Time’s imprint on the left atrium: aging and atrial myopathy
Abstract
Aging is a primary driver of atrial remodeling and dysfunction, and contributes to the increasing prevalence of atrial myopathy in the aging population. Atrial myopathy, characterized by structural, functional, and electrophysiological abnormalities of the atria, is a key pathological process underlying adverse cardiovascular outcomes such as atrial fibrillation (AF), heart failure with preserved ejection fraction (HFpEF), and ischemic stroke. Although these outcomes are often treated as distinct clinical entities, emerging evidence suggests that they may represent symptomatic manifestations of an underlying atrial disease process. Aging promotes atrial myopathy through multiple mechanisms, including inflammation, extracellular matrix remodeling, electrophysiological alterations, cellular senescence, epigenetic modifications, and non-coding RNA regulation. These changes collectively lead to atrial fibrosis, impaired mechanical function, conduction abnormalities, and a prothrombotic state. Despite its clinical significance, atrial myopathy remains an underrecognized entity, with current management strategies primarily focusing on treating its downstream complications rather than the underlying disease. Advances in imaging techniques, biomarker discovery, and molecular research have the potential to improve the early detection and risk stratification of atrial myopathy, paving the way for novel therapeutic strategies. In this review, we discuss the structural, mechanical, electrophysiological, and metabolic changes that occur in the aging atrium, explore the cellular and molecular mechanisms that drive these changes, and highlight recent advances in diagnostic and therapeutic approaches. By shifting the focus from managing AF and HFpEF to targeting the underlying atrial myopathy, we can unlock new avenues for prevention and treatment, ultimately improving cardiovascular health in the aging population.
Keywords
INTRODUCTION
Populations worldwide are aging at a rapid rate. By 2030, one in six people globally will be aged 60 years or older, marking a substantial increase in the aging population[1]. As the global population continues to age, the prevalence of cardiovascular diseases such as atrial fibrillation (AF) and heart failure with preserved ejection fraction (HFpEF) has increased[2,3]. These conditions often coexist and are linked by atrial myopathy, a clinical entity defined as structural, functional, and electrophysiological changes in the atria that may underlie adverse cardiovascular outcomes such as AF, HFpEF, and ischemic stroke[4-8].
Aging drives significant left atrial changes, including enlargement, fibrosis, impaired mechanical function, and conduction abnormalities. The clinical implications of atrial myopathy are profound; it is associated with an increased risk of heart failure, AF, stroke, and dementia[9-12]. Despite its clinical importance, current therapeutic strategies often only target the clinical sequelae rather than the underlying atrial myopathy, leaving many with subclinical disease untreated. Addressing atrial myopathy itself could enable earlier intervention and improved outcomes.
This review explores the impact of aging on the left atrium, detailing structural, mechanical, electrophysiological, histological, and metabolic changes, as well as the underlying mechanisms driving these changes. We also examine clinical manifestations and challenges in identifying and managing atrial myopathy in older adults. By emphasizing early detection and intervention, we aim to highlight potential research and therapeutic opportunities.
HEALTHY VS. UNHEALTHY ATRIAL AGING
Aging is an inevitable biological process, but its trajectory varies significantly among individuals. Healthy aging refers to the preservation of physiological function and the absence of disease-related impairments despite advancing age. In the cardiovascular system, this concept extends to healthy atrial aging, where the atria maintain their structural integrity, mechanical function, and electrophysiological stability over time. In contrast, unhealthy atrial aging is characterized by increased fibrosis, impaired mechanical function, elevated arrhythmogenic potential, and a prothrombotic state, predisposing individuals to conditions such as AF, HFpEF, and stroke[6-8]. Understanding the factors that differentiate these aging trajectories is critical for identifying modifiable risk factors and developing preventative strategies.
A key distinction in aging research is the difference between chronological aging and biological aging. Chronological aging simply reflects the passage of time, whereas biological aging refers to the cumulative impact of genetic, environmental, and lifestyle factors on physiological function. In the context of atrial aging, individuals of the same chronological age may exhibit vastly different levels of atrial health due to differences in biological aging.
Several risk factors contribute to accelerated atrial aging, leading to an increased burden of myopathic changes and its associated complications. Diabetes mellitus promotes atrial fibrosis, impaired relaxation, conduction abnormalities, and increased vulnerability to atrial arrhythmias[13,14]. These changes are mediated at least in part by advanced glycation end products and their associated receptor and upregulation of connective tissue growth factor[15]. Cigarette smoking is another major contributor to accelerated atrial aging; nicotine-induced upregulation of collagen III promotes atrial fibrosis at a younger age, and there is a strong correlation between pack-years and the extent of atrial fibrosis[16]. Hypertension, a common comorbidity in aging adults, is associated with impaired left atrial function as measured by speckle-tracking echocardiography, even after adjustment for left ventricular size and function[17]. In addition to environmental and metabolic risk factors, genetic predisposition also plays a role in determining atrial aging trajectories. Variants in genes such as natriuretic peptide precursor A, myosin light-chain 4, and titin have been implicated in pathological atrial remodeling[18-20].
Another notable contributor to unhealthy atrial aging is cardiac amyloidosis, which is characterized by the extracellular deposition of misfolded amyloid proteins in the myocardium. This typically occurs as either light chain amyloidosis or transthyretin amyloidosis. Atrial amyloidosis impairs atrial function and increases the risk of AF and thromboembolism[21]. Notably, LA mechanical function is severely impaired in cardiac amyloidosis[22]. An analysis of explanted hearts from five patients with transthyretin cardiac amyloidosis revealed mild-moderate subendocardial fibrosis in all samples[23]. AF is frequently observed in cardiac amyloidosis, with one study reporting a prevalence of 44% among 238 patients[24]. Patients with atrial amyloidosis also have a high incidence of intracardiac thrombosis, even in the absence of AF or despite adequate anticoagulation, placing them at significant risk for thromboembolic events[25-28]. A retrospective study of 406 patients with cardiac amyloidosis found that 7.6% experienced an arterial thromboembolic event, with one-third of these cases occurring in individuals who were in sinus rhythm and had no history of AF[29].
EFFECTS OF AGING ON THE ATRIA
We start by reviewing the effects of aging on atrial structure, mechanical function, electrophysiological function, and metabolic function [Table 1].
Effects of aging on atrial structure, mechanical function, electrophysiological function, and metabolic function
Category | Age-related changes | Reference |
Structure | Left atrial enlargement | Singh et al., 2022[30] |
Medrano et al., 2016[31] | ||
Pan et al., Chest, 2008[32] | ||
Increased atrial fibrosis | Lin et al., 2020[35] | |
Gramley, 2009[36] | ||
Jansen et al., 2021[37] | ||
Decreased myocyte density | Takahashi et al., 2023[38] | |
Increased myocyte hypertrophy | Clarke et al., 2017[39] | |
Hayashi et al., 2002[40] | ||
Inflammatory cell infiltration | Wu et al., 2020[41] | |
Tan et al., 2023[42] | ||
Mechanical function | Decreased reservoir strain | Sugimoto et al., 2018[46] |
Nyberg et al., 2023[47] | ||
Singh et al., 2022[30] | ||
Zhang et al., 2023[48] | ||
Abou et al., 2017[49] | ||
Liao et al., 2017[50] | ||
Decreased conduit strain | Sugimoto et al., 2018[46] | |
Nyberg et al., 2023[47] | ||
Singh et al., 2022[30] | ||
Zhang et al., 2023[48] | ||
Liao et al., 2017[50] | ||
Increased contractile strain | Singh et al., 2022[30] | |
Liao et al., 2017[50] | ||
Boyd et al., 2011[51] | ||
Electrophysiological Function | Abnormal P-wave terminal force in V1 | Eranti et al., 2014[53] |
Wolder et al., 2023[54] | ||
Increased P-wave duration | Turhan et al., 2003[55] | |
Jansen et al., 2017[44] | ||
Increased P-wave dispersion | Abou et al., 2017[49] | |
Increased low voltage areas and conduction block | van der Does et al., 2021[60] | |
Kistler et al., 2004[61] | ||
Metabolic function | Impaired oxidative phosphorylation | Emelyanova et al., 2018[65] |
Impaired phosphotransfer and ATP cycling | Nemutlu et al., 2015[66] | |
Altered fatty acid metabolism | Lee et al., 2019[67] |
Structure
Aging leads to significant structural and histological changes in the atria, which contribute to the development of atrial myopathy in older individuals. Morphologically, the size of the left atrium increases with age, a phenomenon attributed to both dilation and hypertrophy of the atrial wall[30-32]. This enlargement is a compensatory response to increased atrial pressure and volume overload, commonly due to age-related conditions such as uncontrolled hypertension and left ventricular diastolic dysfunction[33,34]. Histologically, one of the most prominent age-related changes is the increased fibrosis within the atrial tissue, contributing to decreased atrial compliance and arrhythmogenic substrate[35-37]. Concurrently, there is a decrease in myocyte density and an increase in myocyte hypertrophy, which likely contributes to the impaired atrial mechanical function commonly observed in older individuals[38-40]. Furthermore, inflammatory cell infiltration is observed more frequently, contributing to pathological atrial remodeling[41,42]. The right atrium also undergoes age-related remodeling, including enlargement and fibrosis[43,44]. However, this review primarily focuses on left atrial aging because the predominant relevant clinical outcomes - AF, HF, and stroke - are primarily associated with left atrial myopathy.
Mechanical function
The left atrium is a major contributor to left ventricular filling. It serves as a reservoir for pulmonary venous return during ventricular systole, a conduit during early ventricular diastole, and a booster pump that augments late ventricular filling during ventricular diastole[45]. Aging is associated with a decrease in left atrial reservoir strain, indicative of impaired left atrial compliance[30,46-50]. This decline in reservoir function is likely, in part, due to atrial fibrosis, which has been shown to increase with age[35-37]. Conduit function, much like reservoir function, also seems to decrease significantly with increasing age[30,46-48,50]. Conversely, there often appears to be a compensatory increase in left atrial booster function with advancing age, suggesting that active ventricular filling plays a larger role in maintaining preload[30,50,51]. Collectively, these findings reflect alterations in left atrial mechanical function with increasing age [Table 2].
