MXenes and their composites for advanced cathodes in multivalent ion batteries
Abstract
In recent years, multivalent metal-ion batteries (MMIBs) have garnered significant attention and research interest because of their abundant natural reserves, low cost, and high safety. However, in practical applications, owing to the high charge density of multivalent metal ions and the strong interaction between the intercalated metal ion and the cathode, the cathode exhibits low capacity and poor cycle stability. Therefore, it is crucial to explore suitable cathode materials for use in MMIBs. MXenes are novel two-dimensional materials that have developed rapidly in the field of energy storage. The current use of MXenes as cathodes in MMIBs has not yet been systematically summarized. This review summarizes the evolution and achievements of MXene-based cathodes in MMIBs, including MXenes and their derivatives, MXene/transition metal oxide composites, MXene/sulfur-based material composites, MXene/selenium-based material composites, and other MXene composites. Finally, the current challenges and future development of MXenes for advanced cathodes in MMIBs are discussed.
Keywords
INTRODUCTION
Large-scale development and utilization of fossil fuels have caused resource shortages and environmental pollution[1,2]. Traditional energy-storage technologies cannot meet the needs of social development[3]. Therefore, there is a global consensus on reducing dependence on traditional energy sources and developing clean and renewable energy resources[4]. Efficient storage of clean energy is crucial to ensure a reliable and sustainable energy supply[5]. Lithium-ion batteries (LIBs) are recognized as the most prevalent energy-storage devices owing to their exceptional energy density and cycling stability[6,7]. However, limited lithium resources, high costs, and unsafe and toxic organic electrolytes have triggered the search for more secure and stable energy-storage devices[8].
In recent years, multivalent metal-ion batteries (MMIBs) have undergone rapid development[9]. An outstanding feature of multivalent metals, such as zinc (Zn), aluminum (Al), magnesium (Mg), and calcium (Ca), is their ability to transfer multiple electrons during reactions[10]. Moreover, multivalent metals have many advantages, including abundant natural reserves, low cost, and high safety, making them a favorable choice for large-scale energy storage[11]. Therefore, researchers have shifted their focus to the investigation of MMIBs. However, several challenges remain associated with the use of MMIBs. Although the diameters of multivalent metal ions are similar to those of lithium ions, the high charge density of multivalent metal ions can generate strong Coulombic interactions with the crystal lattice of the cathode material, leading to high energy barriers that may induce adverse changes, such as irreversible phase transition, dissolution of active materials, and structural collapse of the cathode material[12,13]. Therefore, enhancing the conductivity of the cathode materials and expanding the space that can accommodate ions are crucial for optimizing the electrochemical performance of MMIBs.
MXenes, which are typical layered two-dimensional (2D) materials, have the advantages of outstanding electrical conductivity[14], high specific surface area[15], superior mechanical properties[16,17], excellent chemical stability[18], and strong adjustability[19]. To date, the applications of MXenes in advanced batteries have been extensively summarized and discussed. The utilization of MXenes and their derivatives in aqueous batteries has been comprehensively reviewed, including cathodes, anodes, and electrolytes[20-23]. In addition, the applications of MXenes in anodes have been carefully summarized, focusing on modification strategies employed by MXenes for stable and dendrite-free metal anodes[24,25]. With the rise of MMIBs, a review of the applications of MXenes in MMIBs involving the cathode and anode has been published[26]. However, to the best of our knowledge, a comprehensive review of MXenes as advanced cathodes for MMIBs has not yet been conducted. MXenes are primarily utilized as cathode materials in Zn-ion batteries (ZIBs), Al-ion batteries (AIBs), and Mg-ion batteries (MIBs). For Ca-ion batteries (CIBs), Ca2+ has a high coordination number (8), whereas MXenes exhibit a low-coordination environment[13]. Therefore, storing Ca2+ in MXenes is difficult. To date, no studies on MXenes in CIBs have been reported. It is an opportune moment to summarize the research progress and analyze the future directions of the application of MXenes as cathodes in MMIBs.
In this review, we summarize the evolution and accomplishments of MXenes as advanced cathodes for MMIBs. First, we provide an overview of the advantages and synthesis methods for MXenes. Subsequently, we discuss and summarize the applications of MXenes as cathodes in MMIBs, including MXenes and their derivatives, MXene/transition metal oxide composites, MXene/sulfur-based material composites, MXene/selenium-based material composites, and other MXene composites [Figure 1]. Finally, we offer insights into the development prospects of MXenes as cathodes for MMIBs.
MXENES
MXenes are typical layered 2D materials containing transition metal carbides, nitrides, and carbonitrides[27]. The general chemical formula is Mn+1XnTx (n = 1-4), where M denotes the early transition metal, such as titanium (Ti), vanadium (V), molybdenum (Mo), chromium (Cr), and zirconium (Zr), X represents carbon, nitrogen, or carbonitrides, and Tx denotes the surface functional group of the MXenes [Figure 2]. The functional group is typically represented by -OH, -O, -F, and -Cl[28,29]. MXenes and their derivatives have garnered considerable attention from researchers because of their unique structures, particularly in the field of electrochemical energy storage. Notably, the M elements in MXenes that are currently used as cathodes in MMIBs are Ti, V, and Nb. Therefore, it is necessary to explore other MXenes as cathodes for their potential application.
Advantages of MXenes
Physical properties of MXenes
An outstanding advantage of MXenes and their derivatives is their high electrical conductivity, which plays a vital role in enhancing ion and electron transfer. The Ti3C2Tx film was reported to exhibit an impressive electrical conductivity value of approximately 9,880 S cm-1 with a thin film thickness of 88 nm[14]. In addition, the 2D structure of MXenes has a high specific surface area and aspect ratio. This unique structure allows for better contact between the electrolyte and the electrode material, creating more active sites for electrochemical reactions. The nitrogen-doping Ti3C2Tx has been reported to have an open pore structure and a high specific surface area of up to 368.8 m2 g-1[15], while its MAX phase is only 5.1 m2 g-1.
