Nitrogen sulfur dual-doped porous biochar fibers for high performance lithium-sulfur battery
Abstract
Porous carbons have gained great attention for applications in lithium-sulfur (Li-S) batteries. However, achieving high specific surface area, hierarchical porosity, and abundant heteroatom-doping with facile approaches is still challenging. Herein, nitrogen, sulfur dual-doped porous biochar fibers (PBF@N@S) are obtained via a simple and sustainable activation process of cotton fibers. The as-prepared PBF@N@S exhibits a hierarchically interconnected network porous structure and large specific surface area. Meanwhile, abundant nitrogen, sulfur atoms are simultaneously doped in the carbons. These characteristics make the carbon favorable for hosting sulfur. The PBF@N@S sample with 50 wt% sulfur content (PBF@N@S-S-50%) delivers a high initial capacity with excellent cycling performance. Such high performance suggests that the PBF@N@S-S could be a promising cathode material for Li-S batteries.
Keywords
INTRODUCTION
Rechargeable lithium (Li) batteries have played an extraordinary role in portable electronics[1-3]. In the 1990s, Li-ion batteries (LIBs) were successfully commercialized and dominated the energy storage market, led by Sony Company[4]. However, traditional LIBs are reaching their theoretical limits, and there are still challenges in many aspects, such as low specific capacity and energy density, cost, and safety[5,6]. Over the past few years, multielectron reactions have drawn lots of attention as an efficient way for designing and developing high-performance batteries. Particularly, compared to traditional LIBs, Li-sulfur (Li-S) batteries have attracted lots of interest because of their remarkable specific capacity (1,675 mAh/g) and high energy density (2,500 wh/kg)[7-9]. In addition, S, as the active material of cathodes, has plenty of advantages, such as cheap, natural abundance, and eco-friendly[10,11]. Moreover, Li-S batteries offer more safety than commercialized Li-ion batteries because the highly reactive Li anode is passivated with sulfide during operation. As a result, Li-S batteries have attracted more interest and have been considered as one of the most popular candidates for energy storage.
Unfortunately, up to now, the practical application of Li-S batteries is still obstructed by three unsolved issues: (1) the insulating nature of sulfur and lithium sulfides (5 × 10-30 S/cm at 25 ℃); (2) the “shuttle effect” occurred between cathode and lithium anode causing irreversible loss of S; (3) the significant changes of structural and volume in the charge/discharge process[12,13]. To address the above issues, a number of strategies have been made. Among them, incorporating S with highly conductive carbon materials, such as porous carbon, graphene, and carbon nanotubes, is one of the most promising strategies for
The porous biochar prepared from biomass is attracting increasing attention due to its cheap, environmentally friendly, and sustainable development. Up to now, various porous biochars have been reported as S hosts for Li-S batteries[26,27]. As a natural biomass, cotton has been widely used in daily life due to its excellent sustainability.
In this work, we proposed a simple yet effective strategy to turn biomass cotton into heteroatom-doped porous biochar fibers with a hierarchical pore structure. Typically, N, S dual-doped porous biochar fibers (PBF@N@S) were prepared through a simple one-step potassium hydroxide (KOH) activation method. The as-prepared carbons have a unique interconnected porous structure with N, S co-doping, which is beneficial to the impregnation of S. The as-fabricated S/PBF@N@S composite electrode exhibited an excellent specific capacity with high cycling performance.
EXPERIMENTAL
Materials and apparatus
Cystine, KOH, melamine, and nmethyl-2-pyrrolidene (NMP) solution was supplied by Sinopharm Chemical Reagent Co., Ltd. (China). X-ray diffraction (XRD) patterns were tested on a Rigaku Smartlab (9) diffractometer with Cu Kα radiation. The testing range of two-theta was from 5° to 90°, and the scanning rate of 10°/min with a step of 0.02°. Scanning electronic microscopy (SEM) images were obtained with a Hitachi SU8020 electron microscopy. Transmission electron microscopy (TEM) measurements were performed with a JEOL JEM-2100F field emission transmission electron microscope. Raman spectra were obtained using a Renishaw INVIA Raman spectrometer. Gas adsorption/desorption isotherms were obtained from a Quadrasorb SIMP analyzer at the liquid nitrogen temperature. The specific surface area of the carbons was calculated using the Brunauer-Emmett-Teller (BET) equation. X-ray photoelectron spectroscopy (XPS) measurements were conducted on Thermo Fisher Scientific ESCALAB 250Xi equipment. All spectra were calibrated with the binding energy of the C1s peak at 284.8 eV.