Echocardiographic reference ranges for left atrial size and strain across different age groups
Source | aSugimoto et al., 2018[46] | bNyberg et al., 2023[47] | cSingh et al., 2022[30] | ||||||||
Age range (years) | 20-40 | 40-60 | ≥ 60 | < 40 | 40-49 | 50-59 | 60-69 | ≥ 70 | 18-40 | 41-65 | > 65 |
Left atrial maximal volume index (mL/m2) - males | - | - | - | - | - | - | - | - | 16-41 | 16-46 | 18-48 |
Left atrial maximal volume index (mL/m2) - females | - | - | - | - | - | - | - | - | 17-39 | 18-43 | 18-47 |
Reservoir strain (%) - overall | 31 | 28 | 23 | - | - | - | - | - | - | - | - |
Reservoir strain (%) - males | - | - | - | 24-53 | 21-53 | 20-49 | 16-46 | 13-40 | 25-63 | 23-61 | 24-57 |
Reservoir strain (%) - females | - | - | - | 29-53 | 22-53 | 21-48 | 17-44 | 14-39 | 54-81 | 48-78 | 43-76 |
Conduit strain (%) - overall | 16 | 12 | 12 | - | - | - | - | - | - | - | - |
Conduit strain (%) - males | - | - | - | 13-36 | 8-32 | 7-27 | 3-23 | 1-19 | 18-50 | 12-43 | 10-36 |
Conduit strain (%) - females | - | - | - | 15-37 | 10-34 | 9-28 | 4-23 | 2-19 | 19-52 | 12-42 | 9-36 |
Contractile strain (%) - overall | 7 | 9 | 8 | - | - | - | - | - | - | - | - |
Contractile strain (%) - males | - | - | - | 5-23 | 7-28 | 8-27 | 8-28 | 6-26 | 2-23 | 5-28 | 9-32 |
Contractile strain (%) - females | - | - | - | 8-22 | 6-25 | 7-26 | 8-26 | 7-25 | 2-21 | 6-28 | 7-30 |
Electrophysiological function
Age-related electrophysiological changes in the atria are clinically significant and are often reflected in distinct alterations in electrocardiogram (ECG) patterns and electrical mapping studies. P wave parameters, in particular, can offer insight into atrial structure, size, and electrical activation[52]. Abnormal P-wave terminal force in V1, characterized by a significant negative component of the P wave in V1, is more frequently observed with increasing age[53,54]. This abnormal P wave morphology is thought to reflect left atrial enlargement or, as more recent research suggests, disruption of posterior interatrial conduction pathways due to structural remodeling and atrial fibrosis[52]. Additionally, in older individuals, the P-wave duration on ECG, which represents atrial depolarization, is often prolonged, indicating delayed intra-atrial conduction[44,55]. This is accompanied by an increase in P-wave dispersion, a marker of heterogeneous atrial conduction[49]. Abnormal P-wave terminal force, as well as increases in both P-wave duration and dispersion, have been associated with a higher risk of AF[53,54,56-59]. Electrical mapping of the atria in older adults frequently reveals areas of low voltage and conduction block, consistent with atrial fibrosis and remodeling[60,61]. These areas can be considered markers of arrhythmogenic tissue, contributing to the development and maintenance of atrial arrhythmias[62-64].
Metabolism
Age-related metabolic changes in the atria are characterized by a decline in mitochondrial function and disruptions in energy transfer pathways, contributing to atrial vulnerability. A recent study demonstrated significant downregulation of genes coding for mitochondrial proteins in the atria, particularly those involved in oxidative phosphorylation[65]. This downregulation was most pronounced in complex I subunits, resulting in reduced complex I activity and a decline in the respiratory capacity of mitochondria oxidizing NADH-dependent substrates. Additionally, using advanced 18O stable isotope-based phosphometabolomic technology, another study revealed impaired phosphotransfer through adenylate kinase, creatine kinase, glycolytic pathways, and the glycerol-3-phosphate shuttle in the atrial myocardium of aged rats, indicating impaired energetic communication and ATP cycling[66]. This energetic deficit may increase the susceptibility of aged atria to stress. Further supporting the role of metabolic alteration in atrial aging, treatment with empagliflozin in aged spontaneously hypertensive rats was found to reduce left atrial dilation and attenuate atrial fibrosis[67]. Interestingly, empagliflozin also significantly decreased the age-related upregulation of peroxisome proliferator-activated receptor alpha and acyl-CoA dehydrogenase medium chain, both of which are involved in fatty acid metabolism, suggesting a potential therapeutic role in modulating metabolic pathways to mitigate age-related atrial remodeling. These findings underscore the critical impact of metabolic dysfunction on the aging atrium and its potential as a therapeutic target.
In summary, aging leads to significant changes in the atria that contribute to the development of atrial myopathy. Structural enlargement, mechanical dysfunction, electrophysiologic alterations, and metabolic dysfunction are common in advanced age. These changes are underpinned by histological modifications which compromise atrial function. Collectively, these factors illustrate how aging predisposes the atria to pathological remodeling, ultimately increasing the risk of atrial myopathy.
MECHANISMS LINKING AGING WITH ATRIAL MYOPATHY
Age-related inflammation, extracellular matrix remodeling, electrophysiological remodeling, cellular senescence, epigenetic modifications, and non-coding RNAs contribute to pathological atrial remodeling [Figure 1]. Collectively, these mechanisms of age-related atrial myopathy alter the structural integrity of the atrial myocardium and disrupt the normal function of atrial cardiomyocytes, fibroblasts, and endothelial cells, thus leading to pathological atrial remodeling.
Figure 1. Mechanisms contributing to age-related atrial remodeling. Several mechanisms contribute to age-related pathological atrial remodeling: (1) a chronic inflammatory state marked by elevated circulating pro-inflammatory cytokines, including interleukin (IL)-6,
Inflammation
Inflammation plays a pivotal role in the mechanisms that link aging to atrial myopathy, contributing significantly to the structural and functional deterioration of the atrial myocardium. As the body ages, there is a chronic low-grade inflammatory state, often referred to as “inflammaging”, characterized by increased levels of circulating pro-inflammatory factors such as interleukin-6, interleukin-15, and interleukin-18[68]. This persistent inflammatory state promotes the recruitment of immune cells to atrial tissue[41,42]. The presence of these immune cells in the atria exacerbates the production of fibrotic factors, enhancing collagen deposition and the formation of fibrotic patches within the atrial myocardium[69-71]. Such fibrotic remodeling impairs atrial compliance and contractility, and disrupts the normal electrical conduction pathways, increasing the risk for arrhythmias such as AF[72,73]. These processes underline the critical role of inflammation as a driver of pathological remodeling associated with atrial myopathy in aging adults.
Extracellular matrix remodeling
Extracellular matrix remodeling is a central mechanism in the progression of atrial myopathy in the aging heart, marked by the excessive accumulation of collagen within atrial tissue[35-37]. As individuals age, the regulatory balance between matrix synthesis and degradation becomes disrupted, often tipping in favor of matrix deposition. This shift is mediated by an increase in pro-fibrotic factors such as transforming growth factor beta and dysregulation of matrix metalloproteinases and tissue inhibitors of matrix metalloproteinases[74,75]. These fibrotic areas contribute to atrial mechanical dysfunction, particularly impaired reservoir function[73]. Additionally, the fibrotic remodeling of the atrial myocardium impairs normal atrial electrical impulse conduction and promotes electrical heterogeneity, thereby increasing the risk for atrial arrhythmias[72,76].
Electrophysiological remodeling
Beyond the conduction disturbances associated with fibrosis, age-related electrophysiological remodeling in the atria is driven by a complex interplay of molecular and cellular changes that alter the electrical properties of atrial cardiomyocytes. Research on the effects of aging on the expression and function of ion channels in the atria is limited and sometimes contradictory. For instance, studies indicate that NaV1.5, the predominant cardiac voltage-gated sodium channel, is upregulated in the atria of aged rats[77]. Conversely, research in aged canines shows no change in sodium current density or structural remodeling of NaV1.5 in aged atrial cells compared to young controls[78]. However, the same study did note a significant acceleration into the inactivated state and an enhanced use-dependent decrease in peak current in aged right atrial cells, but interestingly not in left atrial cells. Additionally, findings in aged canines revealed that right atrial cells exhibit reduced L-type calcium currents and augmented transient outward and sustained potassium currents[79]. Although the body of literature is limited, these studies collectively suggest that aging is associated with alterations in ion channel expression and function in the atria, which may contribute to increased arrhythmogenic potential. Furthermore, there is evidence that aging is associated with the downregulation of connexin-43, a gap junction protein, in the atria[80]. This likely results in impaired electrical conduction between atrial myocytes. These age-related modifications in ion channel dynamics and gap junction connectivity collectively dimmish the atrial myocardium’s electrical stability.
Cellular senescence
Cellular senescence is characterized by a state of growth arrest, resistance to apoptosis, and the acquisition of a senesce-associated secretory phenotype, which includes the secretion of pro-inflammatory molecules and enzymes involved in extracellular matrix remodeling[81]. Senescent cells are distinct from quiescent cells, which are temporarily non-dividing but can re-enter the cell cycle, and from terminally differentiated cells, which are permanently non-dividing but reach this state through a developmentally programmed process[82]. Senescent cells accumulate in the heart over time and contribute to age-related cardiovascular diseases[83]. The impact of cellular senescence on the cardiovascular system is complex; however, recent evidence suggests it may adversely affect atrial function. A study by Jesel et al. investigated right atrial appendage tissue from patients with sinus rhythm, paroxysmal AF, or permanent AF[84]. They observed a progressive increase in the expression of senescence markers p16 and p53 across these groups, indicating an association between AF progression and human atrial senescence burden[84]. The downstream effects of cellular senescence depend on the cell type. Cardiomyocytes, being terminally differentiated cells, do not undergo senescence in the conventional sense. Instead, cardiomyocyte senescence is characterized by contractile dysfunction, hypertrophic growth, increased pacing frequency, mitochondrial dysfunction, and DNA damage[85]. Although senescent cardiomyocytes do not exhibit a typical senescence-associated secretory phenotype, they release factors such as endothelin-3, transforming growth factor beta-2, and growth differentiation factor 15 that promote myofibroblast activation and cardiomyocyte hypertrophy[86]. Senescent atrial fibroblasts likely contribute to cardiac remodeling by expressing increased levels of fibrotic factors such as collagen, transforming growth factor beta, and matrix metalloproteinase-2 and 9[87]. Additionally, senescent atrial endothelial cells show enhanced expression of vascular cell adhesion molecule-1, transforming growth factor beta, matrix metalloproteinase-2 and -9, and tissue factor, potentially contributing to atrial inflammation, remodeling, and a prothrombotic state[88]. Collectively, these effects likely contribute to aging-associated atrial myopathy.