Additionally, MXenes exhibit excellent strength and toughness. They can withstand high external loads and stresses without breaking or deforming. MXenes also exhibit remarkable hardness, which allows them to withstand scratches and abrasions caused by external forces. A 5-mm-thick hollow Ti3C2Tx cylinder can withstand 4,000 times its own weight[16]. Flexible Ti3C2Tx MXene paper has been reported to have a tensile strength of 83.2 MPa and a conductivity of 265,600 S m-1 and can be folded into small windmills[30]. These characteristics make MXenes an excellent electrode choice for flexible batteries and wearable electronics.
Chemical properties of MXenes
The remarkable chemical stability of MXenes makes them ideal for energy-storage devices, maintaining stability even in complex electrolyte environments[18]. This extends the lifespan of the devices and enhances their reliability and durability. Additionally, their negatively charged surface can be easily combined with other positively charged materials through an electrostatic self-assembly strategy[27], and the layered structure of MXenes enables electrodes to expose more active sites for charge storage, further enhancing their potential for energy-storage applications[31]. A heterostructure material (Cu-HHTP/MX) composed of Cu-HHTP (HHTP = 2,3,6,7,10,11-hexahydroxytriphenylene) and V2CTx MXene was prepared via electrostatic self-assembly[31]. Brunauer-Emmett-Teller (BET) results show that Cu-HHTP/MX has a larger surface area (152.6 m2 g-1) than Cu-HHTP (90.88 m2 g-1), suggesting that MXene can increase the number of active sites for the insertion/extraction of Zn2+ ions.
MXene materials can be tailored to meet specific performance requirements by adjusting their elemental compositions and terminal functional groups (Tx), such as -O, -OH, and -F. According to the reports[32,33], owing to fluorine-containing acid etching, the surface terminations of MXenes inevitably generate
Synthesis methods for MXenes
"Top-down" selective etching method
The “top-down” method removes the A-layer atoms in the MAX phase through direct or indirect chemical etching and then peels them to form single- or multilayer MXene nanosheets. Most MXenes are currently obtained using the “top-down” method, and the typical synthesis method is hydrofluoric acid (HF) etching. Ti3C2Tx was first synthesized using a HF solution to etch the Al layer of the Ti3AlC2 MAX phase, as shown in Figure 3A[35]. During the synthesis of MXenes, some crucial parameters exist, such as the HF concentration, etching time, and reaction temperature[36]. However, the strong corrosiveness and toxicity of HF render the preparation process dangerous. Other fluorine-based etchers have also been investigated. A mixture of hydrochloric acid (HCl) and lithium fluoride (LiF) as an alternative to HF was reported to synthesize
Figure 3. (A) Schematic illustration of the synthesis of Ti3C2Tx by HF. This figure is quoted with permission from Naguib et al. Copyright (2011) John Wiley and Sons[35]. (B) Schematic illustration of the preparation of Ti3C2Tx by HCl + LiF. This figure is quoted with permission from Ghidiu et al. Copyright (2014) Springer Nature[37]. (C) Schematic illustration of the preparation of Ti4N3Tx by high-temperature molten salt method. This figure is quoted with permission from Urbankowski et al. Copyright (2016) RSC Pub[42]. (D) Schematic illustration of the synthesis of Ti3C2Tx by organic polar solvents. (E) TEM image of delaminated Ti3C2Tx sheets etched by organic polar solvents. This figure is quoted with permission from Natu et al. Copyright (2020) Elsevier[43]. (F) Schematic illustration of the preparation of Ti3C2Tx by alkali etching. This figure is quoted with permission from Li et al. Copyright (2018) John Wiley and Sons[45]. (G) Schematic illustration of the synthesis of Ti3C2Tx by Lewis acidic molten melts etching. This figure is quoted with permission from Li et al. Copyright (2019) American Chemical Society[46]. (H) Schematic illustration of the preparation of Ti3C2Tx by halogen etching. This figure is quoted with permission from Jawaid et al. Copyright (2021) American Chemical Society[47].
Although the application of fluorine-based etchers (such as HCl + LiF, salt mixture (LiF + KF + NaF), and NH4HF2) reduces operational risk, it inevitably leads to environmental pollution. Consequently, researchers have redirected their focused on the synthesis of fluorine-free MXenes. Electrochemical etching has been demonstrated as an effective approach. Ti3C2Tx was obtained by anodic corrosion[44]. The Al atoms on the anode side of the MAX phase were extracted and subsequently replaced with hydroxide groups in the
"Bottom-up" direct synthesis method
In the “bottom-up” method, MXenes are directly synthesized without etching the MAX phase. Synthesized MXenes have the advantages of a large size and few defects[48]. Chemical vapor deposition (CVD) was the first direct synthesis method for MXenes. Mo2C MXene was synthesized by reacting Mo atoms from Mo foil with carbon atoms produced from methane at a temperature above 1,085 °C[49]. Mo2C is a chemically stable crystal with a size exceeding 100 µm and a thickness of several nanometers. In addition to conventional CVD systems, large-area Mo2C MXene has been grown using plasma-enhanced pulsed laser deposition[50]. This method combines the advantages of both plasma-enhanced CVD and pulsed laser deposition. The temperature required for this crystal growth is 700 °C, and the laser pulse number can control crystal thickness. In addition, MXenes can be directly synthesized using the salt-templated method. MoO3-coated NaCl was first synthesized using an Mo precursor and NaCl under an Ar atmosphere at 280 °C, and then MoO3-coated NaCl was ammoniated under an NH3 atmosphere at 650 °C to generate MoN MXene[51]. However, large-scale application of MXenes is hindered by the low output, high equipment requirements, and high cost of the “bottom-up” method[48]. Therefore, it is important to explore more efficient methods for expanding the applications of MXenes.
