Risk factors influencing chronic inflammation in neoplastic transition to prostate cancer
Abstract
Chronic inflammation and its role in driving cellular plasticity have recently been documented as a significant risk factor for prostate cancer. The progression of prostate cancer has been linked to stages of inflammation-driven changes, ranging from simple atrophy to prostatic intraepithelial neoplasia and eventually to low- and high-grade neoplastic forms. Long-term oxidative stress and the genetic damage caused by chronic inflammation are among the well-characterized risk factors in the development of prostate cancer. Both uncontrollable and controllable factors contribute to this transition process. Non-modifiable risk factors for prostate cancer include age, race, ethnicity, family history of obesity, and certain genetic predispositions. Modifiable risk factors, such as a sedentary lifestyle, poor diet, obesogenic habits, and microbial dysbiosis, may further elevate the risk of neoplastic transformation. Additionally, environmental pollutants, like chlordecone and nitrates, can interact with biological factors, potentially influencing cellular plasticity. These factors collectively contribute to an increased risk of prostate cancer and may facilitate neoplastic progression. Certain molecular markers have also been implicated in promoting chronic inflammation, enhancing cellular proliferation, and inhibiting apoptosis, thereby aiding in this transition. This review provides a comprehensive summary of the known modifiable and non-modifiable risk factors that contribute to the neoplastic transition in the prostate and elevate the risk of prostate cancer.
Keywords
INTRODUCTION
Prostate cancer remains the second leading cause of cancer-related death among men. In 2024, approximately 300,000 new cases are expected to be diagnosed in the United States, with an estimated 35,250 deaths anticipated from the disease[1]. The recent rise in prostate cancer mortality is largely attributed to an increase in high-grade tumor detection, following the reduced recommendation for serum PSA testing[1]. Major non-modifiable risk factors for prostate cancer include age, race, family history, and genetic predisposition[2]. African-American men are disproportionately affected, facing nearly double mortality risk compared to other racial groups[3]. This disparity is thought to stem from a combination of risk factors including race, obesity, socioeconomic factors, environmental exposures, and genetic variations. Additionally, populations adopting a Westernized diet and sedentary lifestyle face a heightened risk of developing the disease. Environmental exposures, such as pollutants and contaminated water in lower-income areas, further elevate risk[4]. Both lifestyle and environmental factors can contribute to increased prostate cancer risk and are recognized as modifiable risk factors. Familial obesity and related heritable traits have also been linked to a higher risk of the disease. Socioeconomic challenges, including limited access to healthcare and healthy foods, exacerbate obesity and subsequently prostate cancer risk. Furthermore, low socioeconomic status increases exposure to environmental pollutants that may promote chronic intraprostatic inflammation and neoplastic development. A list of modifiable and non-modifiable risk factors is summarized in Figure 1.
Figure 1. Modifiable and non-modifiable risk factors in prostate cancer. Non-modifiable risk factors are intrinsic to individuals’ genetics, biology, or natural environment that cannot change. Modifiable risk factors are lifestyle or environmental factors that can potentially be changed to reduce the risk of developing prostate cancer. While modifiable risk factors have less direct evidence than non-modifiable ones, there is still some association between these factors and prostate cancer risk.
Increasing scientific evidence indicates that both modifiable and non-modifiable risk factors contribute to elevated oxidative stress in the prostate gland. This stress results from an imbalance between the overproduction of reactive oxygen species (ROS) and the body’s diminished ability to neutralize these reactive intermediates. This imbalance can cause tissue damage by altering DNA, proteins, and other macromolecules, leading to a cascade of inflammatory responses[5]. The link between inflammation and cancer is not a new area of study. In 1863, Virchow proposed that cancer often originates in areas of chronic inflammation[6]. Today, chronic inflammation is recognized as a contributing factor to several types of cancer, including liver, colon, lung, bladder, stomach, and pancreatic cancers[7,8]. Multiple studies, including research from our group, have demonstrated an association between chronic inflammation and prostate cancer[9-11].
ROLE OF INFLAMMATION IN PROSTATE CANCER DEVELOPMENT
Chronic intraprostatic inflammation, also known as prostatitis, is a painful condition characterized by an inflamed, swollen, and tender prostate. Despite the use of antibiotics, it can be challenging to treat. Inflammation in the prostate can be classified as acute or chronic and may be triggered by bacterial or non-bacterial agents. Acute inflammation is typically caused by bacterial, viral, fungal, or parasitic infections, which result in the recruitment and activation of polymorphonuclear leukocytes[12]. However, chronic low-grade inflammation may arise from non-bacterial factors, such as an imbalanced diet, exposure to environmental pollutants, hormonal changes, or genetic predispositions[13-15]. The microbiome has been shown to influence prostate inflammation in various prostatic diseases, including prostatitis, benign prostatic hyperplasia, and prostate cancer[16]. Studies have revealed a positive correlation between a clinical history of prostatitis and an increased risk of prostate cancer[10]. A retrospective needle biopsy study further demonstrated that chronic inflammation contributes to neoplastic development[13]. Additionally, other research has observed an increase in polymorphonuclear leukocyte aggregation and elevated PSA levels due to prostate inflammation. Interestingly, the use of non-steroidal anti-inflammatory drugs (NSAIDs) has been linked to reduced serum PSA levels and decreased immune infiltration in prostate biopsies, indicating a potential reduction in prostate cancer risk[17]. Therefore, it is reasonable to propose that unresolved chronic prostate inflammation could eventually lead to neoplastic transformation and, subsequently, the development of prostate cancer[14].
Chronic inflammation and neoplastic development in the prostate
In prostate carcinogenesis, there is growing evidence that regenerative "risk factor lesions", known as proliferative inflammatory atrophy (PIA), emerge as a result of various insults that can damage the prostate[18]. PIA is an atrophic lesion with a morphology resembling simple atrophy but is proliferative in nature. It is associated with inflammation and is considered a possible precursor to prostatic intraepithelial neoplasia (PIN) and prostate cancer. PIA lesions appear to result from the proliferation of prostate epithelial cells in response to inflammatory insults. These lesions may represent a precursor to prostate cancer by providing a productive environment for genomic aberrations and uncontrolled cellular growth. This cellular proliferation can lead to numerous somatic mutations, gene deletions, amplifications, chromosomal rearrangements, changes in DNA methylation, and molecular stress signals - all of which create a favorable environment for tumor development[19]. Focal prostatic epithelial atrophy has long been recognized as a common finding in the peripheral zone of the prostate in aging men[18,20,21]. Many of these atrophic regions are linked to either acute or chronic inflammation and are characterized by proliferative epithelial cells. These lesions are mainly located in the peripheral zone of the prostate, which is the area where prostate cancer typically occurs. Moreover, these regions are often adjacent to high-grade prostatic intraepithelial neoplasia (PIN) lesions or small-volume cancers, particularly in older men. Accumulating evidence supports the potential role of PIA as a precursor lesion to prostate cancer. Studies in rodent models have shown that focal areas of epithelial atrophy, accompanied by inflammation, may play a role in cancer development[22]. Furthermore, genetic changes, such as gains in the centromeric region of chromosome 8, have been observed in human PIA, PIN, and prostate cancer[23].