Preparation of PBF@N@S
The preparation of PBF@N@S is shown in Figure 1. Firstly, the cotton was cleaned and cut into cotton patches using scissors and ground into fine fibers. Cystine was used as N and S sources, and the fine cotton fibers were mixed with KOH/cystine at 60 ℃ for three days with the weight ratio of 1:5. After drying in an oven, pyrolysis was conducted by heating under flowing argon at 800 ℃ with a heating rate of 10 ℃/min with a hold period of 1 h at 800 ℃. For comparison, N-doped porous biochar fibers (PBF@N) were obtained as the same process but using melamine as a N source. In addition, bare biochar fibers (PBF) were obtained by directly carbonizing fine cotton fibers without the use of KOH, cystine, and melamine. Subsequently, all the carbonized products were cleaned using deionized water and anhydrous ethanol.
Electrode preparation and electrochemical measurements
The cathode slurry was prepared by mixing as-prepared carbons (PBF, PBF@N, or PBF@N@S), S with polyvinylidene fluoride (PVDF), and carbon black at a mass ratio of 8:1:1 in NMP solution. The mixed slurry was then coated on an aluminum foil and vacuum dried at 60 oC overnight. The S loading is the same mass ratio as carbons (1:1 mass ratio of S to carbon, named PBF-S-50%, PBF@N-S-50%, and
RESULTS AND DISCUSSION
Characterization of PBFs
Figure 2A shows the XRD patterns of the as-prepared porous carbon samples. All samples show two broad peaks, located at around 25o and 43o two-theta, which are assigned to the reflection of the graphite (002) and (101) planes (JCPDS Card File, No. 41-1487), respectively[23]. No other observable peaks due to impurities have appeared. In addition, as it can be seen, the graphite peak of PBF@N@S is sharper, particularly than the others, which proves that PBF@N@S has better crystallization. Raman spectra are exhibited in Figure 2B, two characteristic peaks at around 1,350 and 1,600 cm-1 can be observed, corresponding to the D and G bands of carbon, respectively[28]. The ratio of the D band to the G band (ID/IG) is usually used to evaluate the density of defective sites[23]. The ID/IGratio is 1.11 for PBF@N@S, 1.01 for PBF@N, and 0.98 for PBF, respectively, indicating that the defect density increased. The improved defect density would lead to more electrochemical active sites[23].
Figure 2. (A) XRD patterns of the PBF, PBF@N, and PBF@N@S and (B) Raman spectra of PBF, PBF@N, and PBF@N@S. PBF@N: N-doped porous biochar fibers; PBF@N@S: N, S dual-doped porous biochar fibers; XRD: X-ray diffraction.
N adsorption/desorption isotherms are used to characterize the porosity of the samples, as illustrated in Figure 3A, and the corresponding pore size distribution is shown in Figure 3B-C. As shown in Figure 3A, the PBF@N@S shows a combination of Type I and II isotherms with an obvious H4 hysteresis loop, indicating that both micropores and mesopores exist. PBF@N also shows a composite of type I and II isotherms but with a small hysteresis loop, indicating a micropore dominant characteristic. PBF shows poor gas adsorption-desorption properties. The calculated BET surface area is 1,696 m2/g, 1,342 m2/g, and
Figure 3. N2 adsorption/desorption isotherms of PBF, PBF@N, and PBF@N@S (A) and pore size distribution of the PBF@N@S (B) and PBF@N (C). PBF: Bare biochar fibers; PBF@N: N-doped porous biochar fibers; PBF@N@S: N, S dual-doped porous biochar fibers.