APPLICATION OF MXENES FOR CATHODE MATERIALS IN MMIBs
MXenes and their derivatives as cathodes
MXenes have developed rapidly in the field of energy storage. They have outstanding electrical conductivity[14], high specific surface area[15], superior mechanical properties[16,17], excellent chemical stability[18], and strong adjustability[19]. However, owing to the strong hydrogen bonds and interlayer van der Waals forces of MXenes, they tend to agglomerate and stack during cycling, leading to a reduction in active sites and acceleration of structural collapse [Figure 4][52]. Moreover, owing to the strong interactions between multivalent ions and MXenes, metal ions exhibit a high diffusion energy barrier during intercalation/deintercalation from MXenes. For example, pure Ti3C2 MXenes cannot store Mg ions and exhibit zero capacity in MIBs[53]. Therefore, to reduce the aggregation of MXenes and the migration barrier of ions, it is necessary to modify pure MXenes to improve their electrochemical performance.
MXenes and their derivatives as cathodes in ZIBs
ZIBs have attracted considerable interest because of their eco-friendliness, cost-effectiveness, and safety[54-57]. The Zn anode has a high theoretical specific capacity (820 mA h g-1 and 5,851 mA h cm-3), a low redox potential [-0.763 V vs. Standard Hydrogen Electrode (SHE)], and abundant reserves, making it an ideal large-scale energy-storage device[58,59]. In the past few years, 2D MXenes have been extensively researched in ZIBs because of their high metal conductivity (close to graphene: 105-106 S m-1), large layer spacing, structural stability, controllable surface functional groups, etc.
Although MXenes have many properties required for ZIB cathodes, the large amount of F surface terminations produced in the traditional fluoric acid etching process usually leads to high hydrophobicity and slow ion diffusion kinetics, which deteriorates the electrochemical performance. Therefore, the replacement of -F functional groups with functional groups that are hydrophilic (-OH) or electrochemically active (-I, -Br) is expected to improve the performance of MXenes. A novel OH-rich terminal V2CTx material with interlayer “K+-pillars” (alk-V2CTx) was proposed [Figure 5A][34]. According to the X-ray diffraction (XRD) patterns [Figure 5B], the (002) peak of alk-V2CTx shifted to a smaller angle, indicating that the inserted K+ ions increased the layer spacing[60]. Simultaneously, the inserted K+ ions improved the structural stability of the alk-V2CTx cathode. Owing to the large number of hydrophilic -OH terminations, the hydrophilicity of alk-V2CTx was significantly better than that of traditional HF-etched V2CTx [Figure 5C], which is conducive to electrolyte infiltration in the electrode. The alk-V2CTx cathode had a specific capacity of 498.2 mA h g-1 at 0.1 A g-1, with a capacity retention of 96.2% after 20,000 cycles at
Figure 5. (A) Illustration of hydrophilicity and structural stability’s improvement of alk-V2CTx. (B) XRD patterns of V2AlC, V2CTx, and alk-V2CTx. (C) Comparison of hydrophilicity between V2CTx and alk-V2CTx. This figure is quoted with permission from Chen et al. Copyright (2023) John Wiley and Sons[34]. (D) SEM image of Ti3C2Br2. (E) HAADF-STEM image and EDS mappings of Ti3C2Br2. (F) Charge and discharge curves of halogenated Ti3C2 MXenes at 0.5 A g-1. This figure is quoted with permission from Li et al. Copyright (2021) American Chemical Society[61]. (G) Schematic illustration of V2CTx cathodes at different voltages by in-situ electrochemical activation. (H and I) SEM images of V2CTx cathodes after electrochemical activation at 1.8 V and 2.0 V. This figure is quoted with permission from Liu et al. Copyright (2020) John Wiley and Sons[62].
In addition to -OH terminations, various halogen terminals (such as -Cl and -Br) can improve the electrochemical properties of MXene. Ti3C2 MXenes with different halogen terminals were obtained by etching with different copper halide molten salts at high temperatures[61]. After etching the Al layers, the scanning electron microscopy (SEM) image of Ti3C2Br2 [Figure 5D] exhibited a multilayer structure similar to that of Ti3C2(OF) MXene (obtained via traditional HF etching), indicating successful halogenated MXene etching. The high-angle annular dark-field scanning transmission electron microscopy (HAADF-STEM) image and energy-dispersive spectroscopy (EDS) elemental mapping [Figure 5E] showed that bromine (Br) was uniformly distributed on Ti3C2Br2. According to the galvanostatic charge and discharge (GCD) curves of Ti3C2 MXenes with different halogen terminals [Figure 5F], Ti3C2Br2 had a specific capacity of
Compared with other MXenes, V2C MXenes uniquely benefit from the multiple valence states of V and the high specific capacity of V-based cathodes. The oxidation of low-valence V in V2C to obtain MXene-based derivatives is a unique method for enhancing the electrochemical properties of V2C MXenes. The low-valence V in the V2CTx cathode was oxidized from V2+/V3+ to V4+/V5+ through initial charging activation, forming a nanoscale V oxide (VOx) coating and achieving more Zn-ion storage[62]. Currently, electrochemical activation, hydrothermal oxidation, and selenization are used to prepare MXene-based derivatives. V2CTx MXene cathodes have been held at different voltages for 2 h, and the obtained products were denoted as 1.4-V2CTx, 1.8-V2CTx, and 2.0-V2CTx [Figure 5G]. Among these MXenes, the outer surface of 1.8-V2CTx was coated with homogeneous nanoscale VOx and the internal V-C-V multilayer structure was retained, whereas 2.0-V2CTx was over-oxidized [Figure 5H and I]. Activated high-valence V species provide additional capacity, and internally retained V-C-V layers ensure high conductivity of the cathodes. Thus, the 1.8-V2CTx cathode had a specific capacity of 358 mA h g-1 even at 30 A g-1. In contrast to traditional acid and alkali etching, an in-situ electrochemical method that removes the Al layer and enhances the V valence of V2AlC has been proposed[63]. During the electrochemical process, the V2AlC cathode undergoes three sequential stages [Figure 6A]. (1) The Al layer is exfoliated by the fluorine-rich electrolyte; (2) V is gradually oxidized to V2O5; and (3) After a long cycle, the V2O5 particles are uniformly distributed on the retained carbon layer, leading to the formation of a V2O5/C/V2O5 structure. The resulting V2O5/C/V2O5 sandwich structure is shown in Figure 6B. The XRD patterns [Figure 6C] of the V2AlC cathode after long-term cycling also demonstrate the presence of V2O5 particles and carbon layers. The obtained cathode had a capacity of 409.7 mA h g-1 at 0.5 A g-1 and 95.7 mA h g-1 at 64 A g-1.