Mutations in the p53 gene, a common feature in many cancers, have been detected in approximately 5% of post-atrophic hyperplasia lesions, a variant of PIA. This mutation rate is similar to that observed in high-grade PIN[23]. A study by Palapattu et al. proposed a model in which PIA arises as a response to microenvironmental stressors such as local ischemia, infections, and toxin exposure affecting normal prostate epithelial cells[24]. According to this model, regions of PIA experiencing oxidative genome damage and unable to adapt may progress to PIN or prostate cancer. Additionally, PIA lesions have been found to share some genetic features with prostate cancer[23]. PIN refers to the neoplastic growth of epithelial cells within preexisting prostatic acini or ducts, with high-grade PIN (HGPIN) being recognized as a precursor to prostate cancer[23,25]. In the model described earlier, it is hypothesized that HGPIN may arise after a period of atrophy, during which atrophic lesions are linked to inflammation and subsequent development of HGPIN, eventually transitioning into cancer. It has also been suggested that PIA might progress directly to carcinoma without passing through a HGPIN phase[24]. Some cancers may originate from PIN lesions without accompanying atrophy, while others can develop without any precursor lesions at all. Lastly, it has been suggested that low-grade carcinomas in the transition zone may arise from adenosis or atypical adenomatous hyperplasia[23].
In 2006, a study conducted by our group examined 177 prostate needle biopsies to assess chronic inflammation and other pathological findings mentioned earlier[13]. Of the 177 biopsies, 144 showed evidence of chronic inflammation. Follow-up biopsies conducted five years later revealed occurrences of prostate cancer with Gleason scores ranging from 6 to 9, or the development of high-grade prostatic intraepithelial neoplasia (HGPIN). These findings indicate a strong correlation between chronic intraprostatic inflammation and both premalignant and malignant changes in the prostate epithelium[13]. In another study by Glover et al., the potential link between chronic inflammation and neoplastic progression was evaluated through an analysis of 36 prostate needle biopsies[9]. A subsequent follow-up study reassessed the presence, absence, and extent of chronic inflammation, along with other relevant pathological findings, in prostate needle biopsies obtained from patients with clinical signs of suspected malignancy[26]. Over three years, a review was conducted on 1,006 prostate needle biopsy cases, which included 202 cases featuring chronic inflammation and 42 cases without inflammation, drawn from an initial pool of 244 patients who did not have prostate cancer. Among the cases with chronic inflammation, 21 were found to have HGPIN, compared to only one case without inflammation. Additionally, pathological findings such as post-atrophic hyperplasia and proliferative inflammatory atrophy were exclusively observed in patients with a history of chronic inflammation. Out of the 202 cases with prior chronic inflammation, 70 patients were diagnosed with prostate adenocarcinoma. In contrast, initial biopsies with benign or no inflammation largely lacked high-grade prostate cancer. Biopsies showing encroaching inflammation were associated with higher-grade prostate cancers, with Gleason scores of 2 or above[26].
In another study from our group, prostate biopsies were categorized into groups based on simple atrophy, proliferative inflammatory atrophy (PIA), high-grade prostatic intraepithelial neoplasia (HGPIN), and cancer, with protein expression levels of Bcl2 and proliferative cell nuclear antigen (PCNA) being evaluated[9]. It was hypothesized that, under chronic inflammation, proliferating epithelial cells attempt to adapt to the altered microenvironment by promoting survival mechanisms. This was reflected by an initial increase in the expression of the anti-apoptosis protein Bcl2 within the biopsy specimens[9]. However, prolonged exposure to oxidative stress may lead to genomic damage in a subset of cells, impairing their normal regulation of cell death. These cells survive and continue to proliferate, favoring the development of malignancy[9]. This transition is characterized by a decrease in Bcl2 expression and a concurrent increase in PCNA expression.
Chronic inflammation also induces progressive changes in acinar or ductal epithelium, which may result in the development of HGPIN[13]. Putzi et al. observed frequent morphologic transitions from PIN and focal atrophy to carcinoma lesions in the surrounding tissue, further implicating inflammation in prostate cancer development[14]. In radical prostatectomies, HGPIN was found to merge with PIA, along with morphological transitions from atrophy to PIN. Carcinoma lesions were also seen adjacent to these PIA lesions, suggesting that such lesions may transition to carcinoma[14]. Kryvenko et al., through a nested case-control study, reported that the presence of HGPIN and simple atrophy increased the risk of prostate cancer[15]. In this cohort, HGPIN was associated with a two-fold increase in prostate cancer risk, with 65% of patients with HGPIN in their initial biopsies later diagnosed with prostate cancer[15]. The California Men’s Health Study (CMHS), a large-scale, multiethnic longitudinal study, aimed to understand prostate cancer development among American males[27]. The CMHS found an increased risk of prostate cancer in patients with a history of prostatitis or chronic inflammation[11]. Similar studies, such as The Prostate, Lung, Colorectal, and Ovarian (PLCO) Trial and the European Randomized Screening for Prostate Cancer (ERSPC), also showed a correlation between elevated PSA levels due to inflammation and the development of prostate cancer.
RISK FACTORS INFLUENCING PROSTATE CANCER
Several non-modifiable and modifiable risk factors for prostate cancer have been previously examined. The influence of these risk factors on the interactions among various biological components may play a role in prostate tumorigenesis.
Non-modifiable risk factors
Disparities in prostate cancer incidence and mortality are well-documented, particularly between African-American (AA) and Caucasian-American (CA) populations[3]. While socioeconomic factors and environmental exposures are significant contributors, biological factors also play a crucial role in this disparity. For instance, androgen receptors and their ligands, such as testosterone, are known to facilitate prostate cancer growth[28]. African-American men have been observed to have higher levels of free testosterone and androgen receptor proteins, which may increase their risk of developing or progressing prostate cancer. Additionally, somatic and germline hypermutations have been noted in AA men with prostate cancer. Notably, AA men have a 2.8-fold higher frequency of mutations in the oncogenic gene BRCA2 compared to CA men[28]. Disease-related loci in AA men with prostate cancer also show higher levels of DNA hypermethylation[28]. FOXA1, a transcription factor essential for androgen receptor binding and proper prostate gland development, has been found to be more frequently mutated in AA men compared to CA men[29]. However, the specific implications of these mutations remain unclear[28-30]. These biological factors, including mutations and ligand signaling associated with race, may contribute to a higher risk of neoplastic growth and prostate cancer progression.
Prostate cancer risk is strongly associated with age, with the highest incidence occurring in men aged 65 years and older[31]. The average age at diagnosis is 66, indicating that the development of prostate cancer can span several decades. Incidence rates of prostate cancer rise significantly with age, from 9.2 per 100,000 men aged 40-44 to 984.9 per 100,000 men aged 70-74[32]. Over time, atrophic and simple lesions in the prostate may evolve and undergo neoplastic transitions, leading to cancer development. Autopsy studies have indicated that localized and minor prostatic lesions can remain undetected for many years before advancing to carcinogenesis[33].
