The role of exercise in bolstering cardiac resilience during aging
Abstract
Aging leads to structural and functional deterioration of the heart, reducing its capacity to withstand internal and external stressors and consequently increasing the risk of heart failure. Exercise is a potent modulator of cardiovascular and metabolic health, offering numerous physiological benefits that can persist throughout the aging process. Studies suggest that exercise can decelerate age-related cardiac deterioration and mitigate the risk of heart failure. In this review, we discuss recent advances in our understanding of exercise-mediated molecular and cellular adaptations that could serve as therapeutic targets for age-related cardiac remodeling and functional decline. We also explore how exercise-induced changes may enhance cardiac resilience with age, examine sex differences in cardiac aging and response to exercise, and highlight the value of murine exercise models as research tools for identifying novel therapeutic targets and strategies to combat heart failure.
Keywords
INTRODUCTION
While global life expectancy is predicted to rise by nearly five years by 2050, disability-free life expectancy is not advancing at the same rate, largely due to the increasing prevalence of chronic conditions such as cardiovascular disease, along with associated risk factors including hypertension, diabetes, obesity, and a sedentary lifestyle[1-3].
Data from the Baltimore Longitudinal Study of Aging show that, even in the absence of overt disease, older adults often have maladaptive structural changes in the myocardium and a decline in cardiac function[3,4]. These changes reduce cardiac reserves[5], increasing the heart’s susceptibility to major pathologies, including heart failure with preserved ejection fraction (HFpEF). HFpEF is a syndrome commonly observed in the elderly, often accompanied by multiple comorbidities, substantial morbidity and mortality (with a ~65% mortality rate within five years of hospitalization), and few effective therapies[3,6]. Addressing these challenges is increasingly urgent as the global senior population continues to grow.
Exercise, defined as a structured and repetitive form of physical activity[7], is a key intervention for preventing cardiovascular and metabolic diseases, promoting longevity, and enhancing quality of life[8-10]. For instance, brisk walking for 450 min per week can extend lifespan by 4.5 years compared to a sedentary lifestyle[11]. Furthermore, both higher volumes and intensities of physical activity are associated with reduced mortality risk[12]. Long-term (over 21 years) participation in vigorous exercise, such as running, is linked to decreased age-related disability, a lower risk of myocardial infarction (MI) and heart failure (HF), as well as an increased lifespan[13-15]. Importantly, recent studies suggest that short-term (8-20 weeks) exercise can decelerate age-related cardiac deterioration and delay the onset of HF[4,9,16,17].
Despite these well-documented benefits, many older adults struggle to engage in regular exercise or achieve the recommended intensity and duration necessary to optimize health outcomes[18]. Investigating the molecular and cellular mechanisms underlying exercise adaptation could provide novel treatment strategies to enhance cardiometabolic resilience, even for individuals unable to exercise. This review explores recently identified molecular contributors to age-related cardiac decline and discusses emerging concepts related to exercise-induced benefits. We also highlight potential protective mechanisms as valuable targets for developing strategies aimed at enhancing cardiac resilience and counteracting the negative effects of cardiac aging and the development of HF.
AGE-RELATED CHANGES IN CARDIAC STRUCTURE AND FUNCTION
The most prominent morphological changes in the aging heart include left ventricular (LV) hypertrophy and cardiac fibrosis[19,20]. While resting cardiac function is generally maintained in the healthy aged heart, these structural changes often lead to, or coincide with reduced cardiac compliance and a decline in systolic reserves during acute exercise[4,19,21,22] [Figure 1]. By the age of 80, diastolic function and early diastolic filling rates decrease by approximately 50%[23]. Maximal aerobic capacity also declines progressively, with a 3%-6% decrease per decade between ages 30-50, and more than 20% per decade after the age of 70[21,24]. This reduction is linked to alterations in either the delivery of oxygen to exercising muscles (cardiac function) or the efficiency of oxygen utilization by the muscles.
Figure 1. Age-related cellular, structural and functional maladaptation in the heart. Aging causes cardiomyocyte hypertrophy and senescence, leading to an accumulation of senescent cells that secrete pro-inflammatory factors known as the senescence-associated secretory phenotype (SASP). This triggers macrophage and granulocyte recruitment and myofibroblast activation in the heart. Mitochondrial dysfunction also occurs, reducing ATP production and increasing ROS, which contributes to endothelial cell dysfunction. These cellular impairments result in structural changes like pathological cardiac hypertrophy, increased myocardial stiffness, fibrosis, and vascular dysfunction, ultimately manifesting as diastolic dysfunction and diminished exercise tolerance.
Women have smaller LVs and higher ventricular stiffness compared to men, with these differences becoming more pronounced with age[3,25]. While men often experience a decline in cardiac contractility after the age of 50, women tend to have preserved contractility; however, diastolic dysfunction is a more common cause of HF in women[3,25,26]. Women also live approximately 5-7 years longer than men globally, are more sedentary during mid to late adulthood[27], and are more prone to multiple comorbidities and greater frailty, particularly in older age. Despite these factors, women demonstrate better survival rates in HF[28,29]. It remains unclear whether the mechanisms underlying exercise intolerance in women with HF differ from those in men, or whether hormonal differences may be playing a role. Investigating how sex differences influence cardiac aging and HF progression could provide valuable insights into mechanisms of heart disease and inform the development of treatment strategies.
AGING INFLUENCES CELLULAR COMPOSITION IN THE HEART
The heart’s structural integrity and function depend on complex interactions between diverse cell types that respond to internal and external stressors in maintaining cardiac homeostasis. With age, the heart’s limited capacity to regenerate cardiomyocytes diminishes[30-32]. Consequently, the loss of regenerative potential and compensatory interactions between cardiomyocytes and non-cardiomyocytes (e.g., fibroblasts and endothelial cells) play a central role in age-related cardiac dysfunction. These cellular interactions, along with cellular changes, such as the accumulation of senescent cells and chronic inflammation, contribute to pathological cardiac remodeling and functional decline. The following sections examine how aging and stress shape these complex cellular dynamics.
Cardiomyocytes
Cardiomyocytes constitute 30%-40% of the total cell population and 80% of the cellular volume in the healthy heart[33]. Aging leads to both an accumulation of senescent cardiomyocytes and a loss of functional cardiomyocytes[25,34] [Figure 1]. Although aging does not significantly alter the low rate of cardiomyocyte apoptosis in the normal human heart[35], oxidative stress, metabolic dysfunction, and chronic inflammation can induce replicative senescence in cardiomyocytes[34,36]. Accumulating senescent cardiomyocytes disrupt intracellular communication and promote both chronic inflammation and cell death[36], which contributes to cardiomyopathy, arrythmia, and HF[36-38]. Replicative senescence is driven by the cell cycle inhibitory tumor suppressor pathways p53-p21 (CDKN1A) and p16INK4A-retinoblastoma-associated protein[39]. Deletion of p16Ink4a in aged mice reduces cardiac hypertrophy and fibrosis, enhances cardiomyocyte proliferation, and extends lifespan[39-41], underscoring the role of senescent cells in age-related pathological remodeling.
Senescent cardiomyocytes may also adopt a pro-inflammatory phenotype by releasing senescence-associated secretory phenotype (SASP) factors [Figure 1], including interleukin-1α and interleukin-6
Vascular endothelial cells
Endothelial cells (EC), comprising 60% of non-cardiomyocytes in the heart[33], are the most abundant cell type and play a central role in cardiac homeostasis. The endothelium regulates vascular tone, angiogenesis, thrombosis, inflammation, and tissue homeostasis[49,50]. However, aging induces EC dysfunction, characterized by a shift toward a vasoconstrictive, pro-inflammatory, and pro-thrombotic phenotype[51]. EC dysfunction disrupts tissue homeostasis and is primarily driven by oxidative stress, with increased release of reactive oxygen species (ROS). While low levels of hydrogen peroxide can promote nitric oxide (NO) production, excessive ROS reduces NO bioavailability and impairs vasodilation[52]. High levels of ROS can also trigger inflammation, as evidenced by increased EC activation and increased EC cytokine expression via nuclear factor kappa-light-chain enhancer of B cells (NF-κB)[53]. Additionally, oxidative stress can impair mitochondrial function and increase mitophagy[54], although the effect on metabolic processes (e.g., glycolysis) remains conflicting[55].
A major consequence of these events is EC senescence[56,57], driven largely by damage to the ECs through oxidative stress. This is believed to be in part due to the unique anatomical location of the ECs at the blood-tissue interface, where they are continually exposed to circulating factors and harmful stimuli such as hypoxia, high glucose levels, inflammatory mediators, and oxidizing molecules, creating a feedback loop that promotes senescence[58]. While senotherapeutics may reduce age-related EC dysfunction, their effects are context-dependent and need careful consideration[56]. Interestingly, while EC dysfunction affects both sexes, men show EC deterioration earlier than women and post-menopausal women show an accelerated rate of EC decline. The mechanism underlying these sex differences remains unclear, particularly whether the differences are linked to a loss of protection, or acceleration of aging by undetermined factors. It is becoming increasingly accepted that hormone changes between sexes have the potential to confer protection against cellular aging; however, the role of hormones in EC decline, particularly estrogen therapy, is complex and still not fully understood[59,60].
