute; parent project muscular dystrophy. Contemporary cardiac issues in Duchenne muscular dystrophy. Working group of the national heart, lung, and blood institute in collaboration with parent project muscular dystrophy. Circulation. 2015;131:1590-8.
12. Miller RG, Hoffman EP. Molecular diagnosis and modern management of Duchenne muscular dystrophy. Neurologic Clinics. 1994;12:699-725.
13. Rio-Pertuz G, Morataya C, Parmar K, Dubay S, Argueta-Sosa E. Dilated cardiomyopathy as the initial presentation of Becker muscular dystrophy: a systematic review of published cases. Orphanet J Rare Dis. 2022;17:194.
14. Falzarano MS, Scotton C, Passarelli C, Ferlini A. Duchenne muscular dystrophy: from diagnosis to therapy. Molecules. 2015;20:18168-84.
15. Guglieri M, Clemens PR, Perlman SJ, et al. Efficacy and safety of Vamorolone vs. placebo and prednisone among boys with Duchenne muscular dystrophy: a randomized clinical trial. JAMA Neurol. 2022;79:1005-14.
16. Deconinck AE, Rafael JA, Skinner JA, et al. Utrophin-dystrophin-deficient mice as a model for Duchenne muscular dystrophy. Cell. 1997;90:717-27.
17. Grady RM, Teng H, Nichol MC, Cunningham JC, Wilkinson RS, Sanes JR. Skeletal and cardiac myopathies in mice lacking utrophin and dystrophin: a model for Duchenne muscular dystrophy. Cell. 1997;90:729-38.
18. Young CS, Mokhonova E, Quinonez M, Pyle AD, Spencer MJ. Creation of a novel humanized dystrophic mouse model of Duchenne muscular dystrophy and application of a CRISPR/Cas9 gene editing therapy. J Neuromuscul Dis. 2017;4:139-45.
19. Sacco A, Mourkioti F, Tran R, et al. Short telomeres and stem cell exhaustion model Duchenne muscular dystrophy in mdx/mTR mice. Cell. 2010;143:1059-71.
20. Mourkioti F, Kustan J, Kraft P, et al. Role of telomere dysfunction in cardiac failure in Duchenne muscular dystrophy. Nat Cell Biol. 2013;15:895-904.
21. Chang AC, Ong SG, LaGory EL, et al. Telomere shortening and metabolic compromise underlie dystrophic cardiomyopathy. Proc Natl Acad Sci U S A. 2016;113:13120-5.
22. Chey YCJ, Corbett MA, Arudkumar J, Piltz SG, Thomas PQ, Adikusuma F. CRISPR-mediated megabase-scale transgene de-duplication to generate a functional single-copy full-length humanized DMD mouse model. BMC Biol. 2024;22:214.
23. Sugihara H, Kimura K, Yamanouchi K, et al. age-dependent echocardiographic and pathologic findings in a rat model with Duchenne muscular dystrophy generated by CRISPR/Cas9 genome editing. Int Heart J. 2020;61:1279-84.
24. Sui T, Lau YS, Liu D, et al. A novel rabbit model of Duchenne muscular dystrophy generated by CRISPR/Cas9. Dis Model Mech. 2018:11.
25. Cooper BJ, Winand NJ, Stedman H, et al. The homologue of the Duchenne locus is defective in X-linked muscular dystrophy of dogs. Nature. 1988;334:154-6.
26. Kornegay JN. The golden retriever model of Duchenne muscular dystrophy. Skelet Muscle. 2017;7:9.
27. Guo LJ, Soslow JH, Bettis AK, et al. Natural history of cardiomyopathy in adult dogs with golden retriever muscular dystrophy. J Am Heart Assoc. 2019;8:e012443.
28. Klymiuk N, Blutke A, Graf A, et al. Dystrophin-deficient pigs provide new insights into the hierarchy of physiological derangements of dystrophic muscle. Hum Mol Genet. 2013;22:4368-82.
29. Stirm M, Klymiuk N, Nagashima H, Kupatt C, Wolf E. Pig models for translational Duchenne muscular dystrophy research. Trends Mol Med. 2024;30:950-64.
30. Chen Y, Zheng Y, Kang Y, et al. Functional disruption of the dystrophin gene in rhesus monkey using CRISPR/Cas9. Hum Mol Genet. 2015;24:3764-74.
31. Ren S, Fu X, Guo W, et al. Profound cellular defects attribute to muscular pathogenesis in the rhesus monkey model of Duchenne muscular dystrophy. Cell. 2024;187:6669-6686.e16.
32. Lambert MR, Spinazzola JM, Widrick JJ, et al. PDE10A inhibition reduces the manifestation of pathology in DMD zebrafish and represses the genetic modifier PITPNA. Mol Ther. 2021;29:1086-101.
33. Hightower RM, Reid AL, Gibbs DE, et al. The SINE compound KPT-350 blocks dystrophic pathologies in DMD zebrafish and mice. Mol Ther. 2020;28:189-201.