Familial obesity and its potential link to prostate cancer susceptibility remain underexplored. Although hereditary obesity is known to elevate the risk of developing prostate cancer, the specific association between inherited obesity and prostate cancer susceptibility has not been extensively studied. The increased risk associated with obesity may contribute to prostate cancer development, potentially interacting with prostate cancer gene expression, particularly at younger ages. Further research is required to investigate how obesity influences the expression of prostate cancer susceptibility genes and to determine whether these heritable conditions can co-develop. Understanding this interplay could provide insights into how genetic predispositions to obesity may impact prostate cancer risk.
Familial cancers are characterized by specific genetic mutations and defined inheritance patterns. The concept of familial aggregation refers to the increased incidence of prostate cancer within families, which can be due to shared environmental factors, genetic predispositions, a combination of both, or chance, given the high overall incidence of the disease. Familial prostate cancer accounts for approximately
Prostate cancer susceptibility genes, such as MIC1, RNASEL, and MSR1, have been linked to familial prostate cancer[36]. These genes typically encode proteins that play critical roles in the body’s response to infection, inflammation, and oxidative stress [Table 1]. Mutations in these proteins can impair their ability to prevent carcinogenesis through these pathways[36,37]. A significant gene linked to prostate carcinogenesis is BRCA1/2. While germline mutations in the breast cancer susceptibility genes BRCA1 and BRCA2 are widely recognized for their involvement in breast and ovarian cancers, they also elevate the risk of other cancers, including prostate cancer[38]. Research has demonstrated that germline mutations in BRCA1/2 genes are linked to familial prostate cancer aggregation[39]. Male carriers of BRCA1/2 mutations have been shown to have a higher risk of prostate cancer compared to those without these mutations[40].
Prostate cancer susceptibility genes
The protein p63, which is homologous to the tumor suppressor gene p53, is involved in regulating the growth and development of various epithelial tissues, including those in the prostate[41,42]. Notably, p63 is selectively expressed by basal cells in the normal prostate gland, which possess stem cell properties and differentiate into secretory luminal cells[41,42]. Studies have shown that p63 is persistently expressed in basal cells of atrophic and benign prostate lesions but is absent in prostate cancer[43]. Abnormalities on chromosome 8 have been associated with lesions like proliferative inflammatory atrophy (PIA) and post-atrophic hyperplasia (PAH), as well as with prostate cancer and high-grade prostatic intraepithelial neoplasia (PIN)[9,44]. Alpha-methylacyl-coenzyme A racemase (AMACR) is a commonly used marker for detecting neoplastic development in the prostate[40]. While AMACR is generally a positive marker for prostate neoplasms, it has also been observed in some benign glands and may be negative in certain cases of prostatic adenocarcinoma[45]. This results in a negative correlation when using p63 and AMACR together, as p63 marks basal cells and AMACR indicates neoplastic progression[45]. Man and Gardner[46] investigated focal basal cell layer disruptions (FBCLDs) in pre-invasive and invasive prostate tumors using various basal cell biomarkers, including p63. They found that FBCLDs were associated with an increase in the proliferative marker Ki-67 and AMACR in luminal cells overlying the basal cells. Additionally, glands adjacent to those with FBCLDs were often negative for AMACR. This study suggested that p63 expression may be lost early in the progression of prostate cancer as a result of internal factors such as radiation, carcinogens, or inflammation, which induce permanent DNA damage in primitive stem cells and contribute to the development of FBCLDs[46].
Inducible nitric oxide synthase (iNOS) is recognized as a significant contributor to nitric oxide (NO) production during inflammatory reactions in tumor tissues and cell lines[47]. However, the role of iNOS in cancer is debated, with some studies showing lower iNOS expression in tumor tissues compared to normal tissues, while others report increased iNOS expression in various forms of human cancer[48-50]. Glover et al. assessed the expression and staining intensity of several biomarkers, including p63, AMACR, COX-2, iNOS, Bcl2, and GSTP1, using a scale from 0 (no staining) to 3 (high intensity)[9]. Their findings indicated that areas preserving the basal cell layer with p63 expression at 72% also exhibited iNOS expression at 68%, with a moderately high staining intensity. Conversely, glands showing more neoplastic characteristics had reduced p63 expression and increased AMACR levels. Additionally, an increase in AMACR expression was associated with a decrease in the staining intensity of iNOS in the transitional glands[9].
The proinflammatory enzyme cyclooxygenase-2 (COX-2) plays a crucial role in converting arachidonic acid to prostaglandins, which are involved in regulating cell proliferation and inflammation. Evidence indicates that COX-2 is upregulated in various cancers, including prostate cancer[36]. Karaivanov et al. showed that COX-2 can be induced by mitogens, tumor promoters, cytokines, growth factors, and hormones, leading to the activation of IL-1, tumor necrosis factor-alpha, NF-κB, and other pathways[47]. Prostaglandins, produced from arachidonic acid via COX-2, are involved in modulating cell proliferation. In the context of prostate cancer, inflammation and reactive oxygen species (ROS) production can alter the balance of prostaglandins[24,36]. Typically, ROS are managed by the superoxide dismutase enzyme system, but oxidative stress can lead to increased production of arachidonic acid, which is then converted to prostaglandins by COX-2. It has been suggested that COX-2 expression may increase in prostatic intraepithelial neoplasia (PIN) but not in prostate cancer itself. Additionally, COX-2 expression has been found to decrease in proliferative inflammatory atrophy (PIA) lesions that are negative for p63 expression[47,51].
Glutathione S-transferase pi class (GSTP1) is a gene that encodes an antioxidant enzyme crucial for detoxifying carcinogens and inflammatory oxidants in prostate cells[36]. Increased GSTP1 expression is often a marker of cellular stress and is commonly observed in proliferative inflammatory atrophy (PIA) and cases of chronic prostatic inflammation[52]. Nelson et al. proposed that PIA cells upregulate GSTP1 in response to oxidative stress and inflammatory oxidants, using it to protect against genomic damage[53]. However, in prostate cancer, GSTP1 and other tumor suppressor genes often undergo epigenetic modifications, such as DNA methylation and histone changes, leading to their loss[54]. GSTP1 CpG island hypermethylation has been notably observed in PIA lesions, often adjacent to high-grade prostatic intraepithelial neoplasia (HGPIN) or early cancer[54]. As chronic inflammation persists, it can lead to the emergence of PIA cells with defective GSTP1 genes, impairing their ability to counteract oxidant damage. This genetic dysfunction is believed to contribute to genomic instability, promoting the progression from PIA to PIN and eventually to prostate cancer, with GSTP1 defects potentially facilitating malignant transformation[47,53].
Modifiable risk factors
A sedentary lifestyle, characterized by poor diet, insufficient exercise, and related conditions such as obesity, is known to impact prostate cancer risk and progression[55]. Prolonged periods of inactivity have been linked to various chronic diseases, including prostate cancer. Orsini et al. documented that men with sedentary work habits faced a 27% increased risk of prostate cancer development[56]. While some studies show conflicting results, the association between sedentary behavior and obesity, which in turn elevates prostate cancer risk, is well-established. Engaging in regular physical activity has been shown to reduce prostate cancer risk by 10% to 30%[57]. Exercise can lower systemic inflammation, hyperinsulinemia, insulin-like growth factor 1 (IGF-1) levels, androgens, and proinflammatory molecules[58]. Additionally, physical activity is associated with improved immune function and a healthier gut microbiome[58].