Cardiac fibroblasts
Cardiac fibrosis is a major contributor to myocardial stiffness and dysfunction in the aging heart, although it is less severe than in HF[6,61,62]. Cardiac fibroblasts, which make up less than 20% of non-cardiomyocytes, increase in number with age[33,63]. Aging, inflammation, and cardiomyocyte hypertrophy activate fibroblasts to transform into myofibroblasts (α-smooth muscle actin expressing fibroblasts)[61,64] through signaling pathways involving TGF-β, angiotensin II, IL-6, and mechanical stress[65-67] [Figure 1].
TGF-β is a key mediator of ECM accumulation and fibrosis. For example, aged heterozygous TGF-β mice (TGF-β +/-) show reduced fibrosis (4% vs. 10%) and improved cardiac compliance[68]. In pressure-overload models, pharmacological TGF-β inhibition or Smad2/3 deletion decreased cardiac fibrosis and improved diastolic function[65,66]. Angiotensin II-AT1 receptor signaling also stimulates TGF-β expression and activates cardiac fibroblasts through endothelin-1, IL-6, and periostin signaling[62,69,70]. ACE inhibitors reduced both cardiac hypertrophy and lowered TGF-β1 levels, implicating TGF-β as a key mediator of angiotensin II effects[71,72]. While TGF-β inhibition has anti-fibrotic effects, it poses challenges due to its critical role in inflammation resolution and tissue integrity.
Activated myofibroblasts promote fibrosis by secreting integrins, collagen, fibronectin, laminin, and regulating ECM composition and stiffness through proteolytic enzymes, including matrix metalloproteinases (MMPs) and their inhibitors plasminogen activator inhibitor-1 (PAI-1) and tissue inhibitors of MMPs (TIMPs)[73]. In aged hearts, fibroblasts upregulate prolyl hydroxylases and lysyl oxidase, enzymes involved in cross-linking ECM proteins, further increasing LV stiffness[74,75]. Myofibroblasts also influence the cardiac microenvironment through paracrine signaling and secretion of insulin-like growth factor factor-1 (IGF-1) and pro-inflammatory SASPs, exacerbating endothelial-to-mesenchymal cell transition (EndMT) and recruiting CCR-2-dependent monocytes (CCR2+), which promote inflammation and senescence[76]. Targeting prolonged myofibroblast activation could reverse cardiac fibrosis, prevent myocardial stiffness, and mitigate HF progression with age.
Leukocytes in the aged heart
Aging is associated with “inflammaging”, a state of chronic low-grade systemic inflammation characterized by altered cytokine/chemokine production, clonal hematopoiesis, myeloid cell shifts, and mitochondrial dysfunction[77,78], which can disrupt cardiac homeostasis[79,80]. Chronic inflammation increases the risk of HF and impairs myocardial function, particularly in older adults[39].
The healthy adult heart contains various leukocytes, including macrophages, neutrophils, and lymphocytes[81], which maintain cardiac homeostasis through cell-to-cell communication with other cardiac cells. Macrophages, the most abundant immune cells in the heart, represent 7%-8% of non-cardiomyocytes in the healthy adult mouse heart, and include at least three distinct subsets: (1) CCR2- MHC II low; (2) CCR2- MHC II high; and (3) CCR2+ MHC II high[82]. The CCR2- macrophages are of embryonic origin and are maintained by local proliferation, while CCR2+ macrophages are replenished by the influx of circulating monocytes[83-85].
As the heart ages, there is a shift in immune cell composition, characterized by an increase in monocyte-derived cardiac macrophages (CCR2+) and granulocytes [Figure 1][86,87]. These macrophages secrete pro-inflammatory cytokines (TNF-α, IL-1β, and IL-6), MMPs, and growth factors (TGF-α and -β), playing critical roles in myeloid cell recruitment, regulation of cardiomyocyte senescence, and ECM turnover in the aging heart[86,88]. Additionally, an increase in CD8+ T-cells has been observed in the aged mouse heart, though the implications of this are not fully understood (reviewed in[80]).
MITOCHONDRIAL DYSFUNCTION IN THE AGED HEART
Cardiac function relies on the ability of cardiomyocytes to maintain energy balance under varying workloads, hormonal levels, nutritional states, and substrate availability[89,90]. While fatty acid oxidation (FAO) is the primary source of adenosine triphosphate (ATP) generation in the healthy heart, aging reduces metabolic flexibility, shifting energy production toward anaerobic glycolysis[91]. This metabolic shift contributes to diminished cardiometabolic reserves and exercise intolerance[92]. Aging also decreases electron transport chain (ETC) activity, reduces oxidative phosphorylation (OXPHOS) and ATP production, and increases ROS generation[93,94].
Studies in aged mice overexpressing mitochondria-specific antioxidant catalase (mCAT) have shown attenuated markers of cardiac aging, including less mitochondrial protein oxidation, cardiac hypertrophy and fibrosis, along with improved diastolic function and extended lifespan[95,96]. These effects were not observed in mice overexpressing peroxisomal catalase (pCAT) or nuclear catalase (nCAT)[96], emphasizing the critical role of site-specific ROS regulation (e.g., mitochondria). Mitochondria-targeted antioxidants MitoTEMPO and MitoQ improve mitochondrial respiration and ATP generation, leading to restored vascular and cardiac function in aged hearts[97-99]. The mitochondria-targeted peptide SS-31 (elamipretide), though not a direct antioxidant, protects mitochondrial cardiolipin from oxidation, improves ETC electron flow, reduces superoxide production, and reverses diastolic dysfunction in aged mice[100].
While these interventions highlight the potential of targeting mitochondrial function as a therapeutic strategy, recent studies have begun to investigate the distinct roles of mitochondrial subpopulations within cardiomyocytes. Specifically, interfibrillar mitochondria (IFM), subsarcolemmal mitochondria (SSM), and perinuclear mitochondria (PNM) each have unique functional roles and metabolic responses[101-104]. IFM primarily supply ATP for sarcomere contraction due to their higher substrate oxidation rates and enzyme activity compared to SSM and PNM[101]. SSM regulate metabolite transport and protein synthesis, while PNM are located near the nuclei and are linked with nuclear functions[103,105,106]. In aged rodents, IFM show reduced ETC complex III and IV activity and decreased OXPHOS capacity, while SSM remain unaffected[104,107,108]. While targeting mitochondrial ROS shows promise in preclinical models of HF[109], translating these findings into clinical practice remains challenging. Understanding the roles of mitochondrial subpopulations could, therefore, enable the development of targeted therapies for mitochondrial dysfunction and open new avenues for age-related cardiac therapeutics.
CARDIAC ADAPTATION TO EXERCISE DURING AGING
Most research demonstrating the cardiac benefits of exercise has been conducted in young populations. However, substantial evidence suggests that the aging heart remains responsive to exercise, which can prevent and mitigate cardiovascular dysfunction[14,15,17,110]. While most forms of exercise induce molecular, structural, and functional adaptations that protect the heart from pathological stress, aerobic exercise is particularly effective in reducing cardiovascular morbidity and mortality[14,15,111]. This section reviews the physiological and molecular adaptations induced by short- and long-term aerobic exercise, with a focus on cardiac aging.
Effect of exercise on pathophysiological changes associated with aging
Short-term aerobic exercise activates signaling pathways that enhance cardiac contractility and increase heart rate and cardiac output (CO), leading to cardiac volume overload. This adaptive response promotes physiological cardiac growth, distinct from pathological hypertrophy in molecular mechanisms, cardiac phenotype, and clinical prognosis[112,113].
Exercise has been shown to partially reverse pathological cardiac remodeling and improve cardiac function in the elderly. For example, performing 30 min of exercise 4-5 days per week throughout life prevented age-related cardiac changes, such as myocardial stiffness and reduced myocardial compliance[114]. Similarly, shorter duration of exercise (6-12 months) improved peak oxygen consumption and aerobic capacity in older adults (65-79 years), resulting in a 10% increase in LV mass while preserving the mass-to-volume ratio[115,116]. These benefits were also seen in middle-aged patients with early-stage HFpEF, characterized by LV hypertrophy and elevated cardiac biomarkers, where exercise significantly improved both myocardial stiffness and cardiac compliance after one year[15,114]. Furthermore, Jakovljevic et al. found that high levels of daily physical activity ( > 12,500 steps per day) preserved cardiac metabolism and aerobic capacity in healthy women across three age groups (young 20-30 years, middle-aged 40-50 years, and older 65-81 years)[117]. However, this activity had limited effects on age-related concentric remodeling, diastolic function, and overall cardiac performance in women[117], indicating potential sex differences in exercise adaptation. Accumulating evidence demonstrates that exercise significantly improves cardiac function[118], highlighting its potential as both a preventative and therapeutic strategy for HFpEF. However, a deeper understanding of the underlying mechanisms, as well as the optimal dose and intensity, is required to fully harness its benefits.