Epigenetic modifications
Aging is characterized by several epigenetic changes, including loss of histones, an imbalance between activating and repressive histone modifications, alterations in DNA methylation, and chromatin remodeling[89]. Growing evidence suggests that these epigenetic changes play a significant role in age-related cardiovascular diseases[90]. A recent study evaluated the association between epigenetic age acceleration - a measure of biological aging based on epigenetic modifications - and the risk of incident AF[91]. The study found that increases in epigenetic age acceleration were significantly associated with a higher risk of developing AF, even after adjusting for chronological age and other risk factors. These findings suggest that age-related epigenetic modifications may contribute to the pathogenesis of AF, highlighting a potential mechanism linking biological aging to atrial myopathy. While specific age-related epigenetic modifications that directly link aging to atrial myopathy have yet to be fully identified, existing evidence connects certain epigenetic alterations with AF and atrial remodeling. For instance, a methylome-wide association study in a community-based cohort identified seven CpG sites that were associated with AF, indicating that DNA methylation may contribute to AF arrhythmogenesis[92]. Additionally, hypermethylation of paired-like homeodomain 2, a key regulatory gene, in the left atrium has been associated with AF and LA dilation[93]. Beyond changes in DNA methylation, histone modifications also appear to contribute to the pathogenesis of atrial myopathy. Downregulation of histone deacetylase 2 is associated with AF and has been shown to cause altered potassium channel expression and action potential prolongation[94]. Moreover, the expression of enhancer of zeste homolog 2, a histone-lysine N-methyltransferase enzyme, is upregulated in the atrial tissue of patients with atrial fibrillation, and this increase is associated with significant atrial fibrosis and fibroblast differentiation[95]. Similar upregulation of enhancer of zeste homolog 2 and increased methyltransferase activity were observed in a mouse model of atrial fibrosis induced by angiotensin II infusion. Together, these findings suggest that epigenetic modifications, which are hallmarks of aging, likely contribute to age-related atrial remodeling and the development of atrial myopathy.
Non-coding RNAs
Non-coding RNAs, including microRNAs and long non-coding RNAs, have recently described roles in age-related cardiovascular disease[96,97]. In mice, microRNA-22 is upregulated with aging and promotes cellular senescence and fibroblast activation[98]. Similarly, mircoRNA-34a, which is also upregulated with age, contributes to age-related cardiac cell death and functional decline by inhibiting PNUTS, a regulator that protects against telomere shortening[99]. Conversely, SARRAH, a long non-coding RNA that supports cardiomyocyte survival by forming a triple helix structure with promoter regions to enhance the expression of cardiac survival genes, is downregulated with aging[100]. Although research on aging-regulated non-coding RNAs in atrial myopathy remains limited, several non-coding RNAs have been implicated in pathological atrial remodeling. MicroRNA-21, which is upregulated in the left atrium of AF patients and positively correlated with atrial collagen content, plays a role in angiotensin II-mediated atrial remodeling[101]. MicroRNA-328, another microRNA elevated in AF, contributes to adverse electrical remodeling by targeting L-type calcium channel genes[102]. Additionally, microRNA-26 has been identified as a determinant of AF vulnerability, as it regulates the expression of KCNJ2, a key component of inward rectifier potassium current[103]. Further research is needed to identify non-coding RNAs involved in age-related atrial myopathy.
CLINICAL IDENTIFICATION AND MANIFESTATIONS OF ATRIAL MYOPATHY IN THE CONTEXT OF AGING
Identifying atrial myopathy in the aging population requires a comprehensive approach, integrating clinical assessment, imaging, and electrophysiological studies. A thorough history and physical examination focusing on symptoms of arrhythmias, HF, and thromboembolic events is essential. Identifying risk factors such as hypertension, valve disease, diabetes, and previous cardiac events is critical, as these conditions often coexist with atrial myopathy in aging adults[104]. Electrocardiography remains the cornerstone for detecting AF and other supraventricular tachycardias. Abnormal P-wave terminal negative force in V1 may reflect left atrial enlargement of disruption of interatrial conduction pathways due to fibrotic remodeling. Furthermore, invasive electrophysiological studies such as atrial voltage mapping can identify areas of low-voltage reflecting atrial fibrosis and arrhythmogenic foci[62,64].
Historically, electrocardiography was the primary method for identifying atrial myopathy. However, echocardiography has emerged as a more comprehensive tool for evaluating left atrial structure and function. Transthoracic echocardiography enables the measurement of atrial volume and strain, with enlarged atria and reduced strain being hallmarks of myopathy[105-108]. Left atrial strain, especially reservoir strain, has gained prominence as a reliable marker of atrial function[109-112]. Although LA strain measurement is not yet widely implemented in clinical practice, the standardization of deformation imaging techniques and ongoing refinement of normal reference ranges are paving the way for its future widespread adoption[30,46,47,113-115]. However, despite demonstrating statistically significant changes in mean values across studies, left atrial strain often exhibits substantial overlap in standard deviations, complicating the interpretation of individual patient measurements. Further validation is needed to enhance its utility in routine clinical assessment. Doppler studies provide insights into diastolic function and atrial contribution to ventricular filling. Pulsed wave Doppler measurements of peak transmitral flow velocity during late diastolic filling have been used in several studies to assess left atrial contractile function[116,117]. However, the utility of this measure is limited, as transmitral flow patterns are influenced by various factors, including age, heart rate, loading conditions, and left ventricular diastolic properties[118]. Tissue Doppler imaging of the mitral annulus during atrial contraction is another measure of left atrial function, and has been shown to correlate with left atrial ejection fraction, ejection force, and kinetic energy[119]. Cardiac magnetic resonance imaging (MRI) offers detailed visualization of atrial structure and fibrosis, with late gadolinium enhancement identifying areas of atrial scarring[120]. Computed tomography imaging can evaluate atrial anatomy, wall thickness, and is a reliable method for detecting thrombi within the left atria or left atrial appendage[121,122].
Several serum biomarkers that may indicate atrial myopathy have been identified, though none have been widely adopted specifically for this purpose in clinical practice. In a study of HFpEF patients enrolled in the RELAX trial, elevated levels of NT-proBNP, endothelin-1, and troponin I were found to correlate with reduced left atrial reservoir and contractile strain, suggesting that these markers may reflect underlying atrial dysfunction[123]. Furthermore, repeated biomarker measurements at the 24-week follow-up demonstrated that higher left atrial reservoir strain was independently associated with a reduction in NT-proBNP, indicating a potential dynamic relationship between atrial strain and circulating biomarkers. These findings underscore the potential usefulness of serum biomarkers for monitoring left atrial function.
Atrial myopathy presents various clinical manifestations [Table 3] that become particularly nuanced in the older population due to the combined effects of age-related physiological decline and the prevalence of comorbid conditions[124,125]. Among these manifestations, AF stands out as the most common and significant[6,8,126,127]. Age is recognized as the most critical risk factor for AF, with epidemiological studies indicating that nearly one-third of individuals aged 85 years or older are affected by this arrhythmia[128,129]. In addition to AF, other arrhythmias such as atrial flutter may also develop, further complicating the clinical picture of atrial myopathy in older adults[130,131]. The clinical implications of AF are profound, including an increased risk of stroke, HF, and mortality[132-134]. Given these severe consequences, there is an ongoing need to improve prevention strategies. Shifting the focus from AF to atrial myopathy may uncover new, potentially more effective preventative approaches by targeting the underlying substrate of atrial dysfunction. Additionally, while current guidelines do not recommend routine screening for AF, this shift in focus could eventually result in sufficient evidence to support screening for atrial myopathy.
Markers and clinical manifestations of atrial myopathy
Markers of atrial myopathy | Reference |
PTFV1 | Chen et al., 2022[52] |
Low-voltage areas | Sim et al., 2019[62] |
Yamaguchi et al., 2022[64] | |
Left atrial enlargement | Lisi et al., 2022[106] |
Patel et al., 2009[107] | |
Decreased left atrial strain | Lisi et al., 2022[106] |
Vieira et al., 2014[105] | |
Kuppahally et al., 2010[108] | |
Late gadolinium enhancement | Siebermair et al., 2017[120] |
Oakes et al., 2009[151] | |
Mitral inflow velocity during late diastole | Thomas et al., 2020[118] |
Mitral annular velocity during atrial contraction | Khankirawatana et al., 2004[119] |
Clinical manifestations | |
Atrial fibrillation | Goldberger et al., 2015[6] |
Rivner et al., 2020[126] | |
Kallergis et al., 2014[127] | |
Shen et al., 2019[8] | |
HFpEF | Omote et al., 2023[7] |
Melenovsky et al., 2015[136] | |
Patel et al., 2021[137] | |
Tamargo et al., 2020[138] | |
Deedwania et al., 2010[139] | |
Thromboembolism | Goldberger et al., 2015[6] |
Shen et al., 2019[8] | |
Smietana et al., 2019[140] | |
Tan et al., 2023[42] |
HF, particularly HFpEF, is another significant clinical manifestation of atrial myopathy in older individuals. Age is also the leading risk factor for the development of HFpEF, making this condition increasingly prevalent in the aging population[135]. Atrial myopathy is one of the most important contributors to disease progression in HFpEF because it leads to left atrial dysfunction, which is closely associated with increased pulmonary vascular resistance and right ventricular dysfunction in HF patients[7,136]. Indeed, atrial myopathy in HFpEF is characterized by increased atrial stiffness and higher peak atrial pressures, distinct from other forms of HF[136]. Furthermore, recent evidence suggests the existence of a unique HFpEF phenotype characterized by disproportionate atrial myopathy with preserved diastolic function, indicating that atrial myopathy may represent a distinct pathophysiological process in HFpEF development rather than merely a consequence of left ventricular dysfunction[137]. Additionally, atrial myopathy may contribute to the development of functional mitral regurgitation in patients with HFpEF, which further reduces cardiac output[138]. Atrial arrhythmias, particularly AF, may also develop, reducing the atrial contribution to left ventricular filling and thereby worsening cardiac output[139].
Thromboembolism is another critical manifestation of atrial myopathy, particularly in aging adults, where it poses a significant risk for morbidity and mortality. Atrial myopathy and the associated atrial remodeling create a prothrombotic state, which may increase the risk of cardioembolic strokes, independent of the presence of AF[6,8,140]. Brambatti et al. suggest that this is the most likely explanation for the lack of temporal association between paroxysmal AF and stroke[141]. Recent findings by Tan et al. provide compelling evidence for this association. In a study involving 180 aging male mice, 20 mice experienced stroke events despite the absence of AF[42]. Notably, these mice exhibited left atrial appendage thrombi, enlarged left atria, and significant endocardial remodeling within the left atrium. These findings underscore the need for careful risk stratification and consideration of anticoagulation in older patients with atrial myopathy, even in the absence of AF, to mitigate the risk of stroke.