Figure 6. (A) Schematic diagram of electrochemical activation of V2AlC cathode at 5 A g-1. (B) Schematic diagram of the resulting uniform distribution of V2O5 particles on two-dimensional carbon layers. (C) XRD patterns of V2AlC cathode after long cycling at
The electrochemical properties of cathodes can also be effectively enhanced through ion insertion. MXene-derived materials intercalated with metal ions (such as Mg2+[64] and Zn2+[65]) have also been extensively studied. Based on the significant ion-adsorption capacity of MXenes, V2CTx was alkalized with KOH
MXenes and their derivatives as cathodes in AIBs
Al metal reserves are abundant. Al is the world’s most productive non-ferrous metal and can be processed easily. In addition, Al metal anodes exhibit a high theoretical capacity of 2,980 mA h g-1. Consequently, rechargeable AIBs have emerged as a promising energy-storage technology owing to their cost-effectiveness, superior safety, and high energy density[68,69]. However, a shortage of suitable cathode materials hinders the development of AIBs. The use of 2D MXenes as cathode materials in AIBs has been extensively studied. Metal ions such as Li+[70], Na+[71], K+[72], Mg2+[53], and Al3+[73] can be inserted into MXene layers, providing new opportunities for MMIBs that lack high-capacity and stable cathode materials[74].
V2CTx MXene was used as a cathode, and its reaction mechanism in AIBs is shown in Figure 7A. During discharging process, the [Al2Cl7]- ions dissociate into [AlCl4]- and Al3+ ions at the cathode/electrolyte interface, followed by the insertion of Al3+ ions into the MXene layers. For the anode, the Al metal undergoes a chemical reaction with [AlCl4]- ions, resulting in the formation of [Al2Cl7]- ions. Although the etching process removes the Al layers in MXenes, most MXene layers remain in the state of multilayer stacking, which is unfavorable for ion transport and reaction kinetics. The use of interpolators to further delaminate MXenes and increase the layer spacing can improve the ion-storage capacity. Fewer-layer V2CTx (TBAOH-FL-V2CTx) [Figure 7B] was the formation of multiple-layer V2CTx (ML-V2CTx) [Figure 7C] after treatment with tetrabutylammonium hydroxide (TBAOH)[75]. The XRD pattern [Figure 7D] shows that the interlayer spacing of TBAOH-FL-V2CTx increased by 5.73 Å compared to ML-V2CTx without TBAOH treatment. More uniform delamination endows the TBAOH-FL-V2CTx cathode with better electrochemical performance. The TBAOH-FL-V2CTx cathode had a discharge capacity of 300 mA h g-1 at 0.1 A g-1. In contrast, that of the ML-V2CTx cathode was approximately 100 mA h g-1 under the same conditions.
Figure 7. (A) Schematic representation of the reaction mechanism of the AIBs with V2CTx MXene as the cathode. (B) Schematic representation of TBAOH intercalation to increase ML-V2CTx MXene layer spacing. (C) Schematic representation of ML-V2CTx etching process. (D) XRD patterns of ML-V2CTx and TBAOH-FL-V2CTx. This figure is quoted with permission from VahidMohammadi et al. Copyright (2017) American Chemical Society[75]. (E) Schematic representation of preparing single-layer Nb2CTx MXene. (F) XRD patterns of P-Nb2CTx and C-Nb2CTx. This figure is quoted with permission from Li et al. Copyright (2022) American Chemical Society[73].