Exercise leads to cardiomyocyte growth and proliferation
Consistent with the reported beneficial effects in humans, we and others have demonstrated that 8 weeks of voluntary running mitigates diastolic dysfunction, the decline in contractile reserves, and reduced cardiomyogenesis in aged mice[17,110,119-122]. While the adult heart has a limited capacity to generate new cardiomyocytes, hypertrophy serves as the primary compensatory cardiomyocyte adaptation in response to increased workload. However, multiple studies show that exercise-induced cardiac growth involves both an increase in cardiomyocyte size and cardiomyocyte proliferation in young and old mice[110,113,120,123,124] [Figure 2]. Despite the lower absolute number of proliferating cardiomyocytes in aged hearts, the relative increase in cardiomyogenesis in exercised aged mice is comparable to that detected in young sedentary mice, suggesting that the aging heart retains a considerable capacity for regeneration in response to aerobic exercise. Mechanistically, exercise-induced cardiomyocyte proliferation is regulated by CCAAT/enhancer-binding protein β-CBP/p300-interacting transactivators with E (glutamic acid)/D (aspartic acid)-rich-carboxyl terminal domain (C/EBPβ-CITED4) axis and microRNA-222 signaling, which drive adaptive cardiac growth and enhance cardiomyocyte survival. These findings highlight the potential for reactivation of intrinsic cardiomyocyte proliferative pathways, even in aging hearts. This adaptive cardiomyogenic response holds therapeutic potential, as cardiomyocyte loss is an important contributor to age-induced cardiac remodeling and HF.
Figure 2. Exercise stimulates molecular and cellular changes in the aged heart. Exercise benefits cardiac cells and molecular processes. Exercise enhances endothelial cell function by increasing nitric oxide (NO) production and reducing reactive oxygen species (ROS), while also elevating vascular endothelial growth factor (VEGF) to support angiogenesis. In fibroblasts, exercise decreases myofibroblast activation, reducing fibrosis and improving cardiac function. It also impacts leukocytes by lowering hematopoietic stem and progenitor cell (HSPC) proliferation, reducing leukocytosis and increasing myeloid-derived suppressor cells (MDSCs) to diminish inflammation. Additionally, exercise promotes physiological cardiac growth through cardiomyocyte proliferation and hypertrophy, improving cardiac function.
Exercise can restore age-associated endothelial cell dysfunction
Short-term aerobic exercise (4-12 weeks) has been shown to mitigate the effects of EC aging, by restoring or even reversing age-related EC changes. For example, aerobic exercise increases intravascular blood flow and shear stress, stimulating NO production and restoring vasodilation or reversing impaired endothelial-dependent dilation (EDD) in previously sedentary individuals and in patients with HF[125,126]. Long-term exercise (more than five times per week for at least 10 years) not only reduces the impact of oxidative stress by increasing NO, but also enhances the expression of antioxidant enzymes such as superoxide dismutase (SOD), catalase, and glutathione S-transferases (GST)[127]. Furthermore, regular exercise reduces serum inflammatory markers; for example, two weeks of voluntary wheel activity in aged mice decreased activation of NF-κB and aortic expression of IL-1 and IL-6[128]. In the aging population, short-term exercise (30 min,
Short-term voluntary running (10-14 weeks) can also stimulate the mobilization of circulating endothelial progenitor cells (EPCs) and enhance their recovery after stress, by activating vascular endothelial growth factor (VEGF) and MMP9, thus promoting healthy vasculogenesis[130] [Figure 2 and 3]. Mitochondrial health is improved by aerobic activity, by reversing impaired mitophagy in senescent ECs (e.g., by elevating the mitochondrial clearance regulator FUNDC1[131-133]), enhancing mitochondrial biogenesis, and upregulating the mitochondrial antioxidant system (reviewed in[133]). Recent data have also revealed that endurance aerobic exercise can regulate microRNAs to benefit EC function (e.g., exercise reduces miR-155 to increase NO production and elevates miR-126 to enhance NO production and reduce both inflammation and ROS levels)[134]. While miRNA changes resulting from exercise can be protective against EC damage, the type and intensity of the exercise must be considered carefully, as some forms of strenuous exercise, such as marathon running, have been linked to elevated oxidative stress[135].
Figure 3. Molecular Pathways in Exercise-Induced Cardioprotection. Complex signaling pathways involved in exercise-induced cardioprotection, targeting age-related cardiac dysfunction and enhancing cardiac resilience. Key pathways include the activation of nuclear factor erythroid 2–related factor 2 (Nrf2), which upregulates metallothioneins (Mt1/2) to reduce ROS, alleviate oxidative stress, and prevent cardiac fibrosis. The β3-adrenergic receptor (β3-AR)/Protein kinase G (PKG) axis improves endothelial function and angiogenesis by increasing NO production through endothelial nitric oxide synthase (eNOS) during exercise, which activates cyclic guanosine monophosphate (cGMP), and PKG. Mechanical stress also boosts NO levels. AMP-activated protein kinase (AMPK) contributes to angiogenesis by increasing vascular endothelial growth factor (VEGF) expression, supporting cardiac performance. The Sirtuin 1 (SIRT1) and Peroxisome proliferator-activated receptor gamma co-activator 1-alpha (PGC1α) axis synergistically enhances mitochondrial function and supports energy metabolism, while PGC1α also interacts with the phosphoinositide 3-kinase (PI3K) pathway to promote mitochondrial biogenesis and improve cardiac function. The insulin-like growth factor 1 (IGF1)/phosphoinositide 3-kinase (PI3K)/serine/threonine kinase 1 (AKT)/mammalian target of rapamycin (mTOR) axis is crucial for cardiomyocyte survival, proliferation, and exercise-induced hypertrophy. Downregulation of CCAAT/enhancer-binding protein beta (C/EBPβ) and upregulation of CBP/p300-interacting transactivator with E/D-rich carboxy-terminal domain 4 (CITED4) promote physiological cardiac growth and cardiomyocyte proliferation via the mTOR pathway. Exercise also elevates several microRNAs (miRNAs) that regulate cardiac health: miR-29 reduces fibrosis, miR-126 enhances endothelial function, miR-342 supports cardiomyocyte proliferation and survival, miR-455 inhibits pathological remodeling, miR-222 promotes cardiomyocyte growth, and miR-17-3p facilitates adaptive cardiomyocyte growth in response to exercise.
Exercise inhibits the activation of fibroblasts and cardiac fibrosis
Recent evidence shows that daily swimming exercise for one to four weeks induces a distinct transcriptional profile in cardiac fibroblasts, reducing the expression of fibrotic markers, such as collagen genes (e.g., collagen type I alpha 1, Col1a1) and myofibroblast activation markers (e.g., periostin, Postn, and Acta2). Exercise activates nuclear factor erythroid 2-related factor (Nrf2) signaling in cardiac fibroblasts, which elevates the expression of antioxidant metallothioneins (Mts, including Mt1 and Mt2), enhancing resistance to oxidative stress and fibrotic remodeling[136]. Importantly, elevated Mts can also be transferred to cardiomyocytes, inhibiting apoptosis[136] and further promoting cardiac resilience.
In addition to Nrf2 activation, exercise-induced transcriptional co-activator CITED4 plays a key role in protecting against fibrosis. In CITED4 knockout mice, the absence of this protein resulted in cardiac dilation and increased fibrosis, under both short-term exercise intervention and pathological stress (such as pressure overload). CITED4 mediates its protective effects through mTOR signaling and the regulation of fibrosis-related microRNAs[137]. Similarly, four weeks of swimming (50 min daily, 6 days per week) activates adenosine monophosphate-activated protein kinase (AMPK) in an isoproterenol-induced mouse model, reducing fibrotic remodeling by inhibiting ROS production[138]. Likewise, short-term treadmill running activates the AMPK/SIRT1/PGC-1α pathway in aged rats, which alleviates cardiac fibrosis[138,139].
MicroRNAs, particularly miR-455 and miR-29, are identified as key mediators of exercise-induced physiological remodeling and cardioprotection. Increased miR-29 expression protects against pathological cardiac remodeling after myocardial infarction by inhibiting COL1A1 and collagen deposition[140]. Similarly, elevated miR-455 levels promote cardiomyocyte proliferation and reduce cardiac fibrosis by inhibiting MMP9[141] [Figure 3]. Both preclinical and clinical studies consistently demonstrate exercise-associated improvements in myocardial stiffening, cardiac compliance and fibrosis. Furthermore, cardiac fibroblasts contribute to physiological remodeling by producing exercise-induced growth factor IGF-1[142], highlighting the complexity of the system and the importance of balanced cross-talk between cardiac cell types in maintaining cardiac health.
Exercise can modulate immune responses
Immune cells are important regulators of the heart’s response to disease-induced stress and the progression of chronic HF[78,80,143,144]. Preclinical and clinical studies have linked exercise to reduced systemic and cardiac inflammation during aging and disease[122,145-148]. However, the cardiac immune response to exercise, especially in young vs. old heart, is unclear, and the roles of resident vs. recruited immune cells require further exploration.
Several studies suggest that exercise can reduce inflammation; however, this effect varies depending on sex differences and exercise intensity. For example, the MoTrPAC study revealed sex-specific changes in pro-inflammatory cytokines across tissues in young rats after eight weeks of endurance exercise[149]. In a different context, short-term exercise in aged diabetic mice lowered systemic cytokine and chemokine levels while increasing the expression of peroxisome proliferator-activated receptor gamma co-activator-1 alpha
Further evidence highlights how short-term exercise can modulate immune function. Frodermann et al. demonstrated that six weeks of voluntary running reduces inflammatory leukocyte production by lowering leptin levels in bone marrow-derived hematopoietic stem and progenitor cells (HSPCs)[152]. Mice lacking the leptin receptor had fewer leukocytes post-MI and improved cardiac function, highlighting leptin’s role in regulating HSPC activity and leukocyte production[152].