RECENT ADVANCEMENTS
Emerging technologies
Emerging technologies are poised to significantly enhance the detection and monitoring of atrial myopathy. High-resolution imaging modalities, including advanced echocardiography and cardiac MRI, can provide detailed insights into atrial structure and function, enabling the detection of subtle changes that may indicate early atrial myopathy[142-144]. Wearable devices such as smartwatches enable real-time tracking of cardiac rhythms and other physiological parameters, thereby facilitating the early identification of atrial dysfunction. Studies have shown that smartwatches can be used to detect various supraventricular arrhythmias, and can identify AF with relatively high sensitivity and specificity[145,146]. Moreover, artificial intelligence-based tools are being rapidly developed to enhance the assessment of atrial function, enabling more accurate detection of subtle atrial abnormalities and early identification of disease. These artificial intelligence tools have demonstrated significant potential in analyzing electrocardiographic and imaging data[147-150]. Together, these advancements hold the promise of transforming the clinical management of atrial myopathy, enabling earlier intervention, more precise monitoring, and ultimately improving patient outcomes.
While these emerging technologies provide valuable tools for detecting atrial dysfunction, the ability to directly identify the underlying atrial substrate, such as atrial fibrosis, may enable even earlier detection and more targeted therapeutic strategies for atrial myopathy before overt dysfunction occurs. Late gadolinium enhancement MRI has been proposed as a non-invasive technique to detect areas of fibrotic tissue within the atria[120,151]. This imaging modality works by leveraging the differential uptake and washout of gadolinium contrast agent between healthy and fibrotic myocardial tissue; fibrotic regions retain gadolinium longer than normal myocardium due to increased extracellular volume, resulting in areas of hyperenhancement on MRI. While quantification of atrial fibrosis by late gadolinium enhancement MRI has demonstrated some clinical utility in predicting arrhythmia recurrence following catheter ablation of AF, it remains an indirect measure of fibrotic burden, may not fully capture the complexity of the fibrotic substrate, and carries technical challenges that may limit broad implementation[152]. Furthermore, its clinical application has yielded mixed results; the DECAAF II trial evaluating MRI-guided fibrosis ablation in conjunction with pulmonary vein isolation did not show a significant reduction in atrial arrhythmia recurrence compared to pulmonary vein isolation alone[153]. This outcome underscores the need for further refinement of imaging techniques and more targeted interventions to effectively address the underlying fibrotic process in atrial myopathy.
Clinical utility of left atrial size and strain measurements
Left atrial size and strain measurements are increasingly recognized as valuable clinical tools for predicting adverse cardiovascular outcomes. Increased left atrial size, a well-established marker of atrial myopathy, has been linked to new-onset congestive HF, postoperative AF, and increased mortality in patients with acute myocardial infarction[154-156]. In particular, minimal left atrial volumes, as opposed to maximal volumes, are not affected by longitudinal left ventricular systolic motion and have been more strongly associated with adverse clinical outcomes[157,158]. More recently, left atrial strain, particularly reservoir strain, has gained attention as a sensitive indicator of both subclinical and overt cardiovascular conditions. Notably, left atrial strain has emerged as a predictor of subclinical AF in older patients at risk of stroke, offering the potential for early detection and intervention[109]. Additionally, decreased left atrial strain has been associated with a higher incidence of ischemic stroke, highlighting its potential role in stroke risk stratification[110]. Furthermore, in patients with congestive HF, reduced reservoir strain correlates with diminished exercise capacity, reinforcing its utility in assessing functional status[111]. Importantly, left atrial reservoir stain has also been identified as a predictor of all-cause mortality and cardiac hospitalization in HF patients[112]. These findings underscore the potential of left atrial measurements not only as diagnostic tools but also as prognostic markers that could guide therapeutic strategies and improve patient outcomes in clinical practice.
CURRENT CHALLENGES
Lack of diagnostic criteria
Despite the growing recognition of atrial myopathy as a distinct clinical entity, there are currently no established diagnostic criteria to accurately diagnose this condition. This lack of standardized criteria poses a significant challenge in both clinical practice and research, making it difficult to assess the true prevalence, progression, and impact of atrial myopathy. There are well-established markers of atrial myopathy that are identifiable via the use of electrocardiography, cardiac imaging, and electrophysiological studies; however, the absence of a unified diagnostic framework means that these findings are often interpreted in isolation. This gap underscores the need for comprehensive criteria that integrate these markers to facilitate earlier and more accurate identification of atrial myopathy, particularly in older patients where the condition is more prevalent[128]. Notably, Kreimer and Gotzmann recently proposed an algorithm for diagnosing atrial myopathy that combines findings from cardiac magnetic resonance imaging, electrophysiological studies, echocardiography, and electrocardiography[159]. This algorithm classifies markers of atrial myopathy into three categories: strong evidence (e.g., low-voltage area > 10%), supporting findings (e.g., decreased LA emptying fractions), and uncertain findings (e.g., LA blood flow velocities). This proposed algorithm may provide a potential foundation for developing a robust and reliable approach to diagnosing atrial myopathy.
Current limitations of LA imaging techniques
Although advancements in imaging techniques have improved the assessment of abnormal LA structure and function, several limitations remain. A review by Olsen et al. discusses key challenges in echocardiographic, MRI, and computed tomography assessment of the LA[160]. Echocardiographic assessment of LA volumes and strain is hindered by methodological variability, including inconsistencies in chamber delineation, tracking techniques, and ECG reference points. Additionally, LA strain values are influenced by technical factors such as atrial wall structure, pulmonary venous anatomy, the inclusion of the interatrial septum, and vendor-dependent measurement differences. Cardiac magnetic resonance with late gadolinium enhancement imaging provides valuable insights into atrial fibrosis but presents technical challenges. Imaging the thin atrial wall requires precise ECG and respiratory gating, as well as expertise in opitmization of inversion times for myocardial nulling. Post-processing is time-intensive, and the lack of standardized segmentation and thresholding methods limits reproducibility across centers. Similarly, although computed tomography offers detailed structural assessment, its clinical utility in atrial myopathy characterization is limited by radiation exposure and the need for iodinated contrast administration. Collectively, these limitations underscore the need for further methodological standardization to enhance the clinical applicability of these imaging techniques.
Additional pathophysiologic insights needed
Understanding the intricate pathophysiology of atrial myopathy in the context of aging remains a significant challenge. The interplay between age-related structural, functional, and electrophysiological changes in the atria and broader cardiovascular system is multifaceted, complicating efforts to identify specific molecular targets for intervention and confounding the development of effective therapeutic strategies. Findings from our recent study in which we characterized left atrial function in three different mouse models of atrial myopathy - aging, HFpEF, and angiotensin II infusion - have underscored the complexity of aging-associated atrial myopathy. While all three models demonstrated left atrial mechanical dysfunction, there was a notable discrepancy in atrial fibrillation inducibility: aged mice did not exhibit the increased susceptibility to AF seen in the HFpEF and angiotensin II infusion groups[48]. This unexpected finding suggests that the mechanisms linking aging to atrial myopathy may differ fundamentally from those driving other forms of the disease. Further research is needed to elucidate the underlying factors contributing to this discrepancy, potentially involving deeper investigation into age-specific molecular pathways and alterations in atrial tissue composition. Additionally, the aging population is highly heterozygous, with significant variations in comorbidities, genetic predispositions, lifestyle factors, and overall health status. This diversity complicates the generalization of research findings and the development of standardized treatment protocols, necessitating a personalized medicine approach, which is still in its nascent stages.
FUTURE PERSPECTIVES
Novel biomarkers
Looking ahead, the identification and validation of biomarkers for atrial myopathy may hold potential for advancing both research and clinical practice. Several biomarkers associated with atrial myopathy have already been identified, but their clinical utility may be limited due to issues of specificity. For example, while elevated levels of troponin I and NT-proBNP have been linked to decreased left atrial reservoir and contractile strain in HFpEF patients, these biomarkers lack specificity because they also indicate other cardiovascular conditions, such as myocardial infarction and HF[123,161,162]. Thus, future efforts should focus on discovering more specific biomarkers that can accurately reflect left atrial dysfunction. Despite these challenges, biomarkers may offer a more precise and non-invasive way to identify atrial myopathy, especially in its early stages, when structural and functional changes might not yet be detectable through conventional imaging techniques. With the validation of such biomarkers, clinicians could identify patients at risk of developing atrial myopathy before significant dysfunction occurs. Furthermore, validated biomarkers would be invaluable for stratifying patients for clinical trials and could serve as surrogate endpoints in studies, potentially accelerating the development of new therapeutic agents.
Therapeutic interventions
Developing effective therapeutic interventions for atrial myopathy poses a significant challenge. Although atrial myopathy is a distinct clinical entity, it often develops secondary to conditions such as hypertension, HFpEF, and mitral valve disease[17,163,164]. Early identification and management of these underlying disorders may help slow their progression and mitigate structural and functional changes in the left atrium. However, in the cases when atrial myopathy occurs as a primary disorder, most therapeutic approaches focus on mitigating the downstream consequences of atrial myopathy - such as AF, HF, and thromboembolic events - rather than addressing the underlying atrial pathology.
Several therapies are under investigation for their potential to protect the left atrium from adverse remodeling. For instance, results of the RACE-3 trial indicated that mineralocorticoid receptor antagonists, such as spironolactone, may promote the maintenance of sinus rhythm in patients with AF and HF, suggesting a protective effect on the left atrium[165,166]. This effect might be attributed to the antifibrotic properties of spironolactone, which could reduce further pathological atrial remodeling[167]. However, it is important to note that spironolactone is not specific to the left atrium and its effects are not limited to this region. Moreover, despite the promising results of the RACE-3 trial, the IMPRESS-AF trial later demonstrated that spironolactone did not improve exercise capacity, E/e’ ratio, or quality of life in patients with chronic AF, but results may have been driven by the fact that AF burden was very high in the trial population[168]. Meanwhile, devices aimed at reducing left atrial pressure are also under exploration. For example, the placement of a transcatheter interatrial shunt device has shown promise by reducing pulmonary capillary wedge pressure during exercise in patients with HF and an ejection fraction above 40%[169]. Similar interventions, such as a shunt device between the left atrium and coronary sinus, are being developed to alleviate the pressure burden on the left atrium[170]. Nonetheless, additional research is necessary to determine whether these devices will lead to improved outcomes for patients with atrial myopathy. Another promising treatment for atrial myopathy targets the underlying causes of impaired atrial function: danicamtiv, a cardiac myosin activator, has been shown to increase ATPase activity and calcium sensitivity in left atrial muscle fibers and improve left atrial function in patients with HF with reduced ejection fraction[171].
Given the accumulation of senescent cells with aging and their contribution to cardiovascular disease, senolytic drugs - which selectively eliminate these cells - hold promise as a therapeutic strategy for mitigating age-related atrial myopathy, though further research is needed to determine their efficacy and safety[172]. Finally, several genetic causes of atrial myopathy have been identified, paving the way for future gene-modifying therapies aimed at correcting these underlying genetic defects[173,174].