34. Wasala NB, Chen SJ, Duan D. Duchenne muscular dystrophy animal models for high-throughput drug discovery and precision medicine. Expert Opin Drug Discov. 2020;15:443-56.
35. Ellwood RA, Hewitt JE, Torregrossa R, et al. Mitochondrial hydrogen sulfide supplementation improves health in the C. elegans Duchenne muscular dystrophy model. Proc Natl Acad Sci U S A. 2021:118.
36. Ellwood RA, Piasecki M, Szewczyk NJ. Caenorhabditis elegans as a model system for Duchenne muscular dystrophy. Int J Mol Sci. 2021;22:4891.
37. Moretti A, Fonteyne L, Giesert F, et al. Somatic gene editing ameliorates skeletal and cardiac muscle failure in pig and human models of Duchenne muscular dystrophy. Nat Med. 2020;26:207-14.
38. Shi Y, Inoue H, Wu JC, Yamanaka S. Induced pluripotent stem cell technology: a decade of progress. Nat Rev Drug Discov. 2017;16:115-30.
39. Kamdar F, Das S, Gong W, et al. Stem cell-derived cardiomyocytes and beta-adrenergic receptor blockade in Duchenne muscular dystrophy cardiomyopathy. J Am Coll Cardiol. 2020;75:1159-74.
40. Dick E, Kalra S, Anderson D, et al. Exon skipping and gene transfer restore dystrophin expression in hiPSC-cardiomyocytes harbouring DMD mutations. Stem Cells Dev. ;2013:150127064140000.
41. Guan X, Mack DL, Moreno CM, et al. Dystrophin-deficient cardiomyocytes derived from human urine: new biologic reagents for drug discovery. Stem Cell Res. 2014;12:467-80.
42. Pioner JM, Guan X, Klaiman JM, et al. Absence of full-length dystrophin impairs normal maturation and contraction of cardiomyocytes derived from human-induced pluripotent stem cells. Cardiovasc Res. 2020;116:368-82.
43. Chang ACY, Pardon G, Chang ACH, et al. Increased tissue stiffness triggers contractile dysfunction and telomere shortening in dystrophic cardiomyocytes. Stem Cell Rep. 2021;16:2169-81.
44. Young CS, Hicks MR, Ermolova NV, et al. A Single CRISPR-Cas9 deletion strategy that targets the majority of DMD patients restores dystrophin function in hiPSC-derived muscle cells. Cell Stem Cell. 2016;18:533-40.
45. Atmanli A, Chai AC, Cui M, et al. Cardiac Myoediting attenuates cardiac abnormalities in human and mouse models of Duchenne muscular dystrophy. Circ Res. 2021;129:602-16.
46. Min YL, Li H, Rodriguez-Caycedo C, et al. CRISPR-Cas9 corrects Duchenne muscular dystrophy Exon 44 deletion mutations in mice and human cells. Sci Adv. 2019;5:eaav4324.
47. Zhang Y, Li Y, Hu Q, et al. The lncRNA H19 alleviates muscular dystrophy by stabilizing dystrophin. Nat Cell Biol. 2020;22:1332-45.
48. Eguchi A, Gonzalez AFGS, Torres-Bigio SI, et al. TRF2 rescues telomere attrition and prolongs cell survival in Duchenne muscular dystrophy cardiomyocytes derived from human iPSCs. Proc Natl Acad Sci U S A. 2023;120:e2209967120.
49. Hofbauer P, Jahnel SM, Papai N, et al. Cardioids reveal self-organizing principles of human cardiogenesis. Cell. 2021;184:3299-3317.e22.
50. Richards DJ, Coyle RC, Tan Y, et al. Inspiration from heart development: Biomimetic development of functional human cardiac organoids. Biomaterials. 2017;142:112-23.
51. Mavrommatis L, Jeong HW, Kindler U, et al. Human skeletal muscle organoids model fetal myogenesis and sustain uncommitted PAX7 myogenic progenitors. Elife. 2023:12.
52. Shahriyari M, Islam MR, Sakib SM, et al. Engineered skeletal muscle recapitulates human muscle development, regeneration and dystrophy. J Cachexia Sarcopenia Muscle. 2022;13:3106-21.
53. ‘t Groen SLM, Franken M, Bock T, Krüger M, de Greef JC, Pijnappel WWMP. A knock down strategy for rapid, generic, and versatile modelling of muscular dystrophies in 3D-tissue-engineered-skeletal muscle. Skelet Muscle. 2024;14:3.
54. Long C, Li H, Tiburcy M, et al. Correction of diverse muscular dystrophy mutations in human engineered heart muscle by single-site genome editing. Sci Adv. 2018;4:eaap9004.