On the clinical side, studies have shown that short-term exercise can modulate inflammation through epigenetic regulation. For example, exercise induces methylation of the pro-inflammatory ASC gene (an adaptor molecule that mediates pro-inflammatory signaling), which decreases ASC expression and lowers pro-inflammatory cytokines such as IL-1β and IL-18[153-155]. In HF patients, increased ASC methylation correlates with less severe symptoms, improved cardiac function, and a reduced risk of adverse outcomes[153,154]. More findings on the effects of exercise on the murine immune system can be found in these excellent studies[149,156,157]. These findings underscore the importance of further investigating exercise-induced immune system changes, particularly with age.
MOLECULAR MECHANISM OF EXERCISE ADAPTATION
Molecular mediators driving cardiomyocyte adaptation to exercise in healthy animals underpin its protective effects in aging and HF. Preclinical rodent studies have identified key signaling pathways that mediate the heart’s adaptive response to exercise and confer protection against pathological stress[158]. Here, we focus on molecular pathways consistently linked to beneficial cardiac remodeling and cardioprotection.
Endurance training increases circulating IGF-1 levels[159], promoting physiological cardiac remodeling through the phosphoinositide 3-kinase/serine/threonine kinase 1 (PI3K-AKT) signaling cascade (IGF-1-PI3K-AKT)[159,160] [Figure 3]. This pathway enhances cardiomyocyte anti-apoptotic capacity and survival; however, IGF-1 activity must be tightly regulated, as prolonged expression can impair cardiac function[159,160]. Short-term swimming activates phosphoinositide 3-kinase (PI3K), particularly the p110α isoform, and increases antioxidant enzyme levels and heat shock proteins, promoting physiological hypertrophy and cardioprotection in mice[161,162]. Notably, activation of the AKT1 pathway enhances myocardial glucose uptake and cardiomyocyte survival[163,164], while PI3K overexpression induces physiological hypertrophy without adverse effects, suggesting its therapeutic potential in HF[161,162]. Conversely, mice with a dominant negative PI3K mutation show an impaired exercise-induced hypertrophic response and greater cardiac dysfunction under stress[161,162].
The C/EBPβ-CITED4 axis, a downstream target of the PI3K-AKT pathway, is a central regulator of exercise-induced cardiac growth and protection against pathological hypertrophy[165] [Figure 3]. In hearts from exercised mice, C/EBPβ downregulation promotes cardiomyogenesis, while preventing pathological changes by inhibiting NF-κB activation[120,165,166] [Figure 3]. This activates the mTORC1 pathway, which confers anti-apoptotic effects on the injured heart[124,165]. Importantly, C/EBPβ downregulation upregulates miR-222, miR-342, and miR-17 expression, which further enhances cardiomyocyte proliferation[123,124] [Figure 3]. Specifically, miR-222 induces physiological cardiomyocyte hypertrophy and proliferation by directly inhibiting Kip1/homeodomain-interacting protein kinase 1 (HIPK1/P27) and homeobox containing 1 (HMBOX1)[167], while elevated miR-17-3p promotes cardiomyocyte survival and proliferation by targeting TIMP3[168] [Figure 3]. Clinically, men with chronic heart failure (HFrEF and HFpEF) exhibit increased serum levels of miR-222 and miR17-3p following acute exercise (2.5-11 min on a bicycle ergometer), suggesting their potential as markers of exercise-induced cardiac adaptation[167,168].
In parallel, the β3-adrenergic receptor-nitric oxide (β3-Ars-NO) axis has emerged as a novel mechanism underlying exercise-induced cardiac remodeling. Animal studies show that eight weeks of voluntary running increases cardiac β3-Ars expression, which protects against ischemia-reperfusion injury by activating endothelial nitric oxide synthase (eNOS) and enhancing NO production[169]. NO subsequently activates soluble guanylate cyclase, elevating cyclic guanosine monophosphate (cGMP) and protein kinase G (PKG) signaling[170] [Figure 3]. While the precise molecular mechanisms of exercise-induced cardiac benefits are still being explored, findings from exercised mouse models provide critical insights into therapeutic strategies for combating age-related cardiac decline and heart failure progression.
EXERCISE IMPROVES MITOCHONDRIAL BIOGENESIS
One of the key adaptations of aerobic exercise in cardiomyocytes is the enhancement of metabolism and mitochondrial biogenesis. Exercise counteracts age-related metabolic changes by increasing mitochondrial numbers, enhancing antioxidant capacity, and reversing metabolic fuel shifts in the heart[92,171-174].
Central to these adaptations is the transcriptional co-activator PGC-1α, which regulates metabolic adaptation to exercise in the heart, promoting mitochondrial biogenesis, oxidative phosphorylation, and mitochondrial abundance[173]. PGC-1α exerts its effects by interacting with the estrogen-related receptors (ERRs), peroxisome proliferator-activated receptor (PPARs), and NRF-1/NRF-2, pathways that are regulated by sirtuin 1 (SIRT1)-mediated deacetylation and AMPK phosphorylation in response to nutrient availability or acute exercise[175-178]. For example, long-term exercise in a diabetic cardiomyopathy mouse model reduced ROS generation and mitigated cardiac metabolic impairments through activation of the PGC-1α-AKT signaling cascade[179]. Interestingly, older individuals show a reduced PGC-1α expression in skeletal muscle following long-term exercise, which may explain the smaller metabolic adaptations observed with aging[180].
Short-term exercise responses in skeletal muscle are primarily mediated by AMPK activation, with PPARδ contributing to longer-term transcriptional regulation that complements AMPKs immediate metabolic effects[181]. While selective PPARδ ligands are being tested as exercise mimetics for HF, AMPK activators pose potential risks, including glycogen accumulation, pathological cardiac hypertrophy, and conduction system abnormalities[182]. Interestingly, cardiometabolic therapies, such as Metformin and Empagliflozin (AMPK activators) or Resveratrol (SIRT1/PGC-1α/AMPK activators), target pathways modulated by exercise. Long-term administration of metformin at a therapeutic dose in healthy non-human primates has shown anti-aging effects at the molecular and tissue levels, including reduced SASPs and cardiac fibrosis, via activation of AMPK and Nrf2 antioxidant pathways[183]. This suggests that mimicking exercise-induced benefits could offer clinically relevant targets for treating age-related cardiac dysfunction.
CONCLUSION
This review highlights the complex interplay between cardiac aging and metabolic comorbidities, which obscure efforts to promote healthy cardiac aging. Key challenges include restoring cardiomyocyte turnover, improving vascular function, and alleviating oxidative stress and cardiac fibrosis. Although the molecular and cellular mechanisms of exercise in human cardiac aging are incompletely understood, evidence from preclinical models and clinical studies supports exercise as the most effective intervention for preventing and alleviating age-related cardiac dysfunction. Optimizing exercise duration and intensity, particularly for elderly populations, is essential to maximize its benefits, with consistent adherence to the recommended exercise regimens being equally critical for achieving meaningful outcomes.
The limited availability of human cardiac tissue is a major challenge for studying exercise-related mechanisms in cardiac aging. However, structural similarities between aging human and rodent hearts emphasize the value of small animal models as complementary tools to clinical studies. Addressing the fundamental mechanisms underlying the benefits of exercise could lead to innovative strategies for preventing, managing, and treating cardiometabolic diseases while also advancing our understanding of the aging process. Additionally, incorporating sex differences into studies of cardiac aging and exercise is essential for developing more effective, personalized therapies. Recent findings emphasize the therapeutic potential of exercise in mitigating multiple aspects of cardiac aging, offering promising avenues to improve cardiac health across diverse aging populations.
DECLARATIONS
Authors’ contributions
Wrote the manuscript: Saini A, Foote K, Vujic A
Prepared figures: Vujic A
Edited the manuscript: Foote K, Bennett M, Vujic A
All authors approved the final version of the manuscript.
Availability of data and materials
Not applicable.
Financial support and sponsorship
This work was supported by the British Heart Foundation Intermediate Fellowship award (RCZA/071) to Dr. Vujic A.
Conflicts of interest
All authors declared that there are no conflicts of interest.
Ethical approval and consent to participate
Not applicable.
Consent for publication
Not applicable.
Copyright
© The Author(s) 2025.
REFERENCES
1. GBD 2021 Forecasting Collaborators. Burden of disease scenarios for 204 countries and territories, 2022-2050: a forecasting analysis for the Global Burden of Disease Study 2021. Lancet. 2024;403:2204-56.
2. Ekelund U, Tarp J, Steene-Johannessen J, et al. Dose-response associations between accelerometry measured physical activity and sedentary time and all cause mortality: systematic review and harmonised meta-analysis. BMJ. 2019;366:l4570.
3. Martin SS, Aday AW, Almarzooq ZI, et al. 2024 heart disease and stroke statistics: a report of US and global data from the American heart association. Circulation. 2024;149:e347-913.