Physical characteristics of PBF, PBF@N, and PBF@N@S
Sample | BET surface area (m2/g) |
PBF | 35 |
PBF@N | 1342 |
PBF@N@S | 1696 |
The morphologies of PBF, PBF@N, and PBF@N@S were studied by SEM and TEM. As can be seen from Figure 4A, the PBF remains the structure of fibers, which shows a smooth surface and no porous structure. PBF@N (Figure 4B) and PBF@N@S (Figure 4C-D) exhibit large amounts of pore structures, which originates from the activation of KOH. According to Figure 4C-D, the large pores connect with each other with a pore size of 1-2 μm in the PBF@N@S. In addition, it can be seen from Figure 4D that plenty of
Figure 4. SEM images of PBF (A), PBF@N (B), and PBF@N@S (C-D) and TEM images of the PBF@N@S (E-F). PBF: Bare biochar fibers; PBF@N: N-doped porous biochar fibers; PBF@N@S: N, S dual-doped porous biochar fibers; SEM: scanning electronic microscopy; TEM: transmission electron microscopy.
TEM also exhibits the formation of an abundant porous structure in the sample PBF@N@S. As shown in Figure 4E and Figure 4F, the abundant microporous structure has small pore sizes, of which most of them are smaller than 2 nm. The abundant microporous structure is beneficial to restrict the volume change of the S due to the strong confinement effect and contributes to the physical adsorption slowing down the S loss. Meanwhile, the crystal lattice of PBF@N@S is hardly observed from the high-resolution TEM image [Figure 4F], indicating an irregular carbon, which has a positive effect on bundling polysulfide.
XPS is used to study the chemical characteristics of the surface of the PBF@N@S. Figure 5A shows the
Figure 5. XPS spectra of PBF@N@S: full survey (A), C 1s spectra (B), N 1s spectra (C), and S 2p spectra (D). PBF@N@S: N, S dual-doped porous biochar fibers; XPS: X-ray photoelectron spectroscopy.
Elemental composition of PBF@N@S obtained from XPS
Elements | C | N | S | O |
Percentage (at.%) | 81.44 | 3.18 | 1.34 | 14.04 |
Electrochemical measurements
To investigate the electrochemical properties of the cathode, electrochemical cycling was first implemented. Figure 6A illustrates the cycling performances of PBF-S-50%, PBF@N-S-50%, and PBF@N@S-S-50% at a current rate of 0.1C. All these three electrodes showed high discharge capacities even after 200 cycles. Obviously, it demonstrates the cycling performance of PBF@N@S-S-50% electrodes is much better than PBF@N-S-50% and PBF-S-50%. The introduction of N and S atoms into the PBF induces asymmetric sites for binding lithium polysulfide, which enables the PBF@N@S electrode to have higher capacity and battery cycling stability. On the other hand, the hierarchical porous structure of PBF@N@S ensures good electrical contact between S and the conductive carbon framework and further accelerates Li-ions transportation, which improves the high performance of the battery.
Figure 6. Electrochemical performances of as-prepared electrode for Li-S batteries: (A) Cycling performance of the PBF-S-50%,
Furthermore, an excellent rate capability performance is observed in Figure 6B. With the C rate successively increasing from 0.1 to 0.2, 0.5, 1, and 2C, the capacity of the three electrodes gradually decreases. When the C rate switches back to 0.1C after 50 cycles, the capacity of the electrodes can be recovered to the initial stage, indicative of their good rate capability. Notably, it is evident that PBF@N@S-S-50% exhibits consistently better electrochemical performance than both PBF@N-S-50% and PBF-S-50% cathodes, including larger specific capacity, longer cyclic life, and superior rate capability.
The electrochemical mechanism of a Li-S battery was studied by cyclic voltammetry (CV), as shown in Figure 6C. The typical CV curves of a S cathode contain two reductive peaks and one oxidative peak. During the cathodic scan, peaks at around 2.35 V were attributed to the transformation from solid sulfur to soluble polysulfide intermediates (Sn2-, n ≥ 4)[36,37]. The other reduction peak that appeared at around 2.02 V can be agreed with the reaction of polysulfide to the lower order insoluble Li2S2 and finally to Li2S from small sulfur molecule S42-[38,39]. Subsequently, the anodic peak at around 2.35 V was coherent with the conversion of Li2S2/Li2S to polysulfides and sulfur. Meanwhile, the peak position and area remained nearly good reaction reversibility in the successive cycles, indicating good stability.