55. Tejedera-Villafranca A, Montolio M, Ramón-Azcón J, Fernández-Costa JM. Mimicking sarcolemmal damagein vitro: a contractile 3D model of skeletal muscle for drug testing in Duchenne muscular dystrophy. Biofabrication. 2023;15:045024.
56. Sheikh O, Yokota T. Developing DMD therapeutics: a review of the effectiveness of small molecules, stop-codon readthrough, dystrophin gene replacement, and exon-skipping therapies. Expert Opin Investig Drugs. 2021;30:167-76.
57. Namgoong JH, Bertoni C. Clinical potential of ataluren in the treatment of Duchenne muscular dystrophy. Degener Neurol Neuromuscul Dis. 2016;6:37-48.
58. Michorowska S. Ataluren-promising therapeutic premature termination codon readthrough frontrunner. Pharmaceuticals (Basel). 2021;14:785.
59. Wagner KR, Hamed S, Hadley DW, et al. Gentamicin treatment of Duchenne and Becker muscular dystrophy due to nonsense mutations. Ann Neurol. 2001;49:706-11.
60. Politano L. Read-through approach for stop mutations in Duchenne muscular dystrophy. An update. Acta Myol. 2021;40:43-50.
61. Lu QL, Yokota T, Takeda S, Garcia L, Muntoni F, Partridge T. The status of exon skipping as a therapeutic approach to Duchenne muscular dystrophy. Mol Ther. 2011;19:9-15.
62. Desjardins CA, Yao M, Hall J, et al. Enhanced exon skipping and prolonged dystrophin restoration achieved by TfR1-targeted delivery of antisense oligonucleotide using FORCE conjugation in mdx mice. Nucleic Acids Res. 2022;50:11401-14.
63. Niks EH, Aartsma-Rus A. Exon skipping: a first in class strategy for Duchenne muscular dystrophy. Expert Opin Biol Ther. 2017;17:225-36.
64. Sang A, Zhuo S, Bochanis A, et al. Mechanisms of action of the US food and drug administration-approved antisense oligonucleotide drugs. BioDrugs. 2024;38:511-26.
67. Vincik LY, Dautel AD, Staples AA, et al. Evolving role of viltolarsen for treatment of Duchenne muscular dystrophy. Adv Ther. 2024;41:1338-50.
69. Duan D. Systemic AAV micro-dystrophin gene therapy for Duchenne muscular dystrophy. Mol Ther. 2018;26:2337-56.
70. Le Guiner C, Servais L, Montus M, et al. Long-term microdystrophin gene therapy is effective in a canine model of Duchenne muscular dystrophy. Nat Commun. 2017;8:16105.
71. Potter RA, Griffin DA, Heller KN, et al. Dose-escalation study of systemically delivered rAAVrh74.MHCK7.micro-dystrophin in the mdx mouse model of Duchenne muscular dystrophy. Hum Gene Ther. 2021;32:375-89.
72. Lai Y, Thomas GD, Yue Y, et al. Dystrophins carrying spectrin-like repeats 16 and 17 anchor nNOS to the sarcolemma and enhance exercise performance in a mouse model of muscular dystrophy. J Clin Invest. 2009;119:624-35.
73. Mendell JR, Sahenk Z, Lehman K, et al. Assessment of systemic delivery of rAAVrh74.MHCK7.micro-dystrophin in children with Duchenne muscular dystrophy: a nonrandomized controlled trial. JAMA Neurol. 2020;77:1122-31.
75. Muhuri M, Levy DI, Schulz M, McCarty D, Gao G. Durability of transgene expression after rAAV gene therapy. Mol Ther. 2022;30:1364-80.
76. Le Hir M, Goyenvalle A, Peccate C, et al. AAV genome loss from dystrophic mouse muscles during AAV-U7 snRNA-mediated exon-skipping therapy. Mol Ther. 2013;21:1551-8.
77. Kachanov A, Kostyusheva A, Brezgin S, et al. The menace of severe adverse events and deaths associated with viral gene therapy and its potential solution. Med Res Rev. 2024;44:2112-93.
78. Bönnemann CG, Belluscio BA, Braun S, Morris C, Singh T, Muntoni F. Dystrophin immunity after gene therapy for Duchenne’s muscular dystrophy. N Engl J Med. 2023;388:2294-6.
79. Lek A, Atas E, Hesterlee SE, Byrne BJ, Bönnemann CG. Meeting report: 2022 muscular dystrophy association summit on ‘safety and challenges in gene transfer therapy’. J Neuromuscul Dis. 2023;10:327-36.
80. after high-dose rAAV9 gene therapy in a patient with Duchenne’s muscular dystrophy. N Engl J Med. 2023;389:2210-1.
81. Mendell JR, Campbell K, Rodino-Klapac L, et al. Dystrophin immunity in Duchenne’s muscular dystrophy. N Engl J Med. 2010;363:1429-37.
83. Xie Q, Chen X, Ma H, et al. Improved gene therapy for spinal muscular atrophy in mice using codon-optimized hSMN1 transgene and hSMN1 gene-derived promotor. EMBO Mol Med. 2024;16:945-65.