4. Fleg JL, O'Connor F, Gerstenblith G, et al. Impact of age on the cardiovascular response to dynamic upright exercise in healthy men and women. J Appl Physiol. 1995;78:890-900.
5. Hollingsworth KG, Blamire AM, Keavney BD, Macgowan GA. Left ventricular torsion, energetics, and diastolic function in normal human aging. Am J Physiol Heart Circ Physiol. 2012;302:H885-92.
6. Shah SJ, Borlaug BA, Kitzman DW, et al. Research priorities for heart failure with preserved ejection fraction: national heart, lung, and blood institute working group summary. Circulation. 2020;141:1001-26.
7. Caspersen CJ, Powell KE, Christenson GM. Physical activity, exercise, and physical fitness: definitions and distinctions for health-related research. Public Health Rep. 1985;100:126-31.
8. Valenzuela PL, Saco-Ledo G, Morales JS, et al. Effects of physical exercise on physical function in older adults in residential care: a systematic review and network meta-analysis of randomised controlled trials. Lancet Healthy Longev. 2023;4:e247-56.
9. Kitzman DW, Brubaker P, Morgan T, et al. Effect of caloric restriction or aerobic exercise training on peak oxygen consumption and quality of life in obese older patients with heart failure with preserved ejection fraction: a randomized clinical trial. JAMA. 2016;315:36-46.
10. Kraigher-Krainer E, Lyass A, Massaro JM, et al. Association of physical activity and heart failure with preserved vs. reduced ejection fraction in the elderly: the Framingham Heart Study. Eur J Heart Fail. 2013;15:742-6.
11. Moore SC, Patel AV, Matthews CE, et al. Leisure time physical activity of moderate to vigorous intensity and mortality: a large pooled cohort analysis. PLoS Med. 2012;9:e1001335.
12. Strain T, Wijndaele K, Dempsey PC, et al. Wearable-device-measured physical activity and future health risk. Nat Med. 2020;26:1385-91.
13. Chakravarty EF, Hubert HB, Lingala VB, Fries JF. Reduced disability and mortality among aging runners: a 21-year longitudinal study. Arch Intern Med. 2008;168:1638-46.
14. Howden EJ, Sarma S, Lawley JS, et al. Reversing the cardiac effects of sedentary aging in middle age-a randomized controlled trial: implications for heart failure prevention. Circulation. 2018;137:1549-60.
15. Hieda M, Sarma S, Hearon CM Jr, et al. One-year committed exercise training reverses abnormal left ventricular myocardial stiffness in patients with stage B heart failure with preserved ejection fraction. Circulation. 2021;144:934-46.
16. Safdar A, Annis S, Kraytsberg Y, et al. Amelioration of premature aging in mtDNA mutator mouse by exercise: the interplay of oxidative stress, PGC-1α, p53, and DNA damage. A hypothesis. Curr Opin Genet Dev. 2016;38:127-32.
17. Roh JD, Houstis N, Yu A, et al. Exercise training reverses cardiac aging phenotypes associated with heart failure with preserved ejection fraction in male mice. Aging Cell. 2020;19:e13159.
18. World Health Organization. WHO guidelines on physical activity and sedentary behaviour. 2020. Available from: https://www.who.int/publications/i/item/9789240015128 [Last accessed on 14 Jan 2025]
19. Cheng S, Xanthakis V, Sullivan LM, et al. Correlates of echocardiographic indices of cardiac remodeling over the adult life course: longitudinal observations from the Framingham Heart Study. Circulation. 2010;122:570-8.
20. Martos R, Baugh J, Ledwidge M, et al. Diastolic heart failure: evidence of increased myocardial collagen turnover linked to diastolic dysfunction. Circulation. 2007;115:888-95.
21. Fleg JL, Morrell CH, Bos AG, et al. Accelerated longitudinal decline of aerobic capacity in healthy older adults. Circulation. 2005;112:674-82.
22. Dannenberg AL, Levy D, Garrison RJ. Impact of age on echocardiographic left ventricular mass in a healthy population (the Framingham Study). Am J Cardiol. 1989;64:1066-8.
23. Schulman SP, Lakatta EG, Fleg JL, Lakatta L, Becker LC, Gerstenblith G. Age-related decline in left ventricular filling at rest and exercise. Am J Physiol. 1992;263:H1932-8.
24. Sui X, LaMonte MJ, Laditka JN, et al. Cardiorespiratory fitness and adiposity as mortality predictors in older adults. JAMA. 2007;298:2507-16.
25. Lakatta EG, Levy D. Arterial and cardiac aging: major shareholders in cardiovascular disease enterprises: Part II: the aging heart in health: links to heart disease. Circulation. 2003;107:346-54.
26. Chyou JY, Qin H, Butler J, Voors AA, Lam CSP. Sex-related similarities and differences in responses to heart failure therapies. Nat Rev Cardiol. 2024;21:498-516.
27. Strain T, Flaxman S, Guthold R, et al. National, regional, and global trends in insufficient physical activity among adults from 2000 to 2022: a pooled analysis of 507 population-based surveys with 5·7 million participants. Lancet Glob Health. 2024;12:e1232-43.
28. Denfeld QE, Habecker BA, Camacho SA, et al. Characterizing sex differences in physical frailty phenotypes in heart failure. Circ Heart Fail. 2021;14:e008076.
29. Dewan P, Rørth R, Jhund PS, et al. Differential impact of heart failure with reduced ejection fraction on men and women. J Am Coll Cardiol. 2019;73:29-40.
30. Bergmann O, Bhardwaj RD, Bernard S, et al. Evidence for cardiomyocyte renewal in humans. Science. 2009;324:98-102.
31. Bergmann O, Zdunek S, Felker A, et al. Dynamics of cell generation and turnover in the human heart. Cell. 2015;161:1566-75.
32. Senyo SE, Steinhauser ML, Pizzimenti CL, et al. Mammalian heart renewal by pre-existing cardiomyocytes. Nature. 2013;493:433-6.
33. Pinto AR, Ilinykh A, Ivey MJ, et al. Revisiting cardiac cellular composition. Circ Res. 2016;118:400-9.
34. López-Otín C, Blasco MA, Partridge L, Serrano M, Kroemer G. The hallmarks of aging. Cell. 2013;153:1194-217.
35. Mallat Z, Fornes P, Costagliola R, et al. Age and gender effects on cardiomyocyte apoptosis in the normal human heart. J Gerontol A Biol Sci Med Sci. 2001;56:M719-23.
36. Tang X, Li PH, Chen HZ. Cardiomyocyte senescence and cellular communications within myocardial microenvironments. Front Endocrinol. 2020;11:280.
37. Leri A, Franco S, Zacheo A, et al. Ablation of telomerase and telomere loss leads to cardiac dilatation and heart failure associated with p53 upregulation. EMBO J. 2003;22:131-9.
38. Chen MS, Lee RT, Garbern JC. Senescence mechanisms and targets in the heart. Cardiovasc Res. 2022;118:1173-87.
39. Baker DJ, Childs BG, Durik M, et al. Naturally occurring p16Ink4a-positive cells shorten healthy lifespan. Nature. 2016;530:184-9.
40. Lewis-McDougall FC, Ruchaya PJ, Domenjo-Vila E, et al. Aged-senescent cells contribute to impaired heart regeneration. Aging Cell. 2019;18:e12931.
41. Meyer K, Hodwin B, Ramanujam D, Engelhardt S, Sarikas A. Essential role for premature senescence of myofibroblasts in myocardial fibrosis. J Am Coll Cardiol. 2016;67:2018-28.
42. Tchkonia T, Zhu Y, van Deursen J, Campisi J, Kirkland JL. Cellular senescence and the senescent secretory phenotype: therapeutic opportunities. J Clin Invest. 2013;123:966-72.
43. Zhu Y, Tchkonia T, Pirtskhalava T, et al. The Achilles’ heel of senescent cells: from transcriptome to senolytic drugs. Aging Cell. 2015;14:644-58.
44. Wang M, Shah AM. Age-associated pro-inflammatory remodeling and functional phenotype in the heart and large arteries. J Mol Cell Cardiol. 2015;83:101-11.
45. Gwechenberger M, Mendoza LH, Youker KA, et al. Cardiac myocytes produce interleukin-6 in culture and in viable border zone of reperfused infarctions. Circulation. 1999;99:546-51.
46. Saucerman JJ, Tan PM, Buchholz KS, McCulloch AD, Omens JH. Mechanical regulation of gene expression in cardiac myocytes and fibroblasts. Nat Rev Cardiol. 2019;16:361-78.
47. Accornero F, van Berlo JH, Benard MJ, Lorenz JN, Carmeliet P, Molkentin JD. Placental growth factor regulates cardiac adaptation and hypertrophy through a paracrine mechanism. Circ Res. 2011;109:272-80.
48. Zhai P, Sadoshima J. Cardiomyocyte senescence and the potential therapeutic role of senolytics in the heart. J Cardiovasc Aging. 2024;4:18.
49. Donato AJ, Machin DR, Lesniewski LA. Mechanisms of dysfunction in the aging vasculature and role in age-related disease. Circ Res. 2018;123:825-48.