Antithrombotic therapy in atrial myopathy
Anticoagulation in patients with atrial myopathy, particularly those with embolic stroke of undetermined source, remains a contentious topic[175]. The recent ARCADIA trial evaluated the efficacy of apixaban compared to aspirin for secondary stroke prevention in this population, enrolling 1,015 participants with cryptogenic stroke and markers of atrial myopathy, such as P-wave terminal force on electrocardiography, elevated NT-proBNP levels, or increased left atrial size on echocardiography[176]. The trial was halted early for futility after a mean follow-up of 1.8 years, demonstrating no significant difference in recurrent stroke rates between apixaban and aspirin groups (4.4% in both). Several factors might explain the negative outcome of the ARCADIA trial. First, a longer follow-up period with more events might have revealed statistically significant differences between the two groups[177]. Second, many participants had hypertension, diabetes, and tobacco use, which increased their baseline risk for atherosclerotic cardiovascular disease, suggesting that recurrent strokes may have been atherosclerotic rather than cardioembolic. Third, the delayed enrollment after the qualifying stroke, along with the mild stroke severity at baseline, may have led to the omission of high-risk patients. Fourth, approximately 46% of participants were enrolled based on NT-proBNP levels alone, a biomarker that may not be sufficiently specific for atrial myopathy[178]. Additionally, the possible inclusion of patients with patent foramen ovale, for whom no data were presented, could have influenced the results, because patent foramen ovale-related atrial dysfunction may promote in situ thrombosis and not qualify as cryptogenic stroke, potentially affecting the study’s applicability and interpretation[179]. Although the ARCADIA trial results were null, it does not mean that we should abandon the idea of anticoagulation in patients with atrial myopathy; instead, the findings provide valuable insights for refining future research in this area.
Primary prevention of atrial myopathy
Many cardiovascular diseases, such as coronary artery disease, have efficacious preventative therapies in addition to secondary therapeutics. Compared to treating atrial myopathy, preventing the onset of atrial myopathy in the aging population also presents a complex challenge due to the multifactorial nature of the disease. Unlike coronary artery disease, which is driven by atherosclerosis and amenable to targeted interventions like statins or antiplatelet agents, the pathogenesis of atrial myopathy involves diverse mechanisms, including inflammation, oxidative stress, and increased atrial stretch[8]. These factors collectively promote atrial remodeling, fibrosis, and electrophysiological changes, all of which are difficult to modify. Thus, while primary prevention of atrial myopathy remains challenging, it underscores the need for more comprehensive and individualized strategies that target the underlying contributors to atrial disease.
SUMMARY
Aging is a primary driver of atrial myopathy, a condition that underlies AF, HFpEF, and thromboembolism. As the older population continues to expand, understanding the interplay between aging and atrial myopathy is increasingly important. This review has highlighted the structural, mechanical, electrophysiological, histological, and metabolic changes that occur in the left atrium with advancing age, emphasizing their role in the pathogenesis of atrial myopathy. The recognition of atrial myopathy as a distinct clinical entity shifts the focus from solely managing its symptomatic manifestations to addressing the underlying atrial pathology. This paradigm shift has significant implications for both the prevention and treatment of cardiovascular diseases in the aging population. Early detection and intervention strategies targeting subclinical atrial myopathy may help prevent progression to more severe outcomes.
Despite the growing evidence linking aging to atrial myopathy, gaps remain in understanding its underlying mechanisms and clinical implications. Advancing diagnostic tools, including imaging and biomarker discovery, will be crucial in identifying atrial myopathy at its earliest stages. Therapies targeting atrial myopathy itself, rather than its complications, hold promise for improving outcomes. Future research should focus on elucidating the molecular and cellular mechanisms driving age-related changes in the atria and exploring potential therapeutic targets.
In conclusion, the aging atrium presents both challenges and opportunities. By advancing our understanding of atrial myopathy and its relationship with aging, we can move toward a more comprehensive approach to cardiovascular disease prevention and management, ultimately improving the quality of life for our aging population.
DECLARATIONS
Acknowledgments
The lead author, Gyberg DJ, would like to express his deepest gratitude to his wife, Jada, for her unwavering support and encouragement throughout this research fellowship.
Authors’ contributions
Drafted: Gyberg DJ, Patel RB, Zhang MJ
Availability of data and materials
Not applicable.
Financial support and sponsorship
None.
Conflicts of interest
Gyberg DJ is a research fellow supported by the Sarnoff Cardiovascular Research Foundation, while the other authors have declared that they have no conflicts of interest.
Ethical approval and consent to participate
Not applicable.
Consent for publication
Not applicable.
Copyright
© The Author(s) 2025.
REFERENCES
1. World Health Organization. Global strategy and action plan on ageing and health. 2017. Available from: https://www.who.int/publications/i/item/global-strategy-and-action-plan-on-ageing-and-health [Last accessed on 18 Mar 2025].
2. Linz D, Gawalko M, Betz K, et al. Atrial fibrillation: epidemiology, screening and digital health. Lancet Reg Health Eur. 2024;37:100786.
3. Borlaug BA, Sharma K, Shah SJ, Ho JE. Heart failure with preserved ejection fraction: JACC scientific statement. J Am Coll Cardiol. 2023;81:1810-34.
4. Fauchier L, Bisson A, Bodin A. Heart failure with preserved ejection fraction and atrial fibrillation: recent advances and open questions. BMC Med. 2023;21:54.
5. Goette A, Kalman JM, Aguinaga L, et al. EHRA/HRS/APHRS/SOLAECE expert consensus on atrial cardiomyopathies: definition, characterization, and clinical implication. Heart Rhythm. 2017;14:e3-40.
6. Goldberger JJ, Arora R, Green D, et al. Evaluating the atrial myopathy underlying atrial fibrillation: identifying the arrhythmogenic and thrombogenic substrate. Circulation. 2015;132:278-91.
7. Omote K, Borlaug BA. Left atrial myopathy in heart failure with preserved ejection fraction. Circ J. 2023;87:1039-46.
9. Inciardi RM, Claggett B, Minamisawa M, et al. Association of left atrial structure and function with heart failure in older adults. J Am Coll Cardiol. 2022;79:1549-61.
10. Inciardi RM, Wang W, Alonso A, et al. Cardiac mechanics and the risk of atrial fibrillation in a community-based cohort of older adults. Eur Heart J Cardiovasc Imaging. 2024;25:1686-94.
11. Maheshwari A, Norby FL, Inciardi RM, et al. Left atrial mechanical dysfunction and the risk for ischemic stroke in people without prevalent atrial fibrillation or stroke: a prospective cohort study. Ann Intern Med. 2023;176:39-48.
12. Wang W, Zhang MJ, Inciardi RM, et al. Association of echocardiographic measures of left atrial function and size with incident dementia. JAMA. 2022;327:1138-48.
13. Lamberts RR, Lingam SJ, Wang HY, et al. Impaired relaxation despite upregulated calcium-handling protein atrial myocardium from type 2 diabetic patients with preserved ejection fraction. Cardiovasc Diabetol. 2014;13:72.
14. Watanabe M, Yokoshiki H, Mitsuyama H, Mizukami K, Ono T, Tsutsui H. Conduction and refractory disorders in the diabetic atrium. Am J Physiol Heart Circ Physiol. 2012;303:H86-95.
15. Kato T, Yamashita T, Sekiguchi A, et al. AGEs-RAGE system mediates atrial structural remodeling in the diabetic rat. J Cardiovasc Electrophysiol. 2008;19:415-20.
16. Goette A, Lendeckel U, Kuchenbecker A, et al. Cigarette smoking induces atrial fibrosis in humans via nicotine. Heart. 2007;93:1056-63.
17. Xu TY, Sun JP, Lee AP, et al. Left atrial function as assessed by speckle-tracking echocardiography in hypertension. Medicine. 2015;94:e526.
18. Disertori M, Quintarelli S, Grasso M, et al. Autosomal recessive atrial dilated cardiomyopathy with standstill evolution associated with mutation of Natriuretic Peptide Precursor A. Circ Cardiovasc Genet. 2013;6:27-36.
19. Peng W, Li M, Li H, et al. Dysfunction of myosin light-chain 4 (MYL4) leads to heritable atrial cardiomyopathy with electrical, contractile, and structural components: evidence from genetically-engineered rats. J Am Heart Assoc. 2017;6:e007030.
20. Zhu Y, Shi J, Zheng B, et al. Genetic findings in patients with primary fibrotic atrial cardiomyopathy. Eur J Med Genet. 2022;65:104429.
21. Vergaro G, Aimo A, Rapezzi C, et al. Atrial amyloidosis: mechanisms and clinical manifestations. Eur J Heart Fail. 2022;24:2019-28.
22. Nochioka K, Quarta CC, Claggett B, et al. Left atrial structure and function in cardiac amyloidosis. Eur Heart J Cardiovasc Imaging. 2017;18:1128-37.
23. Bandera F, Martone R, Chacko L, et al. Clinical importance of left atrial infiltration in cardiac transthyretin amyloidosis. JACC Cardiovasc Imaging. 2022;15:17-29.
24. Sanchis K, Cariou E, Colombat M, et al. Atrial fibrillation and subtype of atrial fibrillation in cardiac amyloidosis: clinical and echocardiographic features, impact on mortality. Amyloid. 2019;26:128-38.
25. Feng D, Syed IS, Martinez M, et al. Intracardiac thrombosis and anticoagulation therapy in cardiac amyloidosis. Circulation. 2009;119:2490-7.
26. Dubrey S, Pollak A, Skinner M, Falk RH. Atrial thrombi occurring during sinus rhythm in cardiac amyloidosis: evidence for atrial electromechanical dissociation. Br Heart J. 1995;74:541-4.
27. El-Am EA, Dispenzieri A, Melduni RM, et al. Direct current cardioversion of atrial arrhythmias in adults with cardiac amyloidosis. J Am Coll Cardiol. 2019;73:589-97.
28. Feng D, Edwards WD, Oh JK, et al. Intracardiac thrombosis and embolism in patients with cardiac amyloidosis. Circulation. 2007;116:2420-6.
29. Cappelli F, Tini G, Russo D, et al. Arterial thrombo-embolic events in cardiac amyloidosis: a look beyond atrial fibrillation. Amyloid. 2021;28:12-8.
30. Singh A, Carvalho Singulane C, Miyoshi T, et al. Normal values of left atrial size and function and the impact of age: results of the world alliance societies of echocardiography study. J Am Soc Echocardiogr. 2022;35:154-64.e3.