Dietary choices significantly impact prostate cancer risk and progression. Numerous studies have linked high-fat diets, especially those dense in animal fats and red meat, to an increased risk of prostate cancer[59]. Men who consume significant amounts of red meat and dairy products are at a higher risk of developing prostate cancer and frequently present with more aggressive forms of the disease[59]. The consumption of cooked red meat can lead to increased levels of 2-Amino-1-methyl-6-phenylimidazo[4,5-b]pyridine (PhIP), a potent heterocyclic amine (HCA) and carcinogen[60]. Similarly, high dairy intake can lead to elevated levels of phytanic acid, which, when metabolized, produces hydrogen peroxide - a reactive oxygen species that can cause oxidative damage to prostatic epithelial cells[59]. Diet also influences the gut microbiome, potentially causing microbial imbalances that might affect the prostate. Diets high in fiber can enhance the production of short-chain fatty acids in the gut microbiome, with varying effects on prostate health. Matsushita et al. demonstrated that high-fat diets and obesity alter insulin-like growth factor 1 (IGF-1) expression. In prostate cancer mice models, high-fat diets combined with antibiotic treatment affected IGF-1 levels and oncogenic pathways such as MAPK and PI3K[61]. Obesity is linked with higher IGF-1 expression due to hyperinsulinemia, which can further contribute to prostate cancer development[61]. Overall, a poor diet, particularly one high in animal fats and red meat, combined with the obesity resulting from such dietary habits, can facilitate the neoplastic transition from benign prostatic atrophy to carcinoma. This effect is magnified when prostatic inflammation is present, highlighting the role of diet and lifestyle in prostate cancer risk and progression.
Obesity, particularly the overgrowth of white adipose tissue, is strongly linked to chronic inflammation, fibrosis, and dysfunction in adipose tissues, all of which contribute to cancer promotion and progression. This association is evident in the peri-prostatic white adipose tissue, which surrounds the prostate and affects its environment. Expansion of peri-prostatic white adipose tissue surrounding the prostate surface is associated with increased expression of NPYIR, LEP, ANGPTI, and HSPB8 genes[62]. Expression of these genes is associated with the regulation of lipolysis, apoptosis, and cellular proliferation. The expression of FADS1, a gene crucial for regulating immune inflammation, is reduced in obese individuals[62]. The rapid expansion of adipose tissues disrupts the homeostasis of the immune system, leading to polarization and proliferation of immune cells. Macrophages increase in proportion to adiposity and leukocytes are further proliferative and polarized in white adipose tissue overgrowth. Increased levels of immune cells in this region increase cytokines and other inflammatory molecules, leading to prostatic inflammation. Adipokines such as leptin and growth factors including IGF1 and TGFβ secreted by adipose tissues from the peri-prostatic white adipose tissue can further regulate prostate cancer aggressiveness[62]. COX-2 is known to be highly expressed in the adipose tissue of obese individuals and increased circulating levels of cytokines[9]. Increased levels of COX-2 produced by the peri-prostatic white adipose tissue may increase inflammation in the prostate and aid in neoplastic transition and carcinogenesis in the prostate[36]. Additional studies are needed to understand if COX-2 produced from subcutaneous adipose tissue surrounding the prostate can facilitate chronic prostatic inflammation in obese individuals. Table 2 provides an overview of the genes implicated in obesity-driven neoplastic growth of the prostate.
Alteration in gene expression due to obesity and implications in prostate cancer
Obesogenic altered gene expression | Prostate cancer implications | References |
NPY1R | Increased expression of NPY1R promotes lipolysis, allowing prostate cancer cells to use free fatty acids as substrate for growth | Saha et al.[62] |
LEP | Increased expression of LEP in obese individuals promotes cell growth and adipo/lipogenesis | Saha et al.[62] |
ANGPTI | anti-apoptotic and proliferation gene with increased expression in obese individuals | Saha et al.[62] |
HSPB8 | anti-apoptotic and proliferation gene with increased expression in obese individuals | Saha et al.[62] |
FADS1 | Decreased expression in obese individuals results in unsaturation of fatty acids providing cancerous substrate | Saha et al.[62] |
COX-2 | Increased expression promotes inflammation and cell proliferation through prostaglandin | Saha et al.[62] |
The human microbiome, comprising a diverse array of microbial species in the oral cavity and gastrointestinal tract, plays a pivotal role in supporting overall health. Alterations in the microbiome, a condition known as dysbiosis, have been implicated in various cancers, including prostate cancer[63-65]. Elevated levels of pathogenic bacterial species have been found in the prostate and may contribute to chronic inflammation that, in turn, facilitates prostate carcinogenesis. These include Fusobacterium nucleatum, Alistipes, Escherichia coli, Lachnospira, and Sphingomonas[66]. These pathogenic species may contribute to chronic inflammation in the prostate, which is a known risk factor for cancer. Escherichia coli. and other bacterial species are known to cause prostate inflammation. Sexually transmitted infections such as Chlamydia trachomatis, Neisseria gonorrhoeae, and Trichomonas vaginalis have been linked to increased PSA levels possibly signifying infection and prostatic inflammation[67]. Bouts of prostate infection through dysbiosis and subsequent immune responses may lead to chronic inflammation, promoting the transition to cellular plasticity. Chronic inflammation produced by the dysbiosis of the microbiome may lead to the formation of PIA lesions[67]. Cellular damage and oxidative stress produced by chronic infection and inflammation may allow for pro-survival of epithelial cells by overexpression of Bcl-2. Prevention of apoptotic cell death through overexpression of Bcl-2 protein and enhanced growth signal could drive neo-plasticity by increasing the proliferation signal, leading to the formation of prostatic intra-epithelial neoplasia. Liu et al. reported that Fusobacterium nucleatum inhibits apoptosis in cancer cells through the indication of reduced Bax/Bcl-2 ratio[68]. The Bax/Bcl-2 ratio is used as a marker to determine the life or death of cells[68]. The abundance of F. nucleatum has been correlated with supporting or imparting an aggressive tumor phenotype[68]. F. nucleatum, an oral pathogen, has also been linked to prostate cancer. Alluri et al. have demonstrated the presence of F. nucleatum in various prostate conditions, including adenocarcinoma, chronic inflammation, and benign prostatic hyperplasia[69]. The presence of F. nucleatum in the prostate may contribute to increased levels of Bcl-2 expression, as noted by Liu et al. Bcl-2 is an anti-apoptotic protein that prevents programmed cell death and facilitates the formation of PIA or “risk factor lesions”[68]. Inhibition of apoptosis through F. nucleatum could support the proposed mechanism of prostate carcinogenesis. Furthermore, F. nucleatum has been found to be a species of interest as a pathogenic bacteria elevated in cases of colorectal and prostate cancer. In colorectal cancer, F. nucleatum is a producer of short-chain fatty acids (SCFA) that may help induce carcinogenesis in the prostate. Butyrate is a SCFA produced by F. nucleatum that may have implications in prostate cancer through the expression of the insulin-like growth factor 1 (IGF-1)[61]. SCFAs have been found to be elevated in prostate cancer patients along with SCFA-producing bacteria. Matsushita et al. observed that the administration of antibiotics led to a decrease in the levels of fecal SCFAs[61]. Supplementation of SCFA was found to increase IGF-1 levels and activate local mitogen-activated protein kinase (MAPK) and phosphatidylinositol-3-kinase (PI3K) oncogenic pathways, promoting the proliferation in prostate cancer cells[61]. The MAPK signaling pathway controls cellular processes such as proliferation, differentiation, and apoptosis[70]. Similarly, the PI3K pathway plays a role in cell growth, survival, and metabolism. Activation of both pathways promotes the progression of precursor lesions in the prostate. Inhibiting the PI3K and MAPK pathway leads to reduced cell proliferation and increased cell death[71]. Increased expression of Bcl-2 and the proliferation of prostate cancer cells through MAPK or PI3K pathways exerted by F. nucleatum may play a role in the neoplastic transition of prostate tissue. SCFAs have been shown to upregulate toll-like receptors (TLRs), promoting prostate cancer cell migration and activating the NF-κB pathway[72]. The effects of F. nucleatum have been associated with several oncogenic pathways, particularly in colorectal cancer. FomA, a surface protein present on the extracellular vesicles of F. nucleatum, binds to TLR2 on intestinal epithelial cells. This interaction modulates innate immunity and promotes local inflammation, which may contribute to the development of neoplasia[73]. Intestinal epithelial cells exposed to these extracellular vesicles trigger NF-κB activation, a process dependent on TLR2[73]. NF-κB then stimulates the expression of proinflammatory genes, including those for cytokines and chemokines, leading to an inflammatory response[74]. F. nucleatum has been shown to activate the Wnt/β-catenin oncogenic pathway through its protein FadA[75]. This pathway can stimulate neoplastic progression by enhancing the overexpression of inflammatory genes. Additionally, FadA can induce the expression of miR-21, which activates the MAPK/ERK and JAK pathways[76-78]. Downregulation of miR-21 has been associated with increased cell migration in neoplastic diseases[79].