84. Laurent M, Geoffroy M, Pavani G, Guiraud S. CRISPR-based gene therapies: from preclinical to clinical treatments. Cells. 2024;13:800.
85. Tabebordbar M, Zhu K, Cheng JKW, et al. In vivo gene editing in dystrophic mouse muscle and muscle stem cells. Science. 2016;351:407-11.
86. Long C, Amoasii L, Mireault AA, et al. Postnatal genome editing partially restores dystrophin expression in a mouse model of muscular dystrophy. Science. 2016;351:400-3.
87. Porto EM, Komor AC, Slaymaker IM, Yeo GW. Base editing: advances and therapeutic opportunities. Nat Rev Drug Discov. 2020;19:839-59.
88. Chemello F, Chai AC, Li H, et al. Precise correction of Duchenne muscular dystrophy exon deletion mutations by base and prime editing. Sci Adv 2021;7.
89. Ryu SM, Koo T, Kim K, et al. Adenine base editing in mouse embryos and an adult mouse model of Duchenne muscular dystrophy. Nat Biotechnol. 2018;36:536-9.
90. Lin J, Jin M, Yang D, et al. Adenine base editing-mediated exon skipping restores dystrophin in humanized Duchenne mouse model. Nat Commun. 2024;15:5927.
91. Xu L, Zhang C, Li H, et al. Efficient precise in vivo base editing in adult dystrophic mice. Nat Commun. 2021;12:3719.
92. Anzalone AV, Randolph PB, Davis JR, et al. Search-and-replace genome editing without double-strand breaks or donor DNA. Nature. 2019;576:149-57.
93. Lek A, Wong B, Keeler A et al. Unexpected death of a Duchenne muscular dystrophy patient in an N-of-1 trial of rAAV9-delivered CRISPR-transactivator. medRxiv. ;2023:2023.05.16.23289881.
94. Nitahara-Kasahara Y, Nakayama S, Kimura K, et al. Immunomodulatory amnion-derived mesenchymal stromal cells preserve muscle function in a mouse model of Duchenne muscular dystrophy. Stem Cell Res Ther. 2023;14:108.
95. Xiao R, Zhou M, Wang P, et al. Full-length dystrophin restoration via targeted exon addition in DMD-patient specific iPSCs and cardiomyocytes. Int J Mol Sci. 2022;23:9176.
96. Lenardič A, Domenig SA, Zvick J, et al. Generation of allogeneic and xenogeneic functional muscle stem cells for intramuscular transplantation. J Clin Invest. 2024:134.
97. Dhoke NR, Kim H, Azzag K, Crist SB, Kiley J, Perlingeiro RCR. A novel CRISPR-Cas9 strategy to target DYSTROPHIN mutations downstream of Exon 44 in patient-specific DMD iPSCs. Cells. 2024;13:972.
98. McDonald CM, Marbán E, Hendrix S, et al. HOPE-2 Study Group. Repeated intravenous cardiosphere-derived cell therapy in late-stage Duchenne muscular dystrophy (HOPE-2): a multicentre, randomised, double-blind, placebo-controlled, phase 2 trial. Lancet. 2022;399:1049-58.
99. Rogers RG, Fournier M, Sanchez L, et al. Disease-modifying bioactivity of intravenous cardiosphere-derived cells and exosomes in mdx mice. JCI Insight. 2019;4:130202.
100. Budzynska K, Siemionow M, Stawarz K, Chambily L, Siemionow K. Chimeric cell therapies as a novel approach for Duchenne muscular dystrophy (DMD) and muscle regeneration. Biomolecules. 2024;14:575.
101. Tinsley JM, Blake DJ, Roche A, et al. Primary structure of dystrophin-related protein. Nature. 1992;360:591-3.
102. Szwec S, Kapłucha Z, Chamberlain JS, Konieczny P. Dystrophin- and utrophin-based therapeutic approaches for treatment of Duchenne muscular dystrophy: A comparative review. BioDrugs. 2024;38:95-119.
103. Li D, Bareja A, Judge L, et al. Sarcolemmal nNOS anchoring reveals a qualitative difference between dystrophin and utrophin. J Cell Sci. 2010;123:2008-13.
104. Belanto JJ, Mader TL, Eckhoff MD, et al. Microtubule binding distinguishes dystrophin from utrophin. Proc Natl Acad Sci U S A. 2014;111:5723-8.
105. Miura P, Jasmin BJ. Utrophin upregulation for treating Duchenne or Becker muscular dystrophy: how close are we? Trends Mol Med. 2006;12:122-9.
106. Falcucci L, Dooley CM, Adamoski D, et al. Transcriptional adaptation upregulates utrophin in Duchenne muscular dystrophy. Nature. 2025;639:493-502.