Based on single-layer MXene obtained by TBAOH treatment [Figure 7E], Li et al. adjusted the single-layer Nb2CTx MXene surface terminated species and removed interlayer water molecules by calcination, enhancing the capacity and cycle life of the cathode[73]. The large layer spacing (1.22 nm) caused by etching and delamination is conducive to ion transport in AIBs. In addition, the XRD patterns [Figure 7F] show that Nb2CTx MXene generated Nb2O5 and amorphous carbon after calcination, which likely contributed to the improved capacity and cycling performance of the cathode[76,77]. After calcination at 200 °C, the
MXenes and their derivatives as cathodes in MIBs
As an emerging advanced energy-storage technology, MIBs have garnered significant research interest, mainly because Mg is abundant (approximately 104 times that of lithium), has a lower redox potential
MXene materials have a unique layered structure that can be used directly as cathodes for Mg-ion storage in MIBs. To promote the reversible removal of Mg ions from MXenes, cetyltrimethylammonium bromide (CTAB) pre-intercalated Ti3C2 MXene was proposed as a cathode[53]. Density functional theory (DFT) verified that CTA+ can facilitate charge transfer to MXenes, thereby enhancing the migration kinetics of Mg atoms on the MXene surface, leading to a significant change in capacity from a value close to zero to a considerable value (300 mA h cm-3 at 50 mA g-1, Figure 8A). In addition to CTAB, carbon nanospheres have been proven to be effective intercalated materials for enhancing the performance of MXenes. Sandwich-structured MXene@C nanospheres were prepared via electrostatic interactions between Ti3C2Tx and carbon nanospheres [Figure 8B][78]. The BET surface area results showed that Ti3C2Tx@C exposed more surface area (103.8 m2 g-1) than pure Ti3C2Tx (12.7 m2 g-1), which further verified that carbon nanospheres can alleviate the restacking of MXene. For Ti3C2Tx@C, the (002) peak shifted to a lower angle than that of Ti3C2Tx. Thus, the intercalation of carbon nanospheres can expand the layer spacing of MXene, resulting in more active sites and diffusion paths for the Mg2+ ions. Therefore, the electrode had excellent Mg-ion storage ability (198.7 mA h g-1 at 0.01 A g-1 and 123.3 mA h g-1 at 0.2 A g-1) [Figure 8C]. In addition to expanding the interlayer spacing, processing 2D MXene nanosheets into 3D structures is an effective strategy for enhancing the electrochemical performance of MXenes. The 3D conductive Ti3C2 networks were prepared by introducing bacterial celluloses (BC) into MXenes to synthesize a flexible freestanding BC/Ti3C2 film
Figure 8. (A) GCD curves of the Ti3C2Tx/CTAB and Ti3C2Tx. This figure is quoted with permission from Xu et al. Copyright (2018) American Chemical Society[53]. (B) Schematic illustration of the synthesis process of Ti3C2Tx@C. (C) Schematic illustration of the
In addition to monometallic MXenes, bimetallic MXenes have also been investigated as energy-storage materials. Bimetallic MXenes utilize bimetallic elements at the M site and exhibit various advantages[80]. TiVCTx MXene not only combines the advantages of the high reducibility of V-based MXene and the high stability of Ti-based MXene, but also exhibits fewer atomic layers and higher conductivity. The synthesis process of TiVCTx is shown in Figure 8E. Therefore, when TiVCTx is directly used as a cathode without conductive additives, binders, and collectors[80], the TiVCTx film exhibits a high specific capacity
Summary of the electrochemical properties of MXenes and their derivatives as cathodes
Battery system | Cathode materials | Capacity | Cycle stability | Ref. |
ZIBs | alk-V2CTx | 498.2 mA h g-1 at 0.1 A g-1 | 96.2% after 20,000 cycles at 10 A g-1 | [34] |
Ti3C2Br2 | 97.6 mA h g-1 at 0.5 A g-1 | 80% after 1,000 cycles at 4 A g-1 | [61] | |
Ti3C2I2 | 135 mA h g-1 at 0.5 A g-1 | 80% after 700 cycles at 4 A g-1 | [61] | |
1.8-V2CTx | 358 mA h g-1 at 30 A g-1 | 81.4% after 2,000 cycles at 30 A g-1 | [62] | |
V2AlC | 409.7 mA h g-1 at 0.5 A g-1 | / | [63] | |
MVO@VC | 287.6 mA h g-1 at 10 A g-1 | 92.9% after 25,000 cycles at 10 A g-1 | [66] | |
VSe2@V2CTx | 231.3 mA h g-1 at 0.5 A g-1 | 93.1% after 600 cycles at 2.0 A g-1 | [67] | |
AIBs | TBAOH-FL-V2CTx | 300 mA h g-1 at 0.1 A g-1 | 42.1% after 100 cycles at 0.2 A g-1 | [75] |
C-Nb2CTx | 108 mA h g-1 at 0.2 A g-1 | 80% after 500 cycles at 0.5 A g-1 | [73] | |
MIBs | CTAB-Ti3C2 | 300 mA h cm-3 at 0.05 A g-1 | 63.1% after 250 cycles at 0.2 A g-1 | [53] |
Ti3C2Tx@C | 198.7 mA h g-1 at 0.01 A g‒1 | 85% after 400 cycles at 0.05 A g-1 | [78] | |
BC/Ti3C2 | 171 mA h g-1 at 0.05 A g-1 | 88% after 100 cycles at 0.05 A g-1 | [79] | |
TiVCTx | 111 mA h g‒1 at 0.05 A g‒1 | 64.8% after 1,000 cycles at 0.5 A g-1 | [80] |
MXene/transition metal oxide composites as cathodes
Manganese oxide as cathodes
Manganese (Mn)-based materials are extensively utilized in energy-storage devices because they offer advantages such as low cost, environmental friendliness, abundant reserves, and high theoretical capacity/voltage[81]. As a transition metal, Mn is abundant in oxidation valence states (bivalent, trivalent, and quadrivalent) and exists in a variety of Mn-based oxides, such as MnO2, MnO, Mn2O3, Mn3O4, and
MXene/manganese oxide composites as cathodes in ZIBs
MnO2 is the most studied Mn-based compound, and its composite with MXenes can take various forms, such as MnO2 particles grown on the surface of MXenes and the assembly of MnO2 nanosheets and MXene sheets by electrostatic interactions. MXenes act as a framework to ensure fast electron transfer, and the Mn dioxide provides a high capacity. Therefore, the composite material exhibits better electrochemical properties. K-V2C@MnO2 composites are obtained by alkalizing V2CTx MXenes (K-V2C) with KOH and growing MnO2in situ on K-V2C using the hydrothermal method [Figure 10A][85]. The K-V2C with inserted K+ can be used as the nucleation site of MnO2 in hydrothermal processes; meanwhile, the insertion of K+ increases the layer spacing of MXenes. An SEM image of K-V2C@MnO2 [Figure 10B and C] shows that the formed MnO2 grows uniformly on the K-V2C surface. XRD patterns show that the formed MnO2 is in the