Bacterial taxa associated with cancer cell proliferation
Elevated bacterial taxa | Method/pathway of apoptosis inhibition or cellular proliferation | References |
Fusobacterium nucleatum | - hydrogen sulfide production stimulates CRC cell proliferation - butyrate producer through amino acids can activate MAPK pathways - F. nucleatum extracellular vesicles can activate FADD-RIPK1-caspase3 signaling pathway to promote necrosis in intestinal epithelial cells - FomA can bind to TLR2 on intestinal epithelial cells, thereby modulating innate immunity - Activates the Wnt/B-catenin oncogenic pathway via FadA - FadA induces miR-21 to activate the MAPK/ERK and JAK oncogenic pathway - Facilitates M2 polarization via TLR4/p-PAK1/p-B-catenin S675 cascade - Modulates KRT7-AS/KRT7 to promote metastasis | Wang et al.[83] |
Lactobacillus | Lactic acid increased expression of anti-apoptotic Bcl-2 protein | Yu et al.[82] |
Bacteroides fragilis | - B. fragilis elevation associated with high expression of AXIN, Bcl-2, and CTNNB1 - bft toxin may upregulate MAPK and Wnt signaling pathways | Dadgar-Zankbar et al.[84] |
Clostridiales | Produced SCFAs regulate IGF1 production, which affects MAPK and PI3K pathways | Matsushita et al.[61] |
Rikenellaceae | Produced SCFAs regulate IGF1 production, which affects MAPK and PI3K pathways | Matsushita et al.[61] |
Environmental factors beyond diet, such as exposure to pollutants, have been implicated in prostate carcinogenesis. Vigneswaran et al. conducted a study to explore the link between the Environmental Quality Index (EQI) and the stage of prostate cancer[86]. The EQI assesses exposure across five domains: air, water, land, built environment, and socio-demographic factors. The study found a strong positive correlation between declining environmental quality and the likelihood of metastatic prostate cancer at diagnosis, particularly in the land, water, and socio-demographic domains[86]. Pollutants in these areas were observed to have a dose-dependent relationship with prostate cancer risk. These pollutants are thought to act as endocrine disruptors, disrupting hormonal balance and promoting prostate carcinogenesis.
Chlordecone, also known as Kepone, is an organochlorine insecticide with known carcinogenic effects related to prostate cancer. Multigner et al. investigated chlordecone levels in the plasma of prostate cancer patients and found a marked association between chlordecone exposure and a higher risk of prostate cancer[87]. The study also identified abdominal obesity as a factor that increased prostate cancer risk. The study further proposed that obesity and chlordecone concentration might be inversely related, as peripheral fat could sequester this lipophilic organochlorine compound. Prolonged exposure to chlordecone was deemed necessary to induce prostate cancer, with the risk being particularly high in individuals with a family history of prostate cancer[87].
Exposure to various environmental agents, including pesticides, bisphenol A, polychlorinated biphenyls, and nitrates, has been linked to a higher risk of prostate cancer[87]. A case-control study in Spain investigated the relationship between waterborne nitrate consumption and prostate cancer, finding that ingestion above 13.8 mg per day was associated with a 1.17-fold increase in risk compared to the lowest third percentile (below 5.5 mg per day)[88]. Additionally, a Canadian case-control study examined the impact of ambient air pollution on prostate cancer risk, revealing a strong positive association. The study assessed levels of particulate matter (PM 2.5) and nitrogen dioxide (NO2) over a 20-year period and suggested that air pollution should be classified as a carcinogen due to its association with increased prostate cancer risk[89]. Both individual environmental agents and broader ambient exposures were shown to elevate prostate cancer risk. The interplay of dietary factors, environmental exposure, and obesity thus may contribute to chronic inflammation, potentially facilitating a neoplastic transition within the prostate. Environmental pollutants associated with the risk of prostate cancer are summarized in Table 4.
Environmental carcinogens and their association with prostate cancer risk
Environmental pollutant(s) | Association to prostate cancer risk | References |
Chlordecone | Strong association with increasing prostate cancer risk over long-term exposure | Multigner et al.[87] |
Bisphenol A (BPA) | Increased serum levels of BPA correlated with a higher risk of prostate cancer | Salamanca-Fernández et al.[90] |
Polychlorinated biphenyls (PCB) | Dietary PCB was associated with fatal and high-grade prostate cancer | Ali et al.[91] |
Nitrates | Significant ingestion of waterborne nitrates associated with increased prostate cancer risk | Donat-Vargas et al.[88] |
PM 2.5 | Strong association with increased prostate cancer risk | Youogo et al.[89] |
Nitrogen dioxide | Strong association with increased prostate cancer risk | Youogo et al.[89] |
CONCLUSION AND FUTURE PERSPECTIVES
The potential role of chronic inflammation in the prostate has been observed in biopsies from men of all ages. The proposed mechanism linking chronic inflammation to carcinogenesis involves repeated tissue damage and regeneration, accompanied by the release of highly reactive oxygen and nitrogen species from inflammatory cells. Research conducted by our group and others supports the hypothesis that chronic inflammation plays a key role in the neoplastic transition to prostate cancer. This is evident from the presence of chronic inflammation, which results in the release of reactive oxygen species (ROS), growth factors, and mitogens, leading to damage to the glandular epithelium. Additional support for this hypothesis comes from observations of loss of the basal cell marker p63, increased expression of AMACR, and decreased levels of inflammatory markers. While chronic inflammation is linked to prostate cancer, distinguishing between inflammation as a cause versus a consequence of cancer can be challenging. Furthermore, individual responses to inflammation may vary widely, complicating the establishment of direct causative relationships. Ongoing research is needed to clarify these relationships and identify specific interventions that may reduce the risk of prostate cancer.