Typical voltage capacity profiles of the PBF@N@S-S-50% at different cycles are shown in Figure 6D. The discharge curves show two typical plateaus, which locate at around 2.1 V and 2.3 V, respectively; this could be assigned to a two-step reaction of S with Li during the discharge process, agreeing well with the results of CV measurements. In addition, the plateau at about 2.1 V could be commonly observed, which might originate from the strong adsorption process of Li2S2 on the microporous besides the reduction process (from Li2S2 to Li2S). This observation is similar to other carbon-sulfur nanocomposite electrodes[40,41]. Such strong interactions can guarantee the stable performance of PBF@N@S-S-50% electrodes. Moreover, the discharge plateaus are considerably stable even after ten cycles, which ensures excellent cyclic performance.
Electrochemical impedance spectroscopy (EIS) is a powerful method for analyzing cyclic changes and was measured to get further insight into the electrochemical performance. The semicircle at medium-to-high frequency was attributed to the charge-transfer process at the interface between the electrolyte and electrode[42]. The Warburg impedance that occurred in low frequency was associated with the semi-infinite diffusion of soluble lithium polysulfide in the electrolyte[43,44]. Figure 7A shows the Nyquist plots of fresh PBF@N@S-S-50%, PBF@N-S-50%, and PBF-S-50% cathodes. The introduction of N and S atoms makes the impedance of PBF@N@S and PBF@N much larger than PBF. Figure 7B exhibits the EIS plots of
CONCLUSIONS
A N, S co-doped hierarchical porous carbon has been successfully prepared via a simple one-step activation method and was employed as a cathode in Li-S batteries. The obtained porous carbon shows a highly interconnected network, which can encapsulate sulfur and polysulfide. The co-doped N and S into the carbon matrix could generate more active sites, which improves the battery capacity and cycling stability. The electrode illustrated an improved electrochemical performance and delivered a high initial capacity (above 1,200 mAh/g at 0.1C) and high reversible capacity after 200 cycles. This work provides a facile route to synthesize heteroatom-doped porous carbon materials for Li-S battery applications.
DECLARATIONS
Authors’ contributionsConceptualization, methodology, investigation, writing, review & editing: Li X
Conceptualization, writing, review & editing: Sun K
Availability of data and materialsNot applicable.
Financial support and sponsorshipNone.
Conflicts of interestAll authors declared that there are no conflicts of interest.
Ethical approval and consent to participateNot applicable.
Consent for publicationNot applicable.
Copyright© The Author(s) 2023.
REFERENCES
1. Qin J, Yang Z, Xing F, Zhang L, Zhang H, Wu Z. Two-dimensional mesoporous materials for energy storage and conversion: current status, chemical synthesis and challenging perspectives. Electrochem Energy Rev 2023;6:9.
2. Li F, Liu R, Liu J, Li H. Voltage hysteresis in transition metal oxide cathodes for Li/Na-Ion batteries. Adv Funct Materials 2023;33:2300602.
3. Wu X, He G, Ding Y. Dealloyed nanoporous materials for rechargeable lithium batteries. Electrochem Energ Rev 2020;3:541-80.
5. Li H, Zhang W, Sun K, et al. Manganese-based materials for rechargeable batteries beyond lithium-ion. Adv Energy Mater 2021;11:2100867.
6. Xin S, Chang Z, Zhang X, Guo Y. Progress of rechargeable lithium metal batteries based on conversion reactions. Natl Sci Rev 2017;4:54-70.
7. Kim J, Lee D, Jung H, Sun Y, Hassoun J, Scrosati B. An advanced lithium-sulfur battery. Adv Funct Mater 2013;23:1076-80.
9. Tao T, Lu S, Fan Y, Lei W, Huang S, Chen Y. Anode improvement in rechargeable lithium-sulfur batteries. Adv Mater 2017;29:1700542.
10. Liu B, Fang R, Xie D, et al. Revisiting scientific issues for industrial applications of lithium-sulfur batteries. Energy Environ Mater 2018;1:196-208.