107. Tinsley J, Deconinck N, Fisher R, et al. Expression of full-length utrophin prevents muscular dystrophy in mdx mice. Nat Med. 1998;4:1441-4.
108. Wilkinson IVL, Perkins KJ, Dugdale H, et al. Chemical proteomics and phenotypic profiling identifies the aryl hydrocarbon receptor as a molecular target of the utrophin modulator ezutromid. Angew Chem Int Ed Engl. 2020;59:2420-8.
109. Guiraud S, Squire SE, Edwards B, et al. Second-generation compound for the modulation of utrophin in the therapy of DMD. Hum Mol Genet. 2015;24:4212-24.
110. Tinsley JM, Potter AC, Phelps SR, Fisher R, Trickett JI, Davies KE. Amelioration of the dystrophic phenotype of mdx mice using a truncated utrophin transgene. Nature. 1996;384:349-53.
111. Odom GL, Gregorevic P, Allen JM, Finn E, Chamberlain JS. Microutrophin delivery through rAAV6 increases lifespan and improves muscle function in dystrophic dystrophin/utrophin-deficient mice. Mol Ther. 2008;16:1539-45.
112. Liao HK, Hatanaka F, Araoka T, et al. In vivo target gene activation via CRISPR/Cas9-mediated trans-epigenetic modulation. Cell. 2017;171:1495-1507.e15.
113. Sengupta K, Mishra MK, Loro E, Spencer MJ, Pyle AD, Khurana TS. Genome editing-mediated utrophin upregulation in Duchenne muscular dystrophy stem cells. Mol Ther Nucleic Acids. 2020;22:500-9.
114. Heier CR, Yu Q, Fiorillo AA, et al. Vamorolone targets dual nuclear receptors to treat inflammation and dystrophic cardiomyopathy. Life Sci Alliance. 2019;2:e201800186.
115. Liu X, Wang Y, Gutierrez JS, et al. Disruption of a key ligand-H-bond network drives dissociative properties in Vamorolone for Duchenne muscular dystrophy treatment. Proc Natl Acad Sci U S A. 2020;117:24285-93.
117. Tidball JG, Welc SS, Wehling‐Henricks M. Immunobiology of inherited muscular dystrophies. Compr Physiol. 2011;8:1313-56.
118. Villalta SA, Nguyen HX, Deng B, Gotoh T, Tidball JG. Shifts in macrophage phenotypes and macrophage competition for arginine metabolism affect the severity of muscle pathology in muscular dystrophy. Hum Mol Genet. 2009;18:482-96.
119. Perandini LA, Chimin P, Lutkemeyer DDS, Câmara NOS. Chronic inflammation in skeletal muscle impairs satellite cells function during regeneration: can physical exercise restore the satellite cell niche? FEBS J. 2018;285:1973-84.
120. Villalta SA, Rinaldi C, Deng B, Liu G, Fedor B, Tidball JG. Interleukin-10 reduces the pathology of mdx muscular dystrophy by deactivating M1 macrophages and modulating macrophage phenotype. Hum Mol Genet. 2011;20:790-805.
121. Villalta SA, Rosenthal W, Martinez L, et al. Regulatory T cells suppress muscle inflammation and injury in muscular dystrophy. Sci Transl Med. 2014;6:258ra142.
122. Rossi G, Antonini S, Bonfanti C, et al. Nfix regulates temporal progression of muscle regeneration through modulation of myostatin expression. Cell Re. ;14:2238-49.
123. Saclier M, Angelini G, Bonfanti C, Mura G, Temponi G, Messina G. Selective ablation of Nfix in macrophages attenuates muscular dystrophy by inhibiting fibro-adipogenic progenitor-dependent fibrosis. J Pathol. 2022;257:352-66.
124. Rossi G, Bonfanti C, Antonini S, et al. Silencing Nfix rescues muscular dystrophy by delaying muscle regeneration. Nat Commun. 2017;8:1055.
125. Babaeijandaghi F, Cheng R, Kajabadi N, et al. Metabolic reprogramming of skeletal muscle by resident macrophages points to CSF1R inhibitors as muscular dystrophy therapeutics. Sci Transl Med. 2022;14:eabg7504.
126. Colussi C, Mozzetta C, Gurtner A, et al. HDAC2 blockade by nitric oxide and histone deacetylase inhibitors reveals a common target in Duchenne muscular dystrophy treatment. Proc Natl Acad Sci U S A. 2008;105:19183-7.
128. Farini A, Tripodi L, Villa C, et al. Microbiota dysbiosis influences immune system and muscle pathophysiology of dystrophin-deficient mice. EMBO Mol Med. 2023;15:e16244.
129. Kalkan H, Pagano E, Paris D, et al. Targeting gut dysbiosis against inflammation and impaired autophagy in Duchenne muscular dystrophy. EMBO Mol Med. 2023;15:e16225.
130. Zong Y, Li H, Liao P, et al. Mitochondrial dysfunction: mechanisms and advances in therapy. Signal Transduct Target Ther. 2024;9:124.