31. Medrano G, Hermosillo-Rodriguez J, Pham T, et al. Left atrial volume and pulmonary artery diameter are noninvasive measures of age-related diastolic dysfunction in mice. J Gerontol A Biol Sci Med Sci. 2016;71:1141-50.
32. Pan NH, Tsao HM, Chang NC, Chen YJ, Chen SA. Aging dilates atrium and pulmonary veins: implications for the genesis of atrial fibrillation. Chest. 2008;133:190-6.
33. Eshoo S, Ross DL, Thomas L. Impact of mild hypertension on left atrial size and function. Circ Cardiovasc Imaging. 2009;2:93-9.
34. Pritchett AM, Mahoney DW, Jacobsen SJ, Rodeheffer RJ, Karon BL, Redfield MM. Diastolic dysfunction and left atrial volume: a population-based study. J Am Coll Cardiol. 2005;45:87-92.
35. Lin KB, Chen KK, Li S, et al. Impaired left atrial performance resulting from age-related arial fibrillation is associated with increased fibrosis burden: insights from a clinical study combining with an in vivo experiment. Front Cardiovasc Med. 2020;7:615065.
37. Jansen HJ, Moghtadaei M, Rafferty SA, Rose RA. Atrial fibrillation in aging and frail mice: modulation by natriuretic peptide receptor C. Circ Arrhythm Electrophysiol. 2021;14:e010077.
38. Takahashi Y, Yamaguchi T, Otsubo T, et al. Histological validation of atrial structural remodelling in patients with atrial fibrillation. Eur Heart J. 2023;44:3339-53.
39. Clarke JD, Caldwell JL, Pearman CM, Eisner DA, Trafford AW, Dibb KM. Increased Ca buffering underpins remodelling of Ca2+ handling in old sheep atrial myocytes. J Physiol. 2017;595:6263-79.
40. Hayashi H, Wang C, Miyauchi Y, et al. Aging-related increase to inducible atrial fibrillation in the rat model. J Cardiovasc Electrophysiol. 2002;13:801-8.
41. Wu L, Emmens RW, van Wezenbeek J, et al. Atrial inflammation in different atrial fibrillation subtypes and its relation with clinical risk factors. Clin Res Cardiol. 2020;109:1271-81.
42. Tan R, Yuan M, Wang L, et al. The pathogenesis of aging-induced left atrial appendage thrombus formation and cardioembolic stroke in mice is influenced by inflammation-derived matrix metalloproteinases. Thromb Res. 2023;226:69-81.
43. Wehrum T, Lodemann T, Hagenlocher P, et al. Age-related changes of right atrial morphology and inflow pattern assessed using 4D flow cardiovascular magnetic resonance: results of a population-based study. J Cardiovasc Magn Reson. 2018;20:38.
44. Jansen HJ, Moghtadaei M, Mackasey M, et al. Atrial structure, function and arrhythmogenesis in aged and frail mice. Sci Rep. 2017;7:44336.
46. Sugimoto T, Robinet S, Dulgheru R, et al. Echocardiographic reference ranges for normal left atrial function parameters: results from the EACVI NORRE study. Eur Heart J Cardiovasc Imaging. 2018;19:630-8.
47. Nyberg J, Jakobsen EO, Østvik A, et al. Echocardiographic reference ranges of global longitudinal strain for all cardiac chambers using guideline-directed dedicated views. JACC Cardiovasc Imaging. 2023;16:1516-31.
48. Zhang MJ, Gyberg DJ, Healy CL, et al. Atrial myopathy quantified by speckle-tracking echocardiography in mice. Circ Cardiovasc Imaging. 2023;16:e015735.
49. Abou R, Leung M, Tonsbeek AM, et al. Effect of aging on left atrial compliance and electromechanical properties in subjects without structural heart disease. Am J Cardiol. 2017;120:140-7.
50. Liao JN, Chao TF, Kuo JY, et al. Age, sex, and blood pressure-related influences on reference values of left atrial deformation and mechanics from a large-scale Asian population. Circ Cardiovasc Imaging. 2017;10:e006077.
51. Boyd AC, Schiller NB, Leung D, Ross DL, Thomas L. Atrial dilation and altered function are mediated by age and diastolic function but not before the eighth decade. JACC Cardiovasc Imaging. 2011;4:234-42.
52. Chen LY, Ribeiro ALP, Platonov PG, et al. P wave parameters and indices: a critical appraisal of clinical utility, challenges, and future research-a consensus document endorsed by the international society of electrocardiology and the international society for holter and noninvasive electrocardiology. Circ Arrhythm Electrophysiol. 2022;15:e010435.
53. Eranti A, Aro AL, Kerola T, et al. Prevalence and prognostic significance of abnormal P terminal force in lead V1 of the ECG in the general population. Circ Arrhythm Electrophysiol. 2014;7:1116-21.
54. Wolder LD, Graff C, Baadsgaard KH, et al. Electrocardiographic P terminal force in lead V1, its components, and the association with stroke and atrial fibrillation or flutter. Heart Rhythm. 2023;20:354-62.
55. Turhan H, Yetkin E, Sahin O, et al. Comparison of P-wave duration and dispersion in patients aged > 65 years with those aged < 45 years. J Electrocardiol. 2003;36:321-6.
56. Magnani JW, Johnson VM, Sullivan LM, et al. P wave duration and risk of longitudinal atrial fibrillation in persons ≥ 60 years old (from the Framingham Heart Study). Am J Cardiol. 2011;107:917-21.e1.
57. Soliman EZ, Prineas RJ, Case LD, Zhang ZM, Goff DC Jr. Ethnic distribution of ECG predictors of atrial fibrillation and its impact on understanding the ethnic distribution of ischemic stroke in the Atherosclerosis Risk in Communities (ARIC) study. Stroke. 2009;40:1204-11.
58. Achmad C, Tiksnadi BB, Akbar MR, et al. Left volume atrial index and P-wave dispersion as predictors of postoperative atrial fibrillation after coronary artery bypass graft: a retrospective cohort study. Curr Probl Cardiol. 2023;48:101031.
59. Materazzo C, Piotti P, Mantovani C, Miceli R, Villani F. Atrial fibrillation after non-cardiac surgery: P-wave characteristics and Holter monitoring in risk assessment. Eur J Cardiothorac Surg. 2007;31:812-6.
60. van der Does WFB, Houck CA, Heida A, et al. Atrial electrophysiological characteristics of aging. J Cardiovasc Electrophysiol. 2021;32:903-12.
61. Kistler PM, Sanders P, Fynn SP, et al. Electrophysiologic and electroanatomic changes in the human atrium associated with age. J Am Coll Cardiol. 2004;44:109-16.
62. Sim I, Bishop M, O'Neill M, Williams SE. Left atrial voltage mapping: defining and targeting the atrial fibrillation substrate. J Interv Card Electrophysiol. 2019;56:213-27.
63. Ahmed-Jushuf F, Murgatroyd F, Dhillon P, Scott PA. The impact of the presence of left atrial low voltage areas on outcomes from pulmonary vein isolation. J Arrhythm. 2019;35:205-14.
64. Yamaguchi T, Otsubo T, Takahashi Y, et al. Atrial structural remodeling in patients with atrial fibrillation is a diffuse fibrotic process: evidence from high-density voltage mapping and atrial biopsy. J Am Heart Assoc. 2022;11:e024521.
65. Emelyanova L, Preston C, Gupta A, et al. Effect of aging on mitochondrial energetics in the human atria. J Gerontol A Biol Sci Med Sci. 2018;73:608-16.
66. Nemutlu E, Gupta A, Zhang S, et al. Decline of phosphotransfer and substrate supply metabolic circuits hinders ATP cycling in aging myocardium. PLoS One. 2015;10:e0136556.
67. Lee HC, Shiou YL, Jhuo SJ, et al. The sodium-glucose co-transporter 2 inhibitor empagliflozin attenuates cardiac fibrosis and improves ventricular hemodynamics in hypertensive heart failure rats. Cardiovasc Diabetol. 2019;18:45.
68. Franceschi C, Capri M, Monti D, et al. Inflammaging and anti-inflammaging: a systemic perspective on aging and longevity emerged from studies in humans. Mech Ageing Dev. 2007;128:92-105.
69. Thomas TP, Grisanti LA. The dynamic interplay between cardiac inflammation and fibrosis. Front Physiol. 2020;11:529075.
70. Mesquita TRR, Zhang R, de Couto G, et al. Mechanisms of atrial fibrillation in aged rats with heart failure with preserved ejection fraction. Heart Rhythm. 2020;17:1025-33.
71. Koh AS, Siau A, Gao F, et al. Left atrial phasic function in older adults is associated with fibrotic and low-grade inflammatory pathways. Gerontology. 2023;69:47-56.
72. Li D, Fareh S, Leung TK, Nattel S. Promotion of atrial fibrillation by heart failure in dogs: atrial remodeling of a different sort. Circulation. 1999;100:87-95.
73. Hopman LHGA, Mulder MJ, van der Laan AM, et al. Impaired left atrial reservoir and conduit strain in patients with atrial fibrillation and extensive left atrial fibrosis. J Cardiovasc Magn Reson. 2021;23:131.
74. Forsey RJ, Thompson JM, Ernerudh J, et al. Plasma cytokine profiles in elderly humans. Mech Ageing Dev. 2003;124:487-93.
75. Bonnema DD, Webb CS, Pennington WR, et al. Effects of age on plasma matrix metalloproteinases (MMPs) and tissue inhibitor of metalloproteinases (TIMPs). J Card Fail. 2007;13:530-40.
76. Polejaeva IA, Ranjan R, Davies CJ, et al. Increased susceptibility to atrial fibrillation secondary to atrial fibrosis in transgenic goats expressing transforming growth factor-β1. J Cardiovasc Electrophysiol. 2016;27:1220-9.
77. Guzadhur L, Pearcey SM, Duehmke RM, et al. Atrial arrhythmogenicity in aged Scn5a+/DeltaKPQ mice modeling long QT type 3 syndrome and its relationship to Na+ channel expression and cardiac conduction. Pflugers Arch. 2010;460:593-601.
78. Baba S, Dun W, Hirose M, Boyden PA. Sodium current function in adult and aged canine atrial cells. Am J Physiol Heart Circ Physiol. 2006;291:H756-61.
79. Dun W, Yagi T, Rosen MR, Boyden PA. Calcium and potassium currents in cells from adult and aged canine right atria. Cardiovasc Res. 2003;58:526-34.
80. Nagibin V, Egan Benova T, Viczenczova C, et al. Ageing related down-regulation of myocardial connexin-43 and up-regulation of MMP-2 may predict propensity to atrial fibrillation in experimental animals. Physiol Res. 2016;65 Suppl 1:S91-S100.