Figure 10. (A) Schematic representation of the preparation of K-V2C@MnO2. (B and C) SEM image of K-V2C@MnO2. This figure is quoted with permission from Zhu et al. Copyright (2021) American Chemical Society[85]. (D) Schematic representation of the preparation process of the MnO2/MXene superlattice. (E) SEM image of the MnO2/MXene. This figure is quoted with permission from
In contrast to the above-mentioned Mn dioxide particles grown on layered MXenes, a 2D MnO2/MXene superlattice was synthesized using the solution-phase assembly method [Figure 10D][87]. The 2D
MXenes can be used not only for aqueous ZIBs but also for non-aqueous ZIBs. The 2D δ-MnO2@MXene heteronanosheets were obtained by deposition of δ-MnO2 nanosheets on MXene [Figure 10H][89]. The deposition was achieved through a chemical reaction between KMnO4 and polydopamine (PDA). In 0.5 M Zn triflate [Zn(OTf)2] in triethyl phosphate (TEP) organic electrolytes, the MnO2@MXene heteronanosheet exhibited only Zn2+ insertion and no H+ insertion, which avoided byproduct formation, Mn shuttling effects, and structural damage. At 10.5 mg cm-2, the MnO2@MXene heteronanosheet cathode achieved an areal capacity of 1.9 mAh cm-2. In addition to layered Mn dioxide, ZnMn2O4 spinel (ZMO) is also a promising energy-storage material. However, during charging and discharging, ZMO is transformed into MnO2. This transformation leads to the degradation of the spinel structure and the formation of irreversible inactive ZnO byproducts, resulting in poor cycling stability [Figure 10I][90]. Based on the above challenges, ZMO nanoparticles were assembled on Ti3C2Tx MXene sheets via a hydrothermal process to synthesize a
Vanadium oxide as cathodes
V has a variety of valence states, and V-based materials have high theoretical capacities and superior rate performances as cathodes[91-94]. The constituent units of the V-O coordination polyhedron include tetrahedrons, triangular bipyramids, and square pyramids, and these units can change according to the oxidation state of V. Consequently, numerous V oxides can be constructed by sharing the angles and/or edges of the polyhedra. However, the main challenge in V-based materials is capacity decay owing to structural damage and the dissolution of cathodes. V2O5·nH2O was reported to have an initial capacity of 273.9 mA h g-1 and retain less than 100 mA h g-1 after 20 cycles in ZIBs, indicating fast capacity decay[95]. The heterogeneous interface formed by the composite of V oxide and MXene facilitated rapid electron and ion transfer, thereby enhancing the electrochemical kinetics. Furthermore, the composite exhibited remarkable energy-storage capabilities at low temperatures.
MXene/vanadium oxide composites as cathodes in ZIBs
V-based oxides are ideal candidates for ZIB cathodes due to their multielectron transfer mechanism and large layer spacing, which are favorable for ion transport[96,97]. Designing V oxides and MXenes into heterostructures is a promising strategy for enhancing charge transport. As illustrated in Figure 11, the difference in the energy bands creates a built-in electric field within the heterostructure, facilitating electron transfer and ion diffusion. Zhang et al. prepared layer-stacked MVO + V2C heterostructures by electrostatic self-assembly using MnxV2O6 (MVO) nanobelts and V2C [Figure 12A], which was used as a cathode in ZIBs operating at a wide-temperature range of -20-40 °C[98]. Many nanochannels and built-in electric fields between the interstacked MnxV2O6 and V2CTx achieve rapid Zn2+ transport and improve the intrinsic conductivity and reaction kinetics. Furthermore, the presence of numerous nanochannel interlayers enhances the wettability of the electrolyte, thereby facilitating access to the electrodes. The cycling performance of the MVO + V2C cathode at -20 °C was tested [Figure 12B], and the results showed that the MVO + V2C cathode had a discharge capacity of 301.3 mA h g-1 at 0.5 A g-1, confirming that the MXene-based heterostructure endows ZIBs with excellent performance at a wide temperature range.
Figure 12. (A) Schematic of the preparation process of MVO + V2C heterostructures. (B) Cycle performance of MVO + V2C cathode at
In addition to MnxV2O6, H2V3O8 nanowires have been utilized to construct a built-in electric field with MXenes to promote ion transfer. The average valence of V in H2V3O8 is 4.67, and high-valence V can accommodate a large number of Zn ions[99]. H2V3O8/Ti3C2Tx was prepared using a one-step hydrothermal method, and the surface of delaminated Ti3C2Tx was uniformly covered with H2V3O8 nanowires
V2O5, a classical V oxide, has also been combined with MXenes to form a built-in electric field. V2O5 nanoplates with Ti3C2Tx MXene layers (VPMX) were assembled using van der Waals forces [Figure 12F][102]. Compared to the V2O5 cathode, MXene coated on the surface of V2O5 inhibited the dissolution of V and enhanced the structural stability of the VPMX cathode during charging and discharging [Figure 12G]. As shown in Figure 11, the heterogeneous interface between V2O5 and MXene allowed rapid electron transfer and improved the bulk electrochemical kinetics. Additionally, the water molecules in the VPMX cathode weakened the electrostatic interactions between the cathode and Zn2+, accelerating ion transport and stabilizing the structure. The VPMX cathode achieved a capacity of 243.6 mA h g-1 at 5.0 A g-1 and a long cycling life of over 5,000 cycles at 10 A g-1.