131. Sun M, Jiang W, Mu N, Zhang Z, Yu L, Ma H. Mitochondrial transplantation as a novel therapeutic strategy for cardiovascular diseases. J Transl Med. 2023;21:347.
132. Sun X, Gao R, Li W, et al. Alda-1 treatment promotes the therapeutic effect of mitochondrial transplantation for myocardial ischemia-reperfusion injury. Bioact Mater. 2021;6:2058-69.
133. Moskowitzova K, Shin B, Liu K, et al. Mitochondrial transplantation prolongs cold ischemia time in murine heart transplantation. J Heart Lung Transplant. 2019;38:92-9.
134. Ikeda G, Santoso MR, Tada Y, et al. Mitochondria-rich extracellular vesicles from autologous stem cell-derived cardiomyocytes restore energetics of ischemic myocardium. J Am Coll Cardiol. 2021;77:1073-88.
135. Masuzawa A, Black KM, Pacak CA, et al. Transplantation of autologously derived mitochondria protects the heart from ischemia-reperfusion injury. Am J Physiol Heart Circ Physiol. 2013;304:H966-82.
136. O’Brien CG, Ozen MO, Ikeda G, et al. Mitochondria-rich extracellular vesicles rescue patient-specific cardiomyocytes from doxorubicin injury: insights into the SENECA trial. JACC CardioOncol. 2021;3:428-40.
137. Lin RZ, Im GB, Luo AC, et al. Mitochondrial transfer mediates endothelial cell engraftment through mitophagy. Nature. 2024;629:660-8.
138. Yang X, Zhou P, Zhao Z, et al. Improvement effect of mitotherapy on the cognitive ability of Alzheimer’s Disease through NAD+/SIRT1-mediated autophagy. Antioxidants (Basel). 2023;12:2006.
139. Zhao J, Qu D, Xi Z, et al. Mitochondria transplantation protects traumatic brain injury via promoting neuronal survival and astrocytic BDNF. Transl Res. 2021;235:102-14.
140. Siemionow M, Bocian K, Bozyk KT, Ziemiecka A, Siemionow K. Chimeric cell therapy transfers healthy donor mitochondria in Duchenne muscular dystrophy. Stem Cell Rev Rep. 2024;20:1819-29.
141. Zhang A, Liu Y, Pan J, et al. Delivery of mitochondria confers cardioprotection through mitochondria replenishment and metabolic compliance. Mol Ther. 2023;31:1468-79.
142. Main EN, Cruz TM, Bowlin GL. Mitochondria as a therapeutic: a potential new frontier in driving the shift from tissue repair to regeneration. Regen Biomate. ;10:rbad070.
143. Zhao Z, Yu Z, Hou Y, Zhang L, Fu A. Improvement of cognitive and motor performance with mitotherapy in aged mice. Int J Biol Sci. 2020;16:849-58.
144. Kim MJ, Lee JM, Min K, Choi YS. Xenogeneic transplantation of mitochondria induces muscle regeneration in an in vivo rat model of dexamethasone-induced atrophy. J Muscle Res Cell Motil. 2024;45:53-68.
145. Wu Z, Chen L, Guo W, et al. Oral mitochondrial transplantation using nanomotors to treat ischaemic heart disease. Nat Nanotechnol. 2024;19:1375-85.
146. Alexander JF, Seua AV, Arroyo LD, et al. Nasal administration of mitochondria reverses chemotherapy-induced cognitive deficits. Theranostics. 2021;11:3109-30.
147. Hughes MC, Ramos SV, Turnbull PC, et al. Impairments in left ventricular mitochondrial bioenergetics precede overt cardiac dysfunction and remodelling in Duchenne muscular dystrophy. J Physiol. 2020;598:1377-92.
148. Murphy MP, Smith RA. Targeting antioxidants to mitochondria by conjugation to lipophilic cations. Annu Rev Pharmacol Toxicol. 2007;47:629-56.
149. Siegel MP, Kruse SE, Percival JM, et al. Mitochondrial-targeted peptide rapidly improves mitochondrial energetics and skeletal muscle performance in aged mice. Aging Cell. 2013;12:763-71.
150. Li B, Xiong W, Liang WM, Chiou JS, Lin YJ, Chang ACY. Targeting of CAT and VCAM1 as novel therapeutic targets for DMD cardiomyopathy. Front Cell Dev Biol. 2021;9:659177.
151. Kyrychenko V, Poláková E, Janíček R, Shirokova N. Mitochondrial dysfunctions during progression of dystrophic cardiomyopathy. Cell Calcium. 2015;58:186-95.
152. Dubinin MV, Talanov EY, Tenkov KS, Starinets VS, Mikheeva IB, Belosludtsev KN. Transport of Ca2+ and Ca2+-dependent permeability transition in heart mitochondria in the early stages of Duchenne muscular dystrophy. Biochim Biophys Acta Bioenerg. 2020;1861:148250.