81. Di Micco R, Krizhanovsky V, Baker D, d'Adda di Fagagna F. Cellular senescence in ageing: from mechanisms to therapeutic opportunities. Nat Rev Mol Cell Biol. 2021;22:75-95.
83. Hu C, Zhang X, Teng T, Ma ZG, Tang QZ. Cellular senescence in cardiovascular diseases: a systematic review. Aging Dis. 2022;13:103-28.
84. Jesel L, Abbas M, Park SH, et al. Atrial fibrillation progression is associated with cell senescence burden as determined by p53 and p16 expression. J Clin Med. 2019;9:36.
85. Tang X, Li PH, Chen HZ. Cardiomyocyte senescence and cellular communications within myocardial microenvironments. Front Endocrinol. 2020;11:280.
86. Anderson R, Lagnado A, Maggiorani D, et al. Length-independent telomere damage drives post-mitotic cardiomyocyte senescence. EMBO J. 2019;38:e100492.
87. Gao XY, Lai YY, Luo XS, et al. Acetyltransferase p300 regulates atrial fibroblast senescence and age-related atrial fibrosis through p53/Smad3 axis. Aging Cell. 2023;22:e13743.
88. Hasan H, Park SH, Auger C, et al. Thrombin induces angiotensin II-mediated senescence in atrial endothelial cells: impact on pro-remodeling patterns. J Clin Med. 2019;8:1570.
89. Sen P, Shah PP, Nativio R, Berger SL. Epigenetic mechanisms of longevity and aging. Cell. 2016;166:822-39.
90. Herman AB, Occean JR, Sen P. Epigenetic dysregulation in cardiovascular aging and disease. J Cardiovasc Aging. 2021;1:10.
91. Roberts JD, Vittinghoff E, Lu AT, et al. Epigenetic age and the risk of incident atrial fibrillation. Circulation. 2021;144:1899-911.
92. Lin H, Yin X, Xie Z, et al. Methylome-wide association study of atrial fibrillation in Framingham heart study. Sci Rep. 2017;7:40377.
93. Doñate Puertas R, Meugnier E, Romestaing C, et al. Atrial fibrillation is associated with hypermethylation in human left atrium, and treatment with decitabine reduces atrial tachyarrhythmias in spontaneously hypertensive rats. Transl Res. 2017;184:57-67.e5.
94. Lugenbiel P, Govorov K, Syren P, et al. Epigenetic regulation of cardiac electrophysiology in atrial fibrillation: HDAC2 determines action potential duration and suppresses NRSF in cardiomyocytes. Basic Res Cardiol. 2021;116:13.
95. Song S, Zhang R, Mo B, et al. EZH2 as a novel therapeutic target for atrial fibrosis and atrial fibrillation. J Mol Cell Cardiol. 2019;135:119-33.
96. Jusic A, Thomas PB, Wettinger SB, et al. Noncoding RNAs in age-related cardiovascular diseases. Ageing Res Rev. 2022;77:101610.
97. Varghese LN, Schwenke DO, Katare R. Role of noncoding RNAs in cardiac ageing. Front Cardiovasc Med. 2023;10:1142575.
98. Jazbutyte V, Fiedler J, Kneitz S, et al. MicroRNA-22 increases senescence and activates cardiac fibroblasts in the aging heart. Age. 2013;35:747-62.
99. Boon RA, Iekushi K, Lechner S, et al. MicroRNA-34a regulates cardiac ageing and function. Nature. 2013;495:107-10.
100. Trembinski DJ, Bink DI, Theodorou K, et al. Aging-regulated anti-apoptotic long non-coding RNA Sarrah augments recovery from acute myocardial infarction. Nat Commun. 2020;11:2039.
101. Adam O, Löhfelm B, Thum T, et al. Role of miR-21 in the pathogenesis of atrial fibrosis. Basic Res Cardiol. 2012;107:278.
102. Lu Y, Zhang Y, Wang N, et al. MicroRNA-328 contributes to adverse electrical remodeling in atrial fibrillation. Circulation. 2010;122:2378-87.
103. Luo X, Pan Z, Shan H, et al. MicroRNA-26 governs profibrillatory inward-rectifier potassium current changes in atrial fibrillation. J Clin Invest. 2013;123:1939-51.
104. Benjamin EJ, Levy D, Vaziri SM, D'Agostino RB, Belanger AJ, Wolf PA. Independent risk factors for atrial fibrillation in a population-based cohort. The Framingham Heart Study. JAMA. 1994;271:840-4.
105. Vieira MJ, Teixeira R, Gonçalves L, Gersh BJ. Left atrial mechanics: echocardiographic assessment and clinical implications. J Am Soc Echocardiogr. 2014;27:463-78.
106. Lisi M, Mandoli GE, Cameli M, et al. Left atrial strain by speckle tracking predicts atrial fibrosis in patients undergoing heart transplantation. Eur Heart J Cardiovasc Imaging. 2022;23:829-35.
107. Patel DA, Lavie CJ, Milani RV, Shah S, Gilliland Y. Clinical implications of left atrial enlargement: a review. Ochsner J. 2009;9:191-6.
108. Kuppahally SS, Akoum N, Burgon NS, et al. Left atrial strain and strain rate in patients with paroxysmal and persistent atrial fibrillation: relationship to left atrial structural remodeling detected by delayed-enhancement MRI. Circ Cardiovasc Imaging. 2010;3:231-9.
109. Olsen FJ, Diederichsen SZ, Jørgensen PG, et al. Left atrial strain predicts subclinical atrial fibrillation detected by long-term continuous monitoring in elderly high-risk individuals. Circ Cardiovasc Imaging. 2024;17:e016197.
110. Mannina C, Ito K, Jin Z, et al. Association of left atrial strain with ischemic stroke risk in older adults. JAMA Cardiol. 2023;8:317-25.
111. Maffeis C, Rossi A, Cannata L, et al. Left atrial strain predicts exercise capacity in heart failure independently of left ventricular ejection fraction. ESC Heart Fail. 2022;9:842-52.
112. Jia F, Chen A, Zhang D, Fang L, Chen W. Prognostic value of left atrial strain in heart failure: a systematic review and meta-analysis. Front Cardiovasc Med. 2022;9:935103.
113. Donal E, Galli E, Schnell F. Left atrial strain: a must or a plus for routine clinical practice? Circ Cardiovasc Imaging. 2017;10:e007023.
114. Badano LP, Kolias TJ, Muraru D, et al. Standardization of left atrial, right ventricular, and right atrial deformation imaging using two-dimensional speckle tracking echocardiography: a consensus document of the EACVI/ASE/Industry Task Force to standardize deformation imaging. Eur Heart J Cardiovasc Imaging. 2018;19:591-600.
115. Pathan F, D'Elia N, Nolan MT, Marwick TH, Negishi K. Normal ranges of left atrial strain by speckle-tracking echocardiography: a systematic review and meta-analysis. J Am Soc Echocardiogr. 2017;30:59-70.e8.
116. Yuda S, Nakatani S, Isobe F, Kosakai Y, Miyatake K. Comparative efficacy of the maze procedure for restoration of atrial contraction in patients with and without giant left atrium associated with mitral valve disease. J Am Coll Cardiol. 1998;31:1097-102.
117. Vasan RS, Larson MG, Levy D, Galderisi M, Wolf PA, Benjamin EJ. National Heart; Lung; and Blood Institute; National Institutes of Health. Doppler transmitral flow indexes and risk of atrial fibrillation (the Framingham Heart Study). Am J Cardiol. 2003;91:1079-83.
118. Thomas L, Muraru D, Popescu BA, et al. Evaluation of left atrial size and function: relevance for clinical practice. J Am Soc Echocardiogr. 2020;33:934-52.
119. Khankirawatana B, Khankirawatana S, Peterson B, Mahrous H, Porter TR. Peak atrial systolic mitral annular velocity by Doppler tissue reliably predicts left atrial systolic function. J Am Soc Echocardiogr. 2004;17:353-60.
120. Siebermair J, Kholmovski EG, Marrouche N. Assessment of left atrial fibrosis by late gadolinium enhancement magnetic resonance imaging: methodology and clinical implications. JACC Clin Electrophysiol. 2017;3:791-802.
121. Whitaker J, Karády J, Karim R, et al. Standardised computed tomographic assessment of left atrial morphology and tissue thickness in humans. Int J Cardiol Heart Vasc. 2021;32:100694.
122. Romero J, Husain SA, Kelesidis I, Sanz J, Medina HM, Garcia MJ. Detection of left atrial appendage thrombus by cardiac computed tomography in patients with atrial fibrillation: a meta-analysis. Circ Cardiovasc Imaging. 2013;6:185-94.
123. Patel RB, Alenezi F, Sun JL, et al. Biomarker profile of left atrial myopathy in heart failure with preserved ejection fraction: insights from the RELAX trial. J Card Fail. 2020;26:270-5.
124. Guo J, Huang X, Dou L, et al. Aging and aging-related diseases: from molecular mechanisms to interventions and treatments. Signal Transduct Target Ther. 2022;7:391.
125. Barnett K, Mercer SW, Norbury M, Watt G, Wyke S, Guthrie B. Epidemiology of multimorbidity and implications for health care, research, and medical education: a cross-sectional study. Lancet. 2012;380:37-43.
126. Rivner H, Mitrani RD, Goldberger JJ. Atrial myopathy underlying atrial fibrillation. Arrhythm Electrophysiol Rev. 2020;9:61-70.
127. Kallergis EM, Goudis CA, Vardas PE. Atrial fibrillation: a progressive atrial myopathy or a distinct disease? Int J Cardiol. 2014;171:126-33.
128. Kornej J, Börschel CS, Benjamin EJ, Schnabel RB. Epidemiology of Atrial fibrillation in the 21st century: novel methods and new insights. Circ Res. 2020;127:4-20.
129. Khurshid S, Ashburner JM, Ellinor PT, et al. Prevalence and incidence of atrial fibrillation among older primary care patients. JAMA Netw Open. 2023;6:e2255838.
130. Granada J, Uribe W, Chyou PH, et al. Incidence and predictors of atrial flutter in the general population. J Am Coll Cardiol. 2000;36:2242-6.
131. Waldo AL. Inter-relationships between atrial flutter and atrial fibrillation. Pacing Clin Electrophysiol. 2003;26:1583-96.
132. Wolf PA, Dawber TR, Thomas HE Jr, Kannel WB. Epidemiologic assessment of chronic atrial fibrillation and risk of stroke: the Framingham study. Neurology. 1978;28:973-7.
133. Odutayo A, Wong CX, Hsiao AJ, Hopewell S, Altman DG, Emdin CA. Atrial fibrillation and risks of cardiovascular disease, renal disease, and death: systematic review and meta-analysis. BMJ. 2016;354:i4482.