MXene/vanadium oxide composites as cathodes in AIBs
In addition to serving as a framework, the numerous functional groups on the MXene surface allow it to interact with other materials via the formation of M-O-Ti (M = metal atoms) and hydrogen bonds. Studies using the M-O-Ti bonds formed between VOPO4-2H2O and MXene to exfoliate nanosheets and obtain VOPO4-nH2O with different water contents have been reported. A VOPO4·nH2O@MXene composite was prepared by mechanical stirring to mix VOPO4·2H2O with different MXene contents (10%, 12%, 16%, and 48%) [Figure 12H][103]. The XRD patterns [Figure 12I] showed that with an increase in MXene, the (001) peak moved to a higher degree, and the number of water molecules in the VOPO4-2H2O interlayer was reduced, indicating that the force between MXene and VOPO4-2H2O affects the number of water molecules in the VOPO4-2H2O interlayer. The VOPO4-H2O@MXene composite with 12% MXene exhibited the best electrochemical properties among all MXene contents. The VOPO4-H2O@MXene cathode achieved a discharge capacity of 329.6 mA h g-1 at 1 A g-1 and a long cycling life of over 2,000 cycles at 1 A g-1 without capacity loss. The electrochemical properties of MXene/transition metal oxide composites as cathodes are summarized in Table 2[85,87-90,98,100-103].
Summary of the electrochemical properties of MXene/transition metal oxide composites as cathodes
Battery system | Cathode materials | Capacity | Cycle stability | Ref. |
ZIBs | K-V2C@MnO2 | 408.1 mA h g-1 at 0.3 A g-1 | 99.1% after 10,000 cycles at 10 A g-1 | [85] |
MnO2/MXene | 315.1 mA h g-1 at 0.2 A g-1 | 88.1% after 5,000 cycles at 5 A g-1 | [87] | |
Ti3C2Tx@MnO2 | 301.2 mA h g-1 at 0.1 A g-1 | 90.6% after 2,000 cycles at 0.5 A g-1 | [88] | |
δ-MnO2@MXene | 163 mA h g-1 at 0.1 A g-1 | 84.5% after 1,000 cycles at 0.1 A g-1 | [89] | |
ZMO@Ti3C2Tx | 172.6 mA h g-1 at 0.1 A g-1 | 92.4% after 5,000 cycles at 1 A g-1 | [90] | |
ZIBs | MVO + V2C | 301.3 mA h g-1 at 0.5 A g-1 (-20 °C) | 100% after 300 cycles at 0.5 A g-1 | [98] |
MVO + V2C | 301.3 mA h g-1 at 0.5 A g-1 (40 °C) | 100% after 120 cycles at 0.5 A g-1 | [98] | |
H2V3O8/MXene | 437.3 mA h g-1 at 3.0 A g-1 | 76.9% after 9,000 cycles at 10 A g-1 | [100] | |
2D HVO@Ti3C2 VPMX73 | 457.1 mA h g-1 at 0.2 A g-1 402.5 mA h g-1 at 0.2 A g-1 | 88.9% after 1,000 cycles at 5 A g-1 99.5% after 5,000 cycles at 10 A g-1 | [101] [102] | |
VPMX73 | 402.5 mA h g-1 at 0.2 A g-1 | 99.5% after 5,000 cycles at 10 A g-1 | [102] | |
AIBs | VOPO4-H2O@MXene | 329.6 mA h g-1 at 1 A g-1 | 100% after 2,000 cycles at 1 A g-1 | [103] |
MXene/sulfur-based material composites as cathodes
Sulfur-based material as cathodes
Sulfur is a lightweight, environmentally friendly element that is abundant on Earth, and sulfur cathodes have a high theoretical capacity (1,675 mA h g-1)[104]. Therefore, sulfur conversion cathodes offer high energy density, cost-effectiveness, and environmental friendliness and can be used in MMIBs. Except for sulfur cathodes, metal sulfides have the advantages of tunable layer spacing and high polarizability. Therefore, metal sulfides are promising candidate cathode materials for MMIBs. Sulfur atoms have a lower electronegativity than oxygen atoms, and the electrostatic interaction between the inserted multivalent ions and metal sulfides is smaller, which helps the electrode structure to remain stable. Metal sulfides, such as Mo6S[105], Co9S8[106], MoS2, WS2, VS2, and VS4, have been used to investigate cathodes[107]. However, sulfur cathodes face challenges such as active material polysulfide dissolution in the electrolyte and sluggish polysulfide transformation kinetics. The development of metal sulfides is hindered by their poor electrical conductivities, which are detrimental to ion transport. For example, pure MoS2 delivers a capacity of only
MXene/sulfur-based material composites as cathodes in ZIBs
Since the electronegativity of S2- is lower than that of O2-, V-based sulfide cathodes have a weaker electrostatic interaction with the bivalent Zn2+ than that of V-based oxide cathodes, thus allowing faster ion diffusion. As a promising cathode candidate for ZIBs, layered VS2 has a large layer spacing and abundant V redox chemical properties. However, the relatively low electrical conductivity and severe agglomeration of VS2 nanosheets deteriorate their electrochemical performance. A new 3D interconnected VS2⊥V4C3Tx heterostructural material was prepared using the solvothermal method [Figure 13A][109]. VS2 nanosheets were uniformly distributed on the surface of the V4C3Tx MXene framework, and this framework improved the conductivity of the cathode. Simultaneously, MXene effectively suppressed the volume change of VS2. Thus, the use of VS2 nanosheets as active materials contributes to their high capacity. Moreover, V4C3Tx MXene is used as a Zn anode coating, which inhibits the growth of Zn dendrites during cycling[110] and provides the anode with a more uniform electric field distribution[111]. The V4C3Tx@Zn symmetrical battery showed excellent cycling stability for approximately 1,700 h at 1 mA cm-2. The full cell was assembled with the VS2⊥V4C3Tx heterostructural material as the cathode and Zn foil with a protective layer of V4C3Tx MXene on the surface as the anode [Figure 13B]. The VS2⊥V4C3Tx||V4C3Tx@Zn battery had a capacity of 157.1 mA h g-1 after 5,000 cycles at 5 A g-1.