153. Willi L, Abramovich I, Fernandez-Garcia J, et al. Bioenergetic and metabolic impairments in induced pluripotent stem cell-derived cardiomyocytes generated from Duchenne muscular dystrophy patients. Int J Mol Sci. 2022;23:9808.
154. Kuno A, Hosoda R, Sebori R, et al. Resveratrol ameliorates mitophagy disturbance and improves cardiac pathophysiology of dystrophin-deficient mdx mice. Sci Rep. 2018;8:15555.
155. Pauly M, Daussin F, Burelle Y, et al. AMPK activation stimulates autophagy and ameliorates muscular dystrophy in the mdx mouse diaphragm. Am J Pathol. 2012;181:583-92.
156. Ljubicic V, Jasmin BJ. Metformin increases peroxisome proliferator-activated receptor γ Co-activator-1α and utrophin a expression in dystrophic skeletal muscle. Muscle Nerve. 2015;52:139-42.
157. Ljubicic V, Burt M, Lunde JA, Jasmin BJ. Resveratrol induces expression of the slow, oxidative phenotype in mdx mouse muscle together with enhanced activity of the SIRT1-PGC-1α axis. Am J Physiol Cell Physiol. 2014;307:C66-82.
158. Hollinger K, Shanely RA, Quindry JC, Selsby JT. Long-term quercetin dietary enrichment decreases muscle injury in mdx mice. Clin Nutr. 2015;34:515-22.
159. Suntar I, Sureda A, Belwal T, et al. Natural products, PGC-1 α , and Duchenne muscular dystrophy. Acta Pharm Sin B. 2020;10:734-45.
160. Allen DG, Whitehead NP, Froehner SC. Absence of dystrophin disrupts skeletal muscle signaling: roles of Ca2+, reactive oxygen species, and nitric oxide in the development of muscular dystrophy. Physiol Rev. 2016;96:253-305.
161. Kyrychenko S, Poláková E, Kang C, et al. Hierarchical accumulation of RyR post-translational modifications drives disease progression in dystrophic cardiomyopathy. Cardiovasc Res. 2013;97:666-75.
162. Fauconnier J, Thireau J, Reiken S, et al. Leaky RyR2 trigger ventricular arrhythmias in Duchenne muscular dystrophy. Proc Natl Acad Sci U S A. 2010;107:1559-64.
163. Zhang SS, Zhou S, Crowley-McHattan ZJ, Wang RY, Li JP. A review of the role of endo/sarcoplasmic reticulum-mitochondria Ca2+ transport in diseases and skeletal muscle function. Int J Environ Res Public Health. 2021;18:3874.
165. Santulli G, Xie W, Reiken SR, Marks AR. Mitochondrial calcium overload is a key determinant in heart failure. Proc Natl Acad Sci U S A. 2015;112:11389-94.
166. Zabłocka B, Górecki DC, Zabłocki K. Disrupted calcium homeostasis in Duchenne muscular dystrophy: a common mechanism behind diverse consequences. Int J Mol Sci. 2021;22:11040.
167. Souidi M, Resta J, Dridi H, et al. Ryanodine receptor dysfunction causes senescence and fibrosis in Duchenne dilated cardiomyopathy. J Cachexia Sarcopenia Muscle. 2024;15:536-51.
168. Goonasekera SA, Lam CK, Millay DP, et al. Mitigation of muscular dystrophy in mice by SERCA overexpression in skeletal muscle. J Clin Invest. 2011;121:1044-52.
169. Kodippili K, Hakim CH, Burke MJ, et al. SERCA2a overexpression improves muscle function in a canine Duchenne muscular dystrophy model. Mol Ther Methods Clin Dev. 2024;32:101268.
170. Balakrishnan R, Mareedu S, Babu GJ. Reducing sarcolipin expression improves muscle metabolism in mdx mice. Am J Physiol Cell Physiol. 2022;322:C260-74.
171. Liu Z, Cai H, Dang Y, Qiu C, Wang J. Adenosine triphosphate-sensitive potassium channels and cardiomyopathies (review). Mol Med Rep. 2016;13:1447-54.
172. Graciotti L, Becker J, Granata AL, Procopio AD, Tessarollo L, Fulgenzi G. Dystrophin is required for the normal function of the cardio-protective KATP channel in cardiomyocytes. PLoS One. 2011;6:e27034.
173. Bienengraeber M, Olson TM, Selivanov VA, et al. ABCC9 mutations identified in human dilated cardiomyopathy disrupt catalytic KATP channel gating. Nat Genet. 2004;36:382-7.
174. Farid TA, Nair K, Massé S, et al. Role of KATP channels in the maintenance of ventricular fibrillation in cardiomyopathic human hearts. Circ Res. 2011;109:1309-18.