134. Benjamin EJ, Wolf PA, D'Agostino RB, Silbershatz H, Kannel WB, Levy D. Impact of atrial fibrillation on the risk of death: the Framingham Heart Study. Circulation. 1998;98:946-52.
135. Redfield MM, Borlaug BA. Heart failure with preserved ejection fraction: a review. JAMA. 2023;329:827-38.
136. Melenovsky V, Hwang SJ, Redfield MM, Zakeri R, Lin G, Borlaug BA. Left atrial remodeling and function in advanced heart failure with preserved or reduced ejection fraction. Circ Heart Fail. 2015;8:295-303.
137. Patel RB, Lam CSP, Svedlund S, et al. Disproportionate left atrial myopathy in heart failure with preserved ejection fraction among participants of the PROMIS-HFpEF study. Sci Rep. 2021;11:4885.
138. Tamargo M, Obokata M, Reddy YNV, et al. Functional mitral regurgitation and left atrial myopathy in heart failure with preserved ejection fraction. Eur J Heart Fail. 2020;22:489-98.
139. Deedwania PC, Lardizabal JA. Atrial fibrillation in heart failure: a comprehensive review. Am J Med. 2010;123:198-204.
140. Smietana J, Plitt A, Halperin JL. Thromboembolism in the absence of atrial fibrillation. Am J Cardiol. 2019;124:303-11.
141. Brambatti M, Connolly SJ, Gold MR, et al. Temporal relationship between subclinical atrial fibrillation and embolic events. Circulation. 2014;129:2094-9.
142. Souza JB, Sousa MG, Laurinavicius AG, et al. Advanced echocardiography techniques (AETs) to assess left atrial structure and function in individuals with resistant hypertension. Echocardiography. 2023;40:792-801.
143. Vera A, Cecconi A, Ximénez-Carrillo Á, et al. Advanced echocardiography with left atrial strain and indexed left atrial three-dimensional volume for predicting underlying atrial fibrillation after cryptogenic stroke. Am J Cardiol. 2022;185:87-93.
144. Peters DC, Lamy J, Sinusas AJ, Baldassarre LA. Left atrial evaluation by cardiovascular magnetic resonance: sensitive and unique biomarkers. Eur Heart J Cardiovasc Imaging. 2021;23:14-30.
145. Pay L, Yumurtaş AÇ, Satti DI, et al. Arrhythmias beyond atrial fibrillation detection using smartwatches: a systematic review. Anatol J Cardiol. 2023;27:126-31.
146. Mannhart D, Lischer M, Knecht S, et al. Clinical validation of 5 direct-to-consumer wearable smart devices to detect atrial fibrillation: BASEL wearable study. JACC Clin Electrophysiol. 2023;9:232-42.
147. Verbrugge FH, Reddy YNV, Attia ZI, et al. Detection of left atrial myopathy using artificial intelligence-enabled electrocardiography. Circ Heart Fail. 2022;15:e008176.
148. Yuan N, Stein NR, Duffy G, et al. Deep learning evaluation of echocardiograms to identify occult atrial fibrillation. NPJ Digit Med. 2024;7:96.
149. Aquino GJ, Chamberlin J, Yacoub B, et al. Diagnostic accuracy and performance of artificial intelligence in measuring left atrial volumes and function on multiphasic CT in patients with atrial fibrillation. Eur Radiol. 2022;32:5256-64.
150. Pieszko K, Hiczkiewicz J, Łojewska K, et al. Artificial intelligence in detecting left atrial appendage thrombus by transthoracic echocardiography and clinical features: the Left Atrial Thrombus on Transoesophageal Echocardiography (LATTEE) registry. Eur Heart J. 2024;45:32-41.
151. Oakes RS, Badger TJ, Kholmovski EG, et al. Detection and quantification of left atrial structural remodeling with delayed-enhancement magnetic resonance imaging in patients with atrial fibrillation. Circulation. 2009;119:1758-67.
152. Marrouche NF, Wilber D, Hindricks G, et al. Association of atrial tissue fibrosis identified by delayed enhancement MRI and atrial fibrillation catheter ablation: the DECAAF study. JAMA. 2014;311:498-506.
153. Marrouche NF, Wazni O, McGann C, et al. Effect of MRI-guided fibrosis ablation vs conventional catheter ablation on atrial arrhythmia recurrence in patients with persistent atrial fibrillation: The DECAAF II randomized clinical trial. JAMA. 2022;327:2296-305.
154. Takemoto Y, Barnes ME, Seward JB, et al. Usefulness of left atrial volume in predicting first congestive heart failure in patients > 65 years of age with well-preserved left ventricular systolic function. Am J Cardiol. 2005;96:832-6.
155. Osranek M, Fatema K, Qaddoura F, et al. Left atrial volume predicts the risk of atrial fibrillation after cardiac surgery: a prospective study. J Am Coll Cardiol. 2006;48:779-86.
156. Beinart R, Boyko V, Schwammenthal E, et al. Long-term prognostic significance of left atrial volume in acute myocardial infarction. J Am Coll Cardiol. 2004;44:327-34.
157. Thadani SR, Shaw RE, Fang Q, Whooley MA, Schiller NB. Left atrial end-diastolic volume index as a predictor of cardiovascular outcomes: the heart and soul study. Circ Cardiovasc Imaging. 2020;13:e009746.
158. Shin SH, Claggett B, Inciardi RM, et al. Prognostic value of minimal left atrial volume in heart failure with preserved ejection fraction. J Am Heart Assoc. 2021;10:e019545.
159. Kreimer F, Gotzmann M. Left atrial cardiomyopathy - a challenging diagnosis. Front Cardiovasc Med. 2022;9:942385.
160. Olsen FJ, Bertelsen L, de Knegt MC, et al. Multimodality cardiac imaging for the assessment of left atrial function and the association with atrial arrhythmias. Circ Cardiovasc Imaging. 2016;9:e004947.
161. Babuin L. Troponin: the biomarker of choice for the detection of cardiac injury. Can Med Assoc J. 2005;173:1191-202.
162. Mueller C, McDonald K, de Boer RA, et al. Heart failure association of the european society of cardiology practical guidance on the use of natriuretic peptide concentrations. Eur J Heart Fail. 2019;21:715-31.
163. Kennedy JW, Yarnall SR, Murray JA, Figley MM. Quantitative angiocardiography. IV. Relationships of left atrial and ventricular pressure and volume in mitral valve disease. Circulation. 1970;41:817-24.
164. Zile MR, Gottdiener JS, Hetzel SJ, et al. Prevalence and significance of alterations in cardiac structure and function in patients with heart failure and a preserved ejection fraction. Circulation. 2011;124:2491-501.
165. Rienstra M, Hobbelt AH, Alings M, et al. Targeted therapy of underlying conditions improves sinus rhythm maintenance in patients with persistent atrial fibrillation: results of the RACE 3 trial. Eur Heart J. 2018;39:2987-96.
166. Patel RB, Shah SJ. Therapeutic targeting of left atrial myopathy in atrial fibrillation and heart failure with preserved ejection fraction. JAMA Cardiol. 2020;5:497-9.
167. Milliez P, Deangelis N, Rucker-Martin C, et al. Spironolactone reduces fibrosis of dilated atria during heart failure in rats with myocardial infarction. Eur Heart J. 2005;26:2193-9.
168. Shantsila E, Shahid F, Sun Y, et al. Spironolactone in atrial fibrillation with preserved cardiac fraction: the IMPRESS-AF trial. J Am Heart Assoc. 2020;9:e016239.
169. Feldman T, Mauri L, Kahwash R, et al. Transcatheter interatrial shunt device for the treatment of heart failure with preserved ejection fraction (REDUCE LAP-HF I [reduce elevated left atrial pressure in patients with heart failure]): a phase 2, randomized, sham-controlled trial. Circulation. 2018;137:364-75.
170. Simard T, Labinaz M, Zahr F, et al. Percutaneous atriotomy for levoatrial-to-coronary sinus shunting in symptomatic heart failure: first-in-human experience. JACC Cardiovasc Interv. 2020;13:1236-47.
171. Voors AA, Tamby JF, Cleland JG, et al. Effects of danicamtiv, a novel cardiac myosin activator, in heart failure with reduced ejection fraction: experimental data and clinical results from a phase 2a trial. Eur J Heart Fail. 2020;22:1649-58.
172. Kumar M, Yan P, Kuchel GA, Xu M. Cellular senescence as a targetable risk factor for cardiovascular diseases: therapeutic implications: JACC family series. JACC Basic Transl Sci. 2024;9:522-34.
173. Marcoux E, Sosnowski D, Ninni S, et al. Genetic atrial cardiomyopathies: common features, specific differences, and broader relevance to understanding atrial cardiomyopathy. Circ Arrhythm Electrophysiol. 2023;16:675-98.
174. Roselli C, Rienstra M, Ellinor PT. Genetics of atrial fibrillation in 2020: GWAS, genome sequencing, polygenic risk, and beyond. Circ Res. 2020;127:21-33.
175. Hart RG, Catanese L, Perera KS, Ntaios G, Connolly SJ. Embolic stroke of undetermined source: a systematic review and clinical update. Stroke. 2017;48:867-72.
176. Kamel H, Longstreth WT Jr, Tirschwell DL, et al. Apixaban to prevent recurrence after cryptogenic stroke in patients with atrial cardiopathy: the ARCADIA randomized clinical trial. JAMA. 2024;331:573-81.
177. Marcus GM, Ovbiagele B. Anticoagulation for atrial cardiopathy in cryptogenic stroke. JAMA. 2024;331:564-6.
178. Chi KY, Nanna M, Nanna MG. Apixaban to prevent recurrence after cryptogenic stroke. JAMA. 2024;331:1965-6.
Cite This Article

How to Cite
Download Citation
Export Citation File:
Type of Import
Tips on Downloading Citation
Citation Manager File Format
Type of Import
Direct Import: When the Direct Import option is selected (the default state), a dialogue box will give you the option to Save or Open the downloaded citation data. Choosing Open will either launch your citation manager or give you a choice of applications with which to use the metadata. The Save option saves the file locally for later use.
Indirect Import: When the Indirect Import option is selected, the metadata is displayed and may be copied and pasted as needed.
About This Article
Copyright
Data & Comments
Data
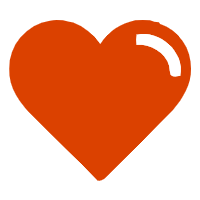
Comments
Comments must be written in English. Spam, offensive content, impersonation, and private information will not be permitted. If any comment is reported and identified as inappropriate content by OAE staff, the comment will be removed without notice. If you have any queries or need any help, please contact us at [email protected].