11. Zhao X, Cheruvally G, Kim C, et al. Lithium/sulfur secondary batteries: a review. J Electrochem Sci Technol 2016;7:97-114.
12. Wang M, Bai Z, Yang T, et al. Advances in high sulfur loading cathodes for practical lithium-sulfur batteries. Adv Energy Mater 2022;12:2201585.
13. Manthiram A, Fu Y, Su YS. Challenges and prospects of lithium-sulfur batteries. Acc Chem Res 2013;46:1125-34.
14. Huang L, Li J, Liu B, et al. Electrode design for lithium-sulfur batteries: problems and solutions. Adv Funct Mater 2020;30:1910375.
15. Zhang Q, Cheng X, Huang J, Peng H, Wei F. Review of carbon materials for advanced lithium-sulfur batteries. Carbon 2015;81:850.
16. Xu Z, Kim J, Kang K. Carbon nanomaterials for advanced lithium sulfur batteries. Nano Today 2018;19:84-107.
17. Liu H, Jia G, Zhu S, Sheng J, Zhang Z, Li Y. Functionalized carbon-based composite materials for cathode application of lithium-sulfur batteries. Acta Chimica Sinica 2022;80:89-97.
18. Zhang SS. Heteroatom-doped carbons: synthesis, chemistry and application in lithium/sulphur batteries. Inorg Chem Front 2015;2:1059-69.
19. Yang J, Xie J, Zhou X, et al. Functionalized N-Doped porous carbon nanofiber webs for a lithium-sulfur battery with high capacity and rate performance. J Phys Chem C 2014;118:1800-7.
20. Niu S, Lv W, Zhou G, et al. N and S co-doped porous carbon spheres prepared using L-cysteine as a dual functional agent for high-performance lithium-sulfur batteries. Chem Commun 2015;51:17720-3.
21. Kim E, Lee AS, Lee T, et al. Organic dye-derived N, S co-doped porous carbon hosts for effective lithium polysulfide confinement in lithium-sulfur batteries. Nanomaterials 2021;11:2954.
22. Díez N, Sevilla M, Fuertes AB. N/S-co-doped porous carbon nanoparticles serving the dual function of sulfur host and separator coating in lithium-sulfur batteries. ACS Appl Energy Mater 2020;3:3397-407.
23. Sun K, Wang C, Tebyetekerwa M, Zhao XS. Electrocapacitive desalination with nitrogen-doped hierarchically structured carbon prepared using a sustainable salt-template method. Chem Eng J 2022;446:137211.
24. Kim DK, Seul Byun J, Moon S, Choi J, Ha Chang J, Suk J. Molten salts approach of metal-organic framework-derived nitrogen-doped porous carbon as sulfur host for lithium-sulfur batteries. Chem Eng J 2022;441:135945.
25. Yang H, Yang Y, Zhang X, et al. Nitrogen-doped porous carbon networks with ACtive Fe-N
26. Benítez A, Amaro-gahete J, Chien Y, Caballero Á, Morales J, Brandell D. Recent advances in lithium-sulfur batteries using biomass-derived carbons as sulfur host. Renew Sust Energ Rev 2022;154:111783.
27. Liu P, Wang Y, Liu J. Biomass-derived porous carbon materials for advanced lithium sulfur batteries. J Energy Chem 2019;34:171-85.
28. Li G, Sun J, Hou W, Jiang S, Huang Y, Geng J. Three-dimensional porous carbon composites containing high sulfur nanoparticle content for high-performance lithium-sulfur batteries. Nat Commun 2016;7:10601.
29. Pang Q, Tang J, Huang H, et al. A nitrogen and sulfur dual-doped carbon derived from polyrhodanine@cellulose for advanced lithium-sulfur batteries. Adv Mater 2015;27:6021-8.
30. Yu X, Yin Y, Ma C, et al. N-doped porous carbon@CNT nanowire as effective polysulfides adsorption-catalysis interlayer for high-performance lithium-sulfur batteries. Chem Eng Sci 2023;268:118400.
31. Tomer VK, Malik R, Tjong J, Sain M. State and future implementation perspectives of porous carbon-based hybridized matrices for lithium sulfur battery. Coord Chem Rev 2023;481:215055.