51. Ungvari Z, Tarantini S, Sorond F, Merkely B, Csiszar A. Mechanisms of vascular aging, a geroscience perspective: JACC focus seminar. J Am Coll Cardiol. 2020;75:931-41.
52. Hamilton CA, Brosnan MJ, McIntyre M, Graham D, Dominiczak AF. Superoxide excess in hypertension and aging: a common cause of endothelial dysfunction. Hypertension. 2001;37:529-34.
53. Csiszar A, Wang M, Lakatta EG, Ungvari Z. Inflammation and endothelial dysfunction during aging: role of NF-κB. J Appl Physiol. 2008;105:1333-41.
54. Gaetano A, Gibellini L, Zanini G, Nasi M, Cossarizza A, Pinti M. Mitophagy and oxidative stress: the role of aging. Antioxidants. 2021;10:794.
55. Unterluggauer H, Mazurek S, Lener B, et al. Premature senescence of human endothelial cells induced by inhibition of glutaminase. Biogerontology. 2008;9:247-59.
56. Han Y, Kim SY. Endothelial senescence in vascular diseases: current understanding and future opportunities in senotherapeutics. Exp Mol Med. 2023;55:1-12.
57. Ting KK, Coleman P, Zhao Y, Vadas MA, Gamble JR. The aging endothelium. Vasc Biol. 2021;3:R35-47.
58. Jia G, Aroor AR, Jia C, Sowers JR. Endothelial cell senescence in aging-related vascular dysfunction. Biochim Biophys Acta Mol Basis Dis. 2019;1865:1802-9.
59. Moreau KL, Babcock MC, Hildreth KL. Sex differences in vascular aging in response to testosterone. Biol Sex Differ. 2020;11:18.
60. Stanhewicz AE, Wenner MM, Stachenfeld NS. Sex differences in endothelial function important to vascular health and overall cardiovascular disease risk across the lifespan. Am J Physiol Heart Circ Physiol. 2018;315:H1569-88.
62. Frangogiannis NG. Cardiac fibrosis: cell biological mechanisms, molecular pathways and therapeutic opportunities. Mol Aspects Med. 2019;65:70-99.
63. Li Z, Duan Q, Cui Y, et al. Cardiac-specific expression of cre recombinase leads to age-related cardiac dysfunction associated with tumor-like growth of atrial cardiomyocyte and ventricular fibrosis and ferroptosis. Int J Mol Sci. 2023;24:3094.
64. Cieslik KA, Taffet GE, Carlson S, Hermosillo J, Trial J, Entman ML. Immune-inflammatory dysregulation modulates the incidence of progressive fibrosis and diastolic stiffness in the aging heart. J Mol Cell Cardiol. 2011;50:248-56.
65. Khalil H, Kanisicak O, Prasad V, et al. Fibroblast-specific TGF-β-Smad2/3 signaling underlies cardiac fibrosis. J Clin Invest. 2017;127:3770-83.
66. Kuwahara F, Kai H, Tokuda K, et al. Transforming growth factor-beta function blocking prevents myocardial fibrosis and diastolic dysfunction in pressure-overloaded rats. Circulation. 2002;106:130-5.
67. Meléndez GC, McLarty JL, Levick SP, Du Y, Janicki JS, Brower GL. Interleukin 6 mediates myocardial fibrosis, concentric hypertrophy, and diastolic dysfunction in rats. Hypertension. 2010;56:225-31.
68. Brooks WW, Conrad CH. Myocardial fibrosis in transforming growth factor β1 heterozygous mice. J Mol Cell Cardiol. 2000;32:187-95.
69. Li L, Fan D, Wang C, et al. Angiotensin II increases periostin expression via Ras/p38 MAPK/CREB and ERK1/2/TGF-β1 pathways in cardiac fibroblasts. Cardiovasc Res. 2011;91:80-9.
70. Gray MO, Long CS, Kalinyak JE, Li HT, Karliner JS. Angiotensin II stimulates cardiac myocyte hypertrophy via paracrine release of TGF-beta 1 and endothelin-1 from fibroblasts. Cardiovasc Res. 1998;40:352-63.
71. Basso N, Cini R, Pietrelli A, Ferder L, Terragno NA, Inserra F. Protective effect of long-term angiotensin II inhibition. Am J Physiol Heart Circ Physiol. 2007;293:H1351-8.
72. Benigni A, Corna D, Zoja C, et al. Disruption of the Ang II type 1 receptor promotes longevity in mice. J Clin Invest. 2009;119:524-30.
73. Ghosh AK, Bradham WS, Gleaves LA, et al. Genetic deficiency of plasminogen activator inhibitor-1 promotes cardiac fibrosis in aged mice: involvement of constitutive transforming growth factor-beta signaling and endothelial-to-mesenchymal transition. Circulation. 2010;122:1200-9.
74. Rohrbach S, Simm A, Pregla R, Franke C, Katschinski DM. Age-dependent increase of prolyl-4-hydroxylase domain (PHD) 3 expression in human and mouse heart. Biogerontology. 2005;6:165-71.
75. Stephens EH, Grande-Allen KJ. Age-related changes in collagen synthesis and turnover in porcine heart valves. J Heart Valve Dis. 2007;16:672-82.
76. Martini H, Iacovoni JS, Maggiorani D, et al. Aging induces cardiac mesenchymal stromal cell senescence and promotes endothelial cell fate of the CD90 + subset. Aging Cell. 2019;18:e13015.
77. Waterstrat A, Van Zant G. Effects of aging on hematopoietic stem and progenitor cells. Curr Opin Immunol. 2009;21:408-13.
78. Swirski FK. Inflammation and CVD in 2017: from clonal haematopoiesis to the CANTOS trial. Nat Rev Cardiol. 2018;15:79-80.
79. Shumliakivska M, Luxán G, Hemmerling I, et al. DNMT3A clonal hematopoiesis-driver mutations induce cardiac fibrosis by paracrine activation of fibroblasts. Nat Commun. 2024;15:606.
80. Swirski FK, Nahrendorf M. Cardioimmunology: the immune system in cardiac homeostasis and disease. Nat Rev Immunol. 2018;18:733-44.
81. Ramos GC, van den Berg A, Nunes-Silva V, et al. Myocardial aging as a T-cell-mediated phenomenon. Proc Natl Acad Sci USA. 2017;114:E2420-9.
82. Epelman S, Lavine KJ, Randolph GJ. Origin and functions of tissue macrophages. Immunity. 2014;41:21-35.
83. Epelman S, Lavine KJ, Beaudin AE, et al. Embryonic and adult-derived resident cardiac macrophages are maintained through distinct mechanisms at steady state and during inflammation. Immunity. 2014;40:91-104.
84. Heidt T, Courties G, Dutta P, et al. Differential contribution of monocytes to heart macrophages in steady-state and after myocardial infarction. Circ Res. 2014;115:284-95.
85. Bajpai G, Schneider C, Wong N, et al. The human heart contains distinct macrophage subsets with divergent origins and functions. Nat Med. 2018;24:1234-45.
86. Hulsmans M, Sager HB, Roh JD, et al. Cardiac macrophages promote diastolic dysfunction. J Exp Med. 2018;215:423-40.
87. Lim GB. Heart failure: macrophages promote cardiac fibrosis and diastolic dysfunction. Nat Rev Cardiol. 2018;15:196-7.
88. Wick G, Grundtman C, Mayerl C, et al. The immunology of fibrosis. Annu Rev Immunol. 2013;31:107-35.
89. Karwi QG, Uddin GM, Ho KL, Lopaschuk GD. Loss of metabolic flexibility in the failing heart. Front Cardiovasc Med. 2018;5:68.
90. Kates AM, Herrero P, Dence C, et al. Impact of aging on substrate metabolism by the human heart. J Am Coll Cardiol. 2003;41:293-9.
91. Nyberg M, Jones AM. Matching of O2 utilization and O2 delivery in contracting skeletal muscle in health, aging, and heart failure. Front Physiol. 2022;13:898395.
92. Lehman JJ, Kelly DP. Transcriptional activation of energy metabolic switches in the developing and hypertrophied heart. Clin Exp Pharmacol Physiol. 2002;29:339-45.
93. Lima T, Li TY, Mottis A, Auwerx J. Pleiotropic effects of mitochondria in aging. Nat Aging. 2022;2:199-213.
94. Picca A, Mankowski RT, Burman JL, et al. Mitochondrial quality control mechanisms as molecular targets in cardiac ageing. Nat Rev Cardiol. 2018;15:543-54.
95. Dai DF, Santana LF, Vermulst M, et al. Overexpression of catalase targeted to mitochondria attenuates murine cardiac aging. Circulation. 2009;119:2789-97.
96. Schriner SE, Linford NJ, Martin GM, et al. Extension of murine life span by overexpression of catalase targeted to mitochondria. Science. 2005;308:1909-11.
97. Owada T, Yamauchi H, Saitoh SI, Miura S, Machii H, Takeishi Y. Resolution of mitochondrial oxidant stress improves aged-cardiovascular performance. Coron Artery Dis. 2017;28:33-43.
98. Gioscia-Ryan RA, LaRocca TJ, Sindler AL, Zigler MC, Murphy MP, Seals DR. Mitochondria-targeted antioxidant (MitoQ) ameliorates age-related arterial endothelial dysfunction in mice. J Physiol. 2014;592:2549-61.