Environmental factors significantly influence the development and progression of prostate cancer. Prolonged exposure to environmental toxins, including heavy metals, pesticides, and industrial chemicals, can elevate the risk of developing this disease. Additionally, factors such as infections and irritants may cause chronic inflammation in the prostate, which has been associated with cancer development. The relationship between these mechanisms and environmental influences in prostate cancer is complex and multifaceted. A major limitation is the difficulty in establishing direct causative links between environmental exposures and prostate cancer risk. More research is necessary to clarify these relationships and create effective prevention and treatment strategies tailored to individual risk profiles. Gaining a better understanding of these dynamics can ultimately enhance outcomes for patients at risk for prostate cancer.
The microbiome is an exciting research area for exploring the development and progression of prostate cancer. Its involvement in promoting the transition to neoplastic states via chronic inflammation is increasingly recognized. However, to gain a comprehensive understanding of how pathogenic bacterial species contribute to prostate carcinogenesis, further investigation is necessary regarding the microbiome’s influence on the transition to neoplastic states, particularly through chronic inflammation and various signaling pathways.
Evidence suggests that NSAIDs and a diet rich in fruits and vegetables can help reduce prostate cancer risk. Both NSAIDs and antioxidant-rich diets present promising preventive strategies against prostate cancer, each with unique feasibility, limitations, and clinical applicability. While NSAIDs offer a targeted approach to managing inflammation associated with cancer risk, antioxidant-rich diets provide a holistic lifestyle modification that can improve overall health. To maximize their potential benefits, personalized approaches should be adopted, considering individual patient risk factors, preferences, and existing health conditions. Ongoing research is essential to better understand the mechanisms underlying these preventive strategies, refine recommendations, and ultimately improve patient outcomes in prostate cancer prevention.
Lastly, improving socioeconomic conditions and implementing policies to reduce exposure to environmental pollutants can also decrease prostate cancer risk, particularly in disadvantaged communities. Future studies are essential to deepen our understanding of how chronic inflammation affects prostate carcinogenesis and to develop effective preventative strategies to reduce risk and slow the progression of prostate neoplasia.
DECLARATIONS
Acknowledgments
This manuscript is dedicated to the memory of Dr. Nathan A. Berger in honor of his Special Issue on “Genetic Susceptibility to Obesity-Related Complications.” Dr. Berger was a pioneer in "Transdisciplinary Research in Energetics and Cancer" (TREC) and made numerous other significant research contributions.
Authors’ contributions
Manuscript conceptualization, investigation, writing - original draft preparation: Prakash P, Gupta S
Methodology, data curation: Prakash P, Verma S
writing - review, revising, and editing, visualization: Prakash P, Verma S, Gupta S
Supervision, project administration, funding acquisition, resources: Gupta S
All authors have read and agreed to the published version of the manuscript.
Availability of data and materials
Not applicable.
Financial support and sponsorship
This study was supported by the Department of Defense Grants W81XWH-19-1-0720 to Gupta S.
Conflicts of interest
Sanjay Gupta is an Editor-in-Chief of Journal of Translational Genetics and Genomics. while the other authors have declared that they have no conflicts of interest.
Ethical approval and consent to participate
Not applicable.
Consent for publication
Not applicable.
Copyright
© The Author(s) 2024.
REFERENCES
1. Key Statistics for prostate cancer. 2017; Available from: https://www.cancer.org/cancer/prostate-cancer/about/key-statistics.html [Last accessed on 28 Oct 2024].
3. Lowder D, Rizwan K, McColl C, et al. Racial disparities in prostate cancer: a complex interplay between socioeconomic inequities and genomics. Cancer Lett 2022;531:71-82.
4. Multigner L, Ndong JR, Oliva A, Blanchet P. Polluants environnementaux et cancer de la prostate: données épidémiologiques [Environmental pollutants and prostate cancer: epidemiological data]. Gynecol Obstet Fertil 2008;36:848-56.
5. Di Virgilio F. New pathways for reactive oxygen species generation in inflammation and potential novel pharmacological targets. Curr Pharm Des 2004;10:1647-52.
9. Glover M, Soni S, Ren Q, Maclennan GT, Fu P, Gupta S. Influence of chronic inflammation on Bcl-2 and PCNA expression in prostate needle biopsy specimens. Oncol Lett 2017;14:3927-34.
10. Stark T, Livas L, Kyprianou N. Inflammation in prostate cancer progression and therapeutic targeting. Transl Androl Urol 2015;4:455-63.
11. Sfanos KS, De Marzo AM. Prostate cancer and inflammation: the evidence. Histopathology 2012;60:199-215.
12. Bäckdahl L, Bushell A, Beck S, et al. Inflammatory signalling as mediator of epigenetic modulation in tissue-specific chronic inflammation. Int J Biochem Cell Biol 2009;41:176-84.
13. MacLennan GT, Eisenberg R, Fleshman RL, et al. The influence of chronic inflammation in prostatic carcinogenesis: a 5-year followup study. J Urol 2006;176:1012-6.
14. Putzi MJ, De Marzo AM. Morphologic transitions between proliferative inflammatory atrophy and high-grade prostatic intraepithelial neoplasia. Urology 2000;56:828-32.
15. Kryvenko ON, Jankowski M, Chitale DA, et al. Inflammation and preneoplastic lesions in benign prostate as risk factors for prostate cancer. Mod Pathol 2012;25:1023-32.
16. Porter CM, Shrestha E, Peiffer LB, Sfanos KS. The microbiome in prostate inflammation and prostate cancer. Prostate Cancer Prostatic Dis 2018;21:345-54.
17. Bozeman CB, Carver BS, Eastham JA, Venable DD. Treatment of chronic prostatitis lowers serum prostate specific antigen. J Urol 2002;167:1723-6.
18. De Marzo AM, Marchi VL, Epstein JI, Nelson WG. Proliferative inflammatory atrophy of the prostate: implications for prostatic carcinogenesis. Am J Pathol 1999;155:1985-92.
19. Koochekpour S. Genetic and epigenetic changes in human prostate cancer. Iran Red Crescent Med J 2011;13:80-98.
20. Kohnen PW, Drach GW. Patterns of inflammation in prostatic hyperplasia: a histologic and bacteriologic study. J Urol 1979;121:755-60.
21. Woenckhaus J, Fenic I. Proliferative inflammatory atrophy: a background lesion of prostate cancer? Andrologia 2008;40:134-7.
22. Reznik G, Hamlin MH 2nd, Ward JM, Stinson SF. Prostatic hyperplasia and neoplasia in aging F344 rats. Prostate 1981;2:261-8.