32. Peng W, Han G, Huang Y, Cao Y, Song S. Insight the effect of crystallinity of natural graphite on the electrochemical performance of reduced graphene oxide. Results Phys 2018;11:131-7.
33. Schroer ZS, Wu Y, Xing Y, et al. Nitrogen-sulfur-doped graphene quantum dots with metal ion-resistance for bioimaging. ACS Appl Nano Mater 2019;2:6858-65.
34. Yang Z, Dai Y, Wang S, Cheng H, Yu J. In situ incorporation of a S, N doped carbon/sulfur composite for lithium sulfur batteries. RSC Adv 2015;5:78017-25.
35. Chen M, Zhao S, Jiang S, et al. Suppressing the polysulfide shuttle effect by heteroatom-doping for high-performance lithium-sulfur batteries. ACS Sustainable Chem Eng 2018;6:7545-57.
36. Bao W, Su D, Zhang W, Guo X, Wang G. 3D metal carbide@mesoporous carbon hybrid architecture as a new polysulfide reservoir for lithium-sulfur batteries. Adv Funct Mater 2016;26:8746-56.
37. Wang D, Liu J, Bao X, Qing C, Zhu T, Wang H. Macro/mesoporous Carbon/defective TiO2 composite as a functional host for lithium-sulfur batteries. ACS Appl Energy Mater 2022;5:2573-9.
38. Lei D, Li J, Xiang M, et al. Synergistic constraint and conversion of lithium polysulfide using a 3D hollow carbon interlayer in ultrahigh sulfur content Li-S batteries. Appl Surf Sci 2023;623:157080.
39. Zhang H, An Y, Li S, et al. Honeycomb porous N-doped carbon synergized with ultrafine Ni2P electrocatalyst for boosting polysulfide conversion in lithium-sulfur batteries. Electrochimica Acta 2023;445:142047.
40. Wen Y, Wang X, Huang J, Li Y, Li T, Ren B. Coffee grounds derived sulfur and nitrogen dual-doped porous carbon for the cathode material of lithium-sulfur batteries. Carbon Lett 2023;33:1265-78.
41. Li M, Liu Z, Tan L, et al. Fabrication of cubic and porous carbon cages with in-situ-grown carbon nanotube networks and cobalt phosphide for high-capacity and stable lithium-sulfur batteries. ACS Sustainable Chem Eng 2022;10:10223-33.
42. Ma L, Liu Q, Zhu H, Liu L, Kang C, Ji Z. Flower-like Ni3Sn2@Ni3S2 with core-shell nanostructure as electrode material for supercapacitors with high rate and capacitance. J Colloid Interface Sci 2022;626:951-62.
43. Peng W, Wang W, Han G, Huang Y, Zhang Y. Fabrication of 3D flower-like MoS2/graphene composite as high-performance electrode for capacitive deionization. Desalination 2020;473:114191.
44. Sun M, Wang X, Li Y, Zhao Z, Qiu J. Integration of desulfurization and lithium-sulfur batteries enabled by amino‐functionalized porous carbon nanofibers. Energy & Environ Materials 2023;6:e12349.
Cite This Article

How to Cite
Download Citation
Export Citation File:
Type of Import
Tips on Downloading Citation
Citation Manager File Format
Type of Import
Direct Import: When the Direct Import option is selected (the default state), a dialogue box will give you the option to Save or Open the downloaded citation data. Choosing Open will either launch your citation manager or give you a choice of applications with which to use the metadata. The Save option saves the file locally for later use.
Indirect Import: When the Indirect Import option is selected, the metadata is displayed and may be copied and pasted as needed.
About This Article
Copyright
Data & Comments
Data
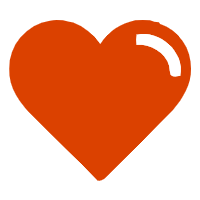
Comments
Comments must be written in English. Spam, offensive content, impersonation, and private information will not be permitted. If any comment is reported and identified as inappropriate content by OAE staff, the comment will be removed without notice. If you have any queries or need any help, please contact us at [email protected].