99. Junior RF, Dabkowski ER, Shekar KC, O Connell KA, Hecker PA, Murphy MP. MitoQ improves mitochondrial dysfunction in heart failure induced by pressure overload. Free Radic Biol Med. 2018;117:18-29.
100. Chiao YA, Zhang H, Sweetwyne M, et al. Late-life restoration of mitochondrial function reverses cardiac dysfunction in old mice. Elife. 2020;9:e55513.
101. Hollander JM, Thapa D, Shepherd DL. Physiological and structural differences in spatially distinct subpopulations of cardiac mitochondria: influence of cardiac pathologies. Am J Physiol Heart Circ Physiol. 2014;307:H1-14.
102. Hernandez-Resendiz S, Prakash A, Loo SJ, et al. Targeting mitochondrial shape: at the heart of cardioprotection. Basic Res Cardiol. 2023;118:49.
103. Hatano A, Okada J, Washio T, Hisada T, Sugiura S. Distinct functional roles of cardiac mitochondrial subpopulations revealed by a 3D simulation model. Biophys J. 2015;108:2732-9.
104. Hinton A Jr, Claypool SM, Neikirk K, et al. Mitochondrial structure and function in human heart failure. Circ Res. 2024;135:372-96.
105. Boengler K, Stahlhofen S, van de Sand A, et al. Presence of connexin 43 in subsarcolemmal, but not in interfibrillar cardiomyocyte mitochondria. Basic Res Cardiol. 2009;104:141-7.
106. Lu X, Thai PN, Lu S, Pu J, Bers DM. Intrafibrillar and perinuclear mitochondrial heterogeneity in adult cardiac myocytes. J Mol Cell Cardiol. 2019;136:72-84.
107. Tatarková Z, Kuka S, Račay P, et al. Effects of aging on activities of mitochondrial electron transport chain complexes and oxidative damage in rat heart. Physiol Res. 2011;60:281-9.
108. Paradies G, Ruggiero FM, Petrosillo G, Quagliariello E. Age-dependent decrease in the cytochrome c oxidase activity and changes in phospholipids in rat-heart mitochondria. Arch Gerontol Geriatr. 1993;16:263-72.
109. Vujic A, Koo ANM, Prag HA, Krieg T. Mitochondrial redox and TCA cycle metabolite signaling in the heart. Free Radic Biol Med. 2021;166:287-96.
110. Lerchenmüller C, Vujic A, Mittag S, et al. Restoration of cardiomyogenesis in aged mouse hearts by voluntary exercise. Circulation. 2022;146:412-26.
111. Lee DH, Rezende LFM, Joh HK, et al. Long-term leisure-time physical activity intensity and all-cause and cause-specific mortality: a prospective cohort of US adults. Circulation. 2022;146:523-34.
112. Beisvag V, Kemi OJ, Arbo I, et al. Pathological and physiological hypertrophies are regulated by distinct gene programs. Eur J Cardiovasc Prev Rehabil. 2009;16:690-7.
113. Seo DY, Kwak HB, Kim AH, et al. Cardiac adaptation to exercise training in health and disease. Pflugers Arch. 2020;472:155-68.
114. Bhella PS, Hastings JL, Fujimoto N, et al. Impact of lifelong exercise “dose” on left ventricular compliance and distensibility. J Am Coll Cardiol. 2014;64:1257-66.
115. Fujimoto N, Prasad A, Hastings JL, et al. Cardiovascular effects of 1 year of progressive and vigorous exercise training in previously sedentary individuals older than 65 years of age. Circulation. 2010;122:1797-805.
116. Woo JS, Derleth C, Stratton JR, Levy WC. The influence of age, gender, and training on exercise efficiency. J Am Coll Cardiol. 2006;47:1049-57.
117. Jakovljevic DG, Papakonstantinou L, Blamire AM, et al. Effect of physical activity on age-related changes in cardiac function and performance in women. Circ Cardiovasc Imaging. 2015;8:e002086.
118. Chesky JA, LaFollette S, Travis M, Fortado C. Effect of physical training on myocardial enzyme activities in aging rats. J Appl Physiol Respir Environ Exerc Physiol. 1983;55:1349-53.
119. Pei Z, Yang C, Guo Y, Dong M, Wang F. Effect of different exercise training intensities on age-related cardiac damage in male mice. Aging. 2021;13:21700-11.
120. Boström P, Mann N, Wu J, et al. C/EBPβ controls exercise-induced cardiac growth and protects against pathological cardiac remodeling. Cell. 2010;143:1072-83.
121. Kwak HB, Song W, Lawler JM. Exercise training attenuates age-induced elevation in Bax/Bcl-2 ratio, apoptosis, and remodeling in the rat heart. FASEB J. 2006;20:791-3.
122. Liao PH, Hsieh DJ, Kuo CH, et al. Moderate exercise training attenuates aging-induced cardiac inflammation, hypertrophy and fibrosis injuries of rat hearts. Oncotarget. 2015;6:35383-94.
123. Vujic A, Lerchenmüller C, Wu TD, et al. Exercise induces new cardiomyocyte generation in the adult mammalian heart. Nat Commun. 2018;9:1659.
124. Ding S, Gan T, Song M, et al. C/EBPB-CITED4 in exercised heart. Adv Exp Med Biol. 2017;1000:247-59.
125. Green DJ, Maiorana A, O’Driscoll G, Taylor R. Effect of exercise training on endothelium-derived nitric oxide function in humans. J Physiol. 2004;561:1-25.
126. Hambrecht R, Fiehn E, Weigl C, et al. Regular physical exercise corrects endothelial dysfunction and improves exercise capacity in patients with chronic heart failure. Circulation. 1998;98:2709-15.
127. Scarfò G, Daniele S, Chelucci E, et al. Regular exercise delays microvascular endothelial dysfunction by regulating antioxidant capacity and cellular metabolism. Sci Rep. 2023;13:17671.
128. Tao X, Chen Y, Zhen K, Ren S, Lv Y, Yu L. Effect of continuous aerobic exercise on endothelial function: a systematic review and meta-analysis of randomized controlled trials. Front Physiol. 2023;14:1043108.
129. Xia WH, Li J, Su C, et al. Physical exercise attenuates age-associated reduction in endothelium-reparative capacity of endothelial progenitor cells by increasing CXCR4/JAK-2 signaling in healthy men. Aging Cell. 2012;11:111-9.
130. Lesniewski LA, Durrant JR, Connell ML, et al. Aerobic exercise reverses arterial inflammation with aging in mice. Am J Physiol Heart Circ Physiol. 2011;301:H1025-32.
131. Kalka C, Masuda H, Takahashi T, et al. Vascular endothelial growth factor165 gene transfer augments circulating endothelial progenitor cells in human subjects. Circ Res. 2000;86:1198-202.
132. Ma L, Li K, Wei W, et al. Exercise protects aged mice against coronary endothelial senescence via FUNDC1-dependent mitophagy. Redox Biol. 2023;62:102693.
133. Gu Q, Wang B, Zhang XF, Ma YP, Liu JD, Wang XZ. Chronic aerobic exercise training attenuates aortic stiffening and endothelial dysfunction through preserving aortic mitochondrial function in aged rats. Exp Gerontol. 2014;56:37-44.
134. Meng Q, Su CH. The impact of physical exercise on oxidative and nitrosative stress: balancing the benefits and risks. Antioxidants. 2024;13:573.
135. Simioni C, Zauli G, Martelli AM, et al. Oxidative stress: role of physical exercise and antioxidant nutraceuticals in adulthood and aging. Oncotarget. 2018;9:17181-98.
136. Lighthouse JK, Burke RM, Velasquez LS, et al. Exercise promotes a cardioprotective gene program in resident cardiac fibroblasts. JCI Insight. 2019;4:e92098.
137. Lerchenmüller C, Rabolli CP, Yeri A, et al. CITED4 protects against adverse remodeling in response to physiological and pathological stress. Circ Res. 2020;127:631-46.
138. Ma X, Fu Y, Xiao H, et al. Cardiac fibrosis alleviated by exercise training is AMPK-dependent. PLoS One. 2015;10:e0129971.
139. Jia D, Hou L, Lv Y, Xi L, Tian Z. Postinfarction exercise training alleviates cardiac dysfunction and adverse remodeling via mitochondrial biogenesis and SIRT1/PGC-1α/PI3K/Akt signaling. J Cell Physiol. 2019;234:23705-18.
140. Dawson K, Wakili R, Ordög B, et al. MicroRNA29: a mechanistic contributor and potential biomarker in atrial fibrillation. Circulation. 2013;127:1466-75.
141. Chaturvedi P, Kalani A, Medina I, Familtseva A, Tyagi SC. Cardiosome mediated regulation of MMP9 in diabetic heart: role of mir29b and mir455 in exercise. J Cell Mol Med. 2015;19:2153-61.
142. Takeda N, Manabe I, Uchino Y, et al. Cardiac fibroblasts are essential for the adaptive response of the murine heart to pressure overload. J Clin Invest. 2010;120:254-65.
143. Epelman S, Liu PP, Mann DL. Role of innate and adaptive immune mechanisms in cardiac injury and repair. Nat Rev Immunol. 2015;15:117-29.