23. Nakayama M, Gonzalgo ML, Yegnasubramanian S, Lin X, De Marzo AM, Nelson WG. GSTP1 CpG island hypermethylation as a molecular biomarker for prostate cancer. J Cell Biochem 2004;91:540-52.
24. Palapattu GS, Sutcliffe S, Bastian PJ, et al. Prostate carcinogenesis and inflammation: emerging insights. Carcinogenesis 2005;26:1170-81.
25. Nakayama M, Bennett CJ, Hicks JL, et al. Hypermethylation of the human glutathione S-transferase-pi gene (GSTP1) CpG island is present in a subset of proliferative inflammatory atrophy lesions but not in normal or hyperplastic epithelium of the prostate: a detailed study using laser-capture microdissection. Am J Pathol 2003;163:923-33.
26. Chen W, Jia L, Gupta S, Maclennan GT. The role of chronic inflammation in prostate carcinogenesis: a follow-up study. Ann Urol Oncol 2019;2:1-8.
27. Enger SM, Van den Eeden SK, Sternfeld B, et al. California men’s health study (CMHS): a multiethnic cohort in a managed care setting. BMC Public Health 2006;6:172.
28. Tewari A, Horninger W, Pelzer AE, et al. Factors contributing to the racial differences in prostate cancer mortality. BJU Int 2005;96:1247-52.
29. Mahal BA, Alshalalfa M, Kensler KH, et al. Racial differences in genomic profiling of prostate cancer. N Engl J Med 2020;383:1083-5.
30. Li D, Zhan Y, Wang N, et al. ETV4 mediates dosage-dependent prostate tumor initiation and cooperates with p53 loss to generate prostate cancer. Sci Adv 2023;9:eadc9446.
33. Leitzmann MF, Rohrmann S. Risk factors for the onset of prostatic cancer: age, location, and behavioral correlates. Clin Epidemiol 2012;4:1-11.
34. Hino O, Kobayashi T. Mourning Dr. Alfred G. Knudson: the two-hit hypothesis, tumor suppressor genes, and the tuberous sclerosis complex. Cancer Sci 2017;108:5-11.
35. Causes of Neoplasia. Available from: http://library.med.utah.edu/WebPath/NEOHTML/NEOPL104.html [Last accessed on 28 Oct 2024].
36. De Nunzio C, Kramer G, Marberger M, et al. The controversial relationship between benign prostatic hyperplasia and prostate cancer: the role of inflammation. Eur Urol 2011;60:106-17.
38. Cavanagh H, Rogers KM. The role of BRCA1 and BRCA2 mutations in prostate, pancreatic and stomach cancers. Hered Cancer Clin Pract 2015;13:16.
39. Alanee SR, Glogowski EA, Schrader KA, Eastham JA, Offit K. Clinical features and management of BRCA1 and BRCA2-associated prostate cancer. Front Biosci 2014;6:15-30.
40. Narod SA, Neuhausen S, Vichodez G, et al. Hereditary Breast Cancer Study Group. Rapid progression of prostate cancer in men with a BRCA2 mutation. Br J Cancer 2008;99:371-4.
41. Molinié V, Hervé JM, Lugagne PM, Lebret T, Botto H. Diagnostic utility of a p63/alpha-methyl coenzyme A racemase (p504s) cocktail in ambiguous lesions of the prostate upon needle biopsy. BJU Int 2006;97:1109-15.
42. Yang A, McKeon F. P63 and P73: P53 mimics, menaces and more. Nat Rev Mol Cell Biol 2000;1:199-207.
43. Signoretti S, Waltregny D, Dilks J, et al. p63 is a prostate basal cell marker and is required for prostate development. Am J Pathol 2000;157:1769-75.
44. Crundwell MC, Chughtai S, Knowles M, et al. Allelic loss on chromosomes 8p, 22q and 18q (DCC) in human prostate cancer. Int J Cancer 1996;69:295-300.
45. Boran C, Kandirali E, Yilmaz F, Serin E, Akyol M. Reliability of the 34βE12, keratin 5/6, p63, bcl-2, and AMACR in the diagnosis of prostate carcinoma. Urol Oncol 2011;29:614-23.
46. Man YG, Gardner WA. Bad seeds produce bad crops: a single stage-process of prostate tumor invasion. Int J Biol Sci 2008;4:246-58.
47. Karaivanov M, Todorova K, Kuzmanov A, Hayrabedyan S. Quantitative immunohistochemical detection of the molecular expression patterns in proliferative inflammatory atrophy. J Mol Histol 2007;38:1-11.
48. Baltaci S, Orhan D, Gögüs C, Türkölmez K, Tulunay O, Gögüs O. Inducible nitric oxide synthase expression in benign prostatic hyperplasia, low- and high-grade prostatic intraepithelial neoplasia and prostatic carcinoma. BJU Int 2001;88:100-3.
49. Aaltoma SH, Lipponen PK, Kosma VM. Inducible nitric oxide synthase (iNOS) expression and its prognostic value in prostate cancer. Anticancer Res 2001;21:3101-6.
50. Klotz T, Bloch W, Volberg C, Engelmann U, Addicks K. Selective expression of inducible nitric oxide synthase in human prostate carcinoma. Cancer 1998;82:897-903.
51. Zha S, Gage WR, Sauvageot J, et al. Cyclooxygenase-2 is up-regulated in proliferative inflammatory atrophy of the prostate, but not in prostate carcinoma. Cancer Res 2001;61:8617-23.
52. De Marzo AM, Platz EA, Sutcliffe S, et al. Inflammation in prostate carcinogenesis. Nat Rev Cancer 2007;7:256-69.
53. Nelson WG, De Marzo AM, Deweese TL, et al. Preneoplastic prostate lesions: an opportunity for prostate cancer prevention. Ann N Y Acad Sci 2001;952:135-44.
54. Yegnasubramanian S. Prostate cancer epigenetics and its clinical implications. Asian J Androl 2016;18:549-58.
55. Lynch BM, Friedenreich CM, Kopciuk KA, Hollenbeck AR, Moore SC, Matthews CE. Sedentary behavior and prostate cancer risk in the NIH-AARP Diet and Health Study. Cancer Epidemiol Biomarkers Prev 2014;23:882-9.
56. Orsini N, Bellocco R, Bottai M, et al. A prospective study of lifetime physical activity and prostate cancer incidence and mortality. Br J Cancer 2009;101:1932-8.
57. Shephard RJ. Physical activity and prostate cancer: an updated review. Sports Med 2017;47:1055-73.
58. Jurdana M. Physical activity and cancer risk. Actual knowledge and possible biological mechanisms. Radiol Oncol 2021;55:7-17.
59. Gathirua-Mwangi WG, Zhang J. Dietary factors and risk for advanced prostate cancer. Eur J Cancer Prev 2014;23:96-109.
60. Rogers LJ, Basnakian AG, Orloff MS, et al. 2-amino-1-methyl-6-phenylimidazo(4,5-b) pyridine (PhIP) induces gene expression changes in JAK/STAT and MAPK pathways related to inflammation, diabetes and cancer. Nutr Metab 2016;13:54.
61. Matsushita M, Fujita K, Hayashi T, et al. Gut microbiota-derived short-chain fatty acids promote prostate cancer growth via IGF1 signaling. Cancer Res 2021;81:4014-26.