175. Rubi L, Koenig X, Kubista H, Todt H, Hilber K. Decreased inward rectifier potassium current IK1 in dystrophin-deficient ventricular cardiomyocytes. Channels (Austin). 2017;11:101-8.
176. Pacioretty LM, Cooper BJ, Gilmour RF Jr. Reduction of the transient outward potassium current in canine X-linked muscular dystrophy. Circulation. 1994;90:1350-6.
177. Fatima S, Zhou H, Chen Y, Liu Q. Role of ferroptosis in the pathogenesis of heart disease. Front Physiol. 2024;15:1450656.
178. Gujja P, Rosing DR, Tripodi DJ, Shizukuda Y. Iron overload cardiomyopathy: better understanding of an increasing disorder. J Am Coll Cardiol. 2010;56:1001-12.
179. Wansapura JP, Hor KN, Mazur W, et al. Left ventricular T2 distribution in Duchenne muscular dystrophy. J Cardiovasc Magn Reson. 2010;12:14.
180. Andrysiak K, Machaj G, Priesmann D, et al. Dysregulated iron homeostasis in dystrophin-deficient cardiomyocytes: correction by gene editing and pharmacological treatment. Cardiovasc Res. 2024;120:69-81.
181. Furihata T, Takada S, Kakutani N, et al. Cardiac-specific loss of mitoNEET expression is linked with age-related heart failure. Commun Biol. 2021;4:138.
182. Alves FM, Kysenius K, Caldow MK, et al. Iron overload and impaired iron handling contribute to the dystrophic pathology in models of Duchenne muscular dystrophy. J Cachexia Sarcopenia Muscle. 2022;13:1541-53.
183. Bizot F, Goossens R, Tensorer T, et al. Histone deacetylase inhibitors improve antisense-mediated exon-skipping efficacy in mdx mice. Mol Ther Nucleic Acids. 2022;30:606-20.
184. Barthélémy F, Wang RT, Hsu C, et al. Targeting RyR activity boosts antisense exon 44 and 45 skipping in human DMD skeletal or cardiac muscle culture models. Mol Ther Nucleic Acids. 2019;18:580-9.
185. Kendall GC, Mokhonova EI, Moran M, et al. Dantrolene enhances antisense-mediated exon skipping in human and mouse models of Duchenne muscular dystrophy. Sci Transl Med. 2012;4:164ra160.
186. Hayashita-Kinoh H, Guillermo PH, Nitahara-Kasahara Y, et al. Improved transduction of canine X-linked muscular dystrophy with rAAV9-microdystrophin via multipotent MSC pretreatment. Mol Ther Methods Clin Dev. 2021;20:133-41.
187. Mishra MK, Loro E, Sengupta K, Wilton SD, Khurana TS. Functional improvement of dystrophic muscle by repression of utrophin: let-7c interaction. PLoS One. 2017;12:e0182676.
188. Cacchiarelli D, Incitti T, Martone J, et al. miR-31 modulates dystrophin expression: new implications for Duchenne muscular dystrophy therapy. EMBO Rep. 2011;12:136-41.
189. Peccate C, Mollard A, Le Hir M, et al. Antisense pre-treatment increases gene therapy efficacy in dystrophic muscles. Hum Mol Genet. 2016;25:3555-63.
190. Blitek M, Gastaldi C, Doisy M, et al. Combined 20-hydroxyecdysone and antisense-mediated exon skipping improve functional outcomes in a mouse model of Duchenne muscular dystrophy. Nucleic Acid Ther. 2025; doi: 10.1089/nat.2024.0085.
191. Cervia D, Zecchini S, Pincigher L, et al. Oral administration of plumbagin is beneficial in in vivo models of Duchenne muscular dystrophy through control of redox signaling. Free Radic Biol Med. 2024;225:193-207.
192. Villa C, Secchi V, Macchi M, et al. Magnetic-field-driven targeting of exosomes modulates immune and metabolic changes in dystrophic muscle. Nat Nanotechnol. 2024;19:1532-43.
Cite This Article

How to Cite
Download Citation
Export Citation File:
Type of Import
Tips on Downloading Citation
Citation Manager File Format
Type of Import
Direct Import: When the Direct Import option is selected (the default state), a dialogue box will give you the option to Save or Open the downloaded citation data. Choosing Open will either launch your citation manager or give you a choice of applications with which to use the metadata. The Save option saves the file locally for later use.
Indirect Import: When the Indirect Import option is selected, the metadata is displayed and may be copied and pasted as needed.
About This Article
Special Issue
Copyright
Data & Comments
Data
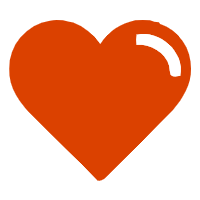
Comments
Comments must be written in English. Spam, offensive content, impersonation, and private information will not be permitted. If any comment is reported and identified as inappropriate content by OAE staff, the comment will be removed without notice. If you have any queries or need any help, please contact us at [email protected].