144. Leuschner F, Nahrendorf M. Novel functions of macrophages in the heart: insights into electrical conduction, stress, and diastolic dysfunction. Eur Heart J. 2020;41:989-94.
145. Yang HL, Hsieh PL, Hung CH, et al. Early moderate intensity aerobic exercise intervention prevents doxorubicin-caused cardiac dysfunction through inhibition of cardiac fibrosis and inflammation. Cancers. 2020;12:1102.
146. Xu X, Wan W, Powers AS, et al. Effects of exercise training on cardiac function and myocardial remodeling in post myocardial infarction rats. J Mol Cell Cardiol. 2008;44:114-22.
147. Noz MP, Hartman YAW, Hopman MTE, et al. Sixteen-week physical activity intervention in subjects with increased cardiometabolic risk shifts innate immune function towards a less proinflammatory state. J Am Heart Assoc. 2019;8:e013764.
148. Morici G, Zangla D, Santoro A, et al. Supramaximal exercise mobilizes hematopoietic progenitors and reticulocytes in athletes. Am J Physiol Regul Integr Comp Physiol. 2005;289:R1496-503.
149. Study Group, Lead Analysts, MoTrPAC Study Group. Temporal dynamics of the multi-omic response to endurance exercise training. Nature. 2024;629:174-83.
150. Botta A, Laher I, Beam J, et al. Short term exercise induces PGC-1α, ameliorates inflammation and increases mitochondrial membrane proteins but fails to increase respiratory enzymes in aging diabetic hearts. PLoS One. 2013;8:e70248.
151. Feng L, Li G, An J, et al. Exercise training protects against heart failure via expansion of myeloid-derived suppressor cells through regulating IL-10/STAT3/S100A9 pathway. Circ Heart Fail. 2022;15:e008550.
152. Frodermann V, Rohde D, Courties G, et al. Exercise reduces inflammatory cell production and cardiovascular inflammation via instruction of hematopoietic progenitor cells. Nat Med. 2019;25:1761-71.
153. Butts B, Butler J, Dunbar SB, Corwin E, Gary RA. Effects of exercise on ASC methylation and IL-1 cytokines in heart failure. Med Sci Sports Exerc. 2018;50:1757-66.
154. Butts B, Gary RA, Dunbar SB, Butler J. Methylation of apoptosis-associated speck-like protein with a caspase recruitment domain and outcomes in heart failure. J Card Fail. 2016;22:340-6.
155. Nakajima K, Takeoka M, Mori M, et al. Exercise effects on methylation of ASC gene. Int J Sports Med. 2010;31:671-5.
156. Duggal NA, Niemiro G, Harridge SDR, Simpson RJ, Lord JM. Can physical activity ameliorate immunosenescence and thereby reduce age-related multi-morbidity? Nat Rev Immunol. 2019;19:563-72.
157. Langston PK, Sun Y, Ryback BA, et al. Regulatory T cells shield muscle mitochondria from interferon-γ-mediated damage to promote the beneficial effects of exercise. Sci Immunol. 2023;8:eadi5377.
158. Vega RB, Konhilas JP, Kelly DP, Leinwand LA. Molecular mechanisms underlying cardiac adaptation to exercise. Cell Metab. 2017;25:1012-26.
159. Kim J, Wende AR, Sena S, et al. Insulin-like growth factor I receptor signaling is required for exercise-induced cardiac hypertrophy. Mol Endocrinol. 2008;22:2531-43.
160. Weeks KL, Bernardo BC, Ooi JYY, Patterson NL, Mcmullen JR. The IGF1-PI3K-akt signaling pathway in mediating exercise-induced cardiac hypertrophy and protection. Adv Exp Med Biol. 2017;1000:187-210.
161. McMullen JR, Shioi T, Zhang L, et al. Phosphoinositide 3-kinase(p110α) plays a critical role for the induction of physiological, but not pathological, cardiac hypertrophy. Proc Natl Acad Sci USA. 2003;100:12355-60.
162. Weeks KL, Gao X, Du XJ, et al. Phosphoinositide 3-kinase p110α is a master regulator of exercise-induced cardioprotection and PI3K gene therapy rescues cardiac dysfunction. Circ Heart Fail. 2012;5:523-34.
163. Matsui T, Tao J, del Monte F, et al. Akt activation preserves cardiac function and prevents injury after transient cardiac ischemia in vivo. Circulation. 2001;104:330-5.
164. DeBosch B, Treskov I, Lupu TS, et al. Akt1 is required for physiological cardiac growth. Circulation. 2006;113:2097-104.
165. Bezzerides VJ, Platt C, Lerchenmüller C, et al. CITED4 induces physiologic hypertrophy and promotes functional recovery after ischemic injury. JCI Insight. 2016;1:e85904.
166. Zou J, Li H, Chen X, et al. C/EBPβ knockdown protects cardiomyocytes from hypertrophy via inhibition of p65-NFκB. Mol Cell Endocrinol. 2014;390:18-25.
167. Liu X, Xiao J, Zhu H, et al. miR-222 is necessary for exercise-induced cardiac growth and protects against pathological cardiac remodeling. Cell Metab. 2015;21:584-95.
168. Shi J, Bei Y, Kong X, et al. miR-17-3p contributes to exercise-induced cardiac growth and protects against myocardial ischemia-reperfusion injury. Theranostics. 2017;7:664-76.
169. Calvert JW, Condit ME, Aragón JP, et al. Exercise protects against myocardial ischemia-reperfusion injury via stimulation of β3-adrenergic receptors and increased nitric oxide signaling: role of nitrite and nitrosothiols. Circ Res. 2011;108:1448-58.
170. Yang L, Jia Z, Yang L, et al. Exercise protects against chronic β-adrenergic remodeling of the heart by activation of endothelial nitric oxide synthase. PLoS One. 2014;9:e96892.
171. Fajardo G, Coronado M, Matthews M, Bernstein D. Mitochondrial quality control in the heart: the balance between physiological and pathological stress. Biomedicines. 2022;10:1375.
172. Ghahremani R, Damirchi A, Salehi I, Komaki A, Esposito F. Mitochondrial dynamics as an underlying mechanism involved in aerobic exercise training-induced cardioprotection against ischemia-reperfusion injury. Life Sci. 2018;213:102-8.
173. Lehman JJ, Barger PM, Kovacs A, Saffitz JE, Medeiros DM, Kelly DP. Peroxisome proliferator-activated receptor gamma coactivator-1 promotes cardiac mitochondrial biogenesis. J Clin Invest. 2000;106:847-56.
174. Vettor R, Valerio A, Ragni M, et al. Exercise training boosts eNOS-dependent mitochondrial biogenesis in mouse heart: role in adaptation of glucose metabolism. Am J Physiol Endocrinol Metab. 2014;306:E519-28.
175. Scarpulla RC, Vega RB, Kelly DP. Transcriptional integration of mitochondrial biogenesis. Trends Endocrinol Metab. 2012;23:459-66.
176. Musi N, Hirshman MF, Arad M, et al. Functional role of AMP-activated protein kinase in the heart during exercise. FEBS Lett. 2005;579:2045-50.
177. Timm KN, Tyler DJ. The role of AMPK activation for cardioprotection in doxorubicin-induced cardiotoxicity. Cardiovasc Drugs Ther. 2020;34:255-69.
178. Qian L, Zhu Y, Deng C, et al. Peroxisome proliferator-activated receptor gamma coactivator-1 (PGC-1) family in physiological and pathophysiological process and diseases. Signal Transduct Target Ther. 2024;9:50.
179. Judge S, Jang YM, Smith A, et al. Exercise by lifelong voluntary wheel running reduces subsarcolemmal and interfibrillar mitochondrial hydrogen peroxide production in the heart. Am J Physiol Regul Integr Comp Physiol. 2005;289:R1564-72.
180. Lanza IR, Short DK, Short KR, et al. Endurance exercise as a countermeasure for aging. Diabetes. 2008;57:2933-42.
181. Narkar VA, Downes M, Yu RT, et al. AMPK and PPARdelta agonists are exercise mimetics. Cell. 2008;134:405-15.
182. Kim TT, Dyck JR. Is AMPK the savior of the failing heart? Trends Endocrinol Metab. 2015;26:40-8.
Cite This Article
How to Cite
Download Citation
Export Citation File:
Type of Import
Tips on Downloading Citation
Citation Manager File Format
Type of Import
Direct Import: When the Direct Import option is selected (the default state), a dialogue box will give you the option to Save or Open the downloaded citation data. Choosing Open will either launch your citation manager or give you a choice of applications with which to use the metadata. The Save option saves the file locally for later use.
Indirect Import: When the Indirect Import option is selected, the metadata is displayed and may be copied and pasted as needed.
About This Article
Copyright
Data & Comments
Data
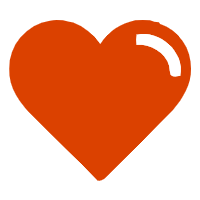
Comments
Comments must be written in English. Spam, offensive content, impersonation, and private information will not be permitted. If any comment is reported and identified as inappropriate content by OAE staff, the comment will be removed without notice. If you have any queries or need any help, please contact us at [email protected].