62. Saha A, Kolonin MG, DiGiovanni J. Obesity and prostate cancer - microenvironmental roles of adipose tissue. Nat Rev Urol 2023;20:579-96.
63. Yu I, Wu R, Tokumaru Y, Terracina KP, Takabe K. The role of the microbiome on the pathogenesis and treatment of colorectal cancer. Cancers 2022;14:5685.
64. Kustrimovic N, Bombelli R, Baci D, Mortara L. Microbiome and prostate cancer: a novel target for prevention and treatment. Int J Mol Sci 2023;24:1511.
65. Miyabayashi K, Ijichi H, Fujishiro M. The role of the microbiome in pancreatic cancer. Cancers 2022;14:4479.
66. Mjaess G, Karam A, Roumeguère T, et al. Urinary microbiota and prostatic diseases: the key for the lock? Prostate Cancer Prostatic Dis 2023;26:451-60.
67. Sfanos KS, Yegnasubramanian S, Nelson WG, De Marzo AM. The inflammatory microenvironment and microbiome in prostate cancer development. Nat Rev Urol 2018;15:11-24.
68. Liu Y, Baba Y, Ishimoto T, et al. Fusobacterium nucleatum confers chemoresistance by modulating autophagy in oesophageal squamous cell carcinoma. Br J Cancer 2021;124:963-74.
69. Alluri LSC, Paes Batista da Silva A, Verma S, et al. Presence of specific periodontal pathogens in prostate gland diagnosed with chronic inflammation and adenocarcinoma. Cureus 2021;13:e17742.
70. Guo YJ, Pan WW, Liu SB, Shen ZF, Xu Y, Hu LL. ERK/MAPK signalling pathway and tumorigenesis. Exp Ther Med 2020;19:1997-2007.
71. Yang J, Nie J, Ma X, Wei Y, Peng Y, Wei X. Targeting PI3K in cancer: mechanisms and advances in clinical trials. Mol Cancer 2019;18:26.
72. Liu Y, Zhou Q, Ye F, Yang C, Jiang H. Gut microbiota-derived short-chain fatty acids promote prostate cancer progression via inducing cancer cell autophagy and M2 macrophage polarization. Neoplasia 2023;43:100928.
73. Martin-Gallausiaux C, Malabirade A, Habier J, Wilmes P. Fusobacterium nucleatum extracellular vesicles modulate gut epithelial cell innate immunity via FomA and TLR2. Front Immunol 2020;11:583644.
74. Liu T, Zhang L, Joo D, Sun SC. NF-κB signaling in inflammation. Signal Transduct Target Ther 2017;2:17023.
75. Rubinstein MR, Baik JE, Lagana SM, et al. Fusobacterium nucleatum promotes colorectal cancer by inducing Wnt/β-catenin modulator Annexin A1. EMBO Rep 2019;20:e47638.
76. Yang Y, Weng W, Peng J, et al. Fusobacterium nucleatum increases proliferation of colorectal cancer cells and tumor development in mice by activating toll-like receptor 4 signaling to nuclear factor-κB, and up-regulating expression of MicroRNA-21. Gastroenterology 2017;152:851-66.e24.
77. Yu YN, Yu TC, Zhao HJ, et al. Berberine may rescue Fusobacterium nucleatum-induced colorectal tumorigenesis by modulating the tumor microenvironment. Oncotarget 2015;6:32013-26.
78. Jenike AE, Halushka MK. miR-21: a non-specific biomarker of all maladies. Biomark Res 2021;9:18.
79. Chen T, Li Q, Wu J, et al. Fusobacterium nucleatum promotes M2 polarization of macrophages in the microenvironment of colorectal tumours via a TLR4-dependent mechanism. Cancer Immunol Immunother 2018;67:1635-46.
80. Duan H, Wang L, Huangfu M, Li H. The impact of microbiota-derived short-chain fatty acids on macrophage activities in disease: mechanisms and therapeutic potentials. Biomed Pharmacother 2023;165:115276.
81. Chen S, Su T, Zhang Y, et al. Fusobacterium nucleatum promotes colorectal cancer metastasis by modulating KRT7-AS/KRT7. Gut Microbes 2020;11:511-25.
82. Yu T, Ji L, Lou L, et al. Fusobacterium nucleatum affects cell apoptosis by regulating intestinal flora and metabolites to promote the development of colorectal cancer. Front Microbiol 2022;13:841157.
83. Wang S, Liu Y, Li J, et al. Fusobacterium nucleatum Acts as a pro-carcinogenic bacterium in colorectal cancer: from association to causality. Front Cell Dev Biol 2021;9:710165.
84. Dadgar-Zankbar L, Shariati A, Bostanghadiri N, et al. Evaluation of enterotoxigenic Bacteroides fragilis correlation with the expression of cellular signaling pathway genes in Iranian patients with colorectal cancer. Infect Agent Cancer 2023;18:48.
85. Fernandes A, Oliveira A, Guedes C, Fernandes R, Soares R, Barata P. Effect of radium-223 on the gut microbiota of prostate cancer patients: a pilot case series study. Curr Issues Mol Biol 2022;44:4950-9.
86. Vigneswaran HT, Jagai JS, Greenwald DT, et al. Association between environmental quality and prostate cancer stage at diagnosis. Prostate Cancer Prostatic Dis 2021;24:1129-36.
87. Multigner L, Ndong JR, Giusti A, et al. Chlordecone exposure and risk of prostate cancer. J Clin Oncol 2010;28:3457-62.
88. Donat-Vargas C, Kogevinas M, Castaño-Vinyals G, et al. Long-term exposure to nitrate and trihalomethanes in drinking water and prostate cancer: a multicase-control study in Spain (MCC-Spain). Environ Health Perspect 2023;131:37004.
89. Youogo LMK, Parent ME, Hystad P, Villeneuve PJ. Ambient air pollution and prostate cancer risk in a population-based Canadian case-control study. Environ Epidemiol 2022;6:e219.
90. Salamanca-Fernández E, Rodríguez-Barranco M, Amiano P, et al. Bisphenol-A exposure and risk of breast and prostate cancer in the Spanish European prospective investigation into cancer and nutrition study. Environ Health 2021;20:88.
Cite This Article
How to Cite
Download Citation
Export Citation File:
Type of Import
Tips on Downloading Citation
Citation Manager File Format
Type of Import
Direct Import: When the Direct Import option is selected (the default state), a dialogue box will give you the option to Save or Open the downloaded citation data. Choosing Open will either launch your citation manager or give you a choice of applications with which to use the metadata. The Save option saves the file locally for later use.
Indirect Import: When the Indirect Import option is selected, the metadata is displayed and may be copied and pasted as needed.
About This Article
Copyright
Data & Comments
Data
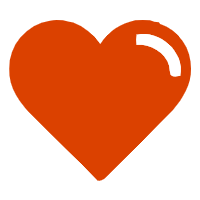
Comments
Comments must be written in English. Spam, offensive content, impersonation, and private information will not be permitted. If any comment is reported and identified as inappropriate content by OAE staff, the comment will be removed without notice. If you have any queries or need any help, please contact us at [email protected].