Figure 13. (A) Schematic diagram for the preparation for the VS2⊥V4C3Tx material. (B) The schematic diagram of the
MXene/sulfur-based material composites as cathodes in AIBs
Metal-sulfide/MXene composites have also been used as cathodes for AIBs. Encapsulating the active material particles with MXenes can reduce the loss of the active material and inhibit the aggregation of nanoparticles, thereby ensuring sufficient active sites and improving the electrochemical performance of the composite cathode. The synthesis of a new type of cobalt (Co) sulfide nanoparticles/MXene composite is shown in Figure 13C[112]. In the first step, a spongy 3D precursor of ZIF-67 encapsulated in an interlayer of MXene was prepared by in-situ growth and self-assembly. In the second step, the ZIF-67@MXene precursor and an appropriate amount of S powder were annealed at high temperatures. After carbonization and vulcanization, a 3D MXene-wrapped Co sulfide nanoparticle composite embedded in a hollow carbon nanobox (Co9S8 NP@NPC@MXene) was prepared. Compared to the Co9S8 NP@NPC composite,
MXene/sulfur-based material composite as cathode in MIBs
Sulfur is an ideal cathode material owing to its abundance, cost-effectiveness, and high theoretical capacity[113]. Nevertheless, sulfur faces various challenges, such as poor electrical conductivity, slow electron and ion transport, and polysulfide shuttling[114]. MXenes with high electronic conductivities and rich termination groups have been demonstrated to effectively increase sulfur utilization[115]. Therefore, the combination of sulfur and MXenes can effectively exert synergistic effects on both materials. MXenes as sulfur host materials in Mg-S batteries were first reported in 2021[116]. The S8-sandwich cathode is composed of Ti3C2Tx, carbon nanotubes (CNT) and S8 nanoparticles through low-temperature vacuum-filtration methods without a current collector or binder. Notably, as shown in Figure 14A, the S8-sandwich is a sandwich-like architecture, where MXene acts as an interlayer with a mixed cathode of S8 and CNT. S8, as the source of redox-active sulfur, makes a major contribution to the capacity; CNT plays a critical role in the formation of the conductive network, and MXene with polar termination groups reduces sulfur shuttle and confines the sulfur species. The GCD curves demonstrate that the MXene interlayer significantly improves the capacity and average voltage. Therefore, MXene increases the discharge capacity of the electrode material from 290 to 530 mA h g-1, with 83% capacity retention over 25 cycles. Furthermore, a Ti3C2@CoO heterostructure was synthesized as sulfur hosts via a self-assembly and annealing process[117]. DFT confirms that CoO can guarantee more Mg polysulfide adsorption during the cycle, and Ti3C2 is favorable for Mg-ion diffusion [Figure 14B]. Batteries were assembled using S-Ti3C2@CoO as the cathode, Mg foils as the anode, and 1 M Mg(TFSI)2/AlCl3/diglyme as the electrolyte. The battery exhibited an excellent capacity of
Figure 14. (A) Schematic illustration of S8-mixed cathode. This figure is quoted with permission from Kaland et al. Copyright (2021) John Wiley and Sons[116]. (B) Schematic diagram of magnesium polysulfide redox mechanism of the S-Ti3C2@CoO electrode. This figure is quoted with permission from Xu et al. Copyright (2022) Elsevier[117]. (C) Cycle performance and SEM image of MoS2/Ti3C2Tx. This figure is quoted with permission from Xu et al. Copyright (2018) Elsevier[108]. (D) Schematic illustration of CuS/d-Ti3C2Tx structure. This figure is quoted with permission from Cheng et al. Copyright (2023) Royal Society of Chemistry[118]. SEM images of (E) Ti3C2, (F) VS4 and (G) VS4@Ti3C2/C. This figure is quoted with permission from Zhu et al. Copyright (2022) Elsevier[119].
The fabrication of MXene composites with metal sulfides is a common strategy for exploring new materials. A petal-like MoS2/Ti3C2Tx composite was prepared using a hydrothermal method[108]. MXene acts as a substrate to improve the conductivity of the composite, and MoS2 grows on the MXene surface to provide abundant active sites for Mg2+ storage. The TEM images show that MoS2/Ti3C2Tx has a fluffy petal-like nanosheet morphology and that the MoS2 layers in the composite are thinner than those in bare MoS2, which is beneficial for exposing more active sites and Mg-ion diffusion channels. Owing to the high conductivity and abundant surface functional groups of MXene, coupled with the high capacity of few-layer MoS2 nanosheets, MoS2/Ti3C2Tx exhibits excellent electrochemical capacity (165 mA h g-1 at
In addition, V-based sulfides can be combined with MXenes via hydrothermal and electrostatic self-assembly strategies. VS4 nanosheets have been generated in situ on a carbon-coated Ti3C2 MXene matrix (denoted as VS4@Ti3C2/C)[119]. According to the V 2p spectrum, two peaks were observed at 513.8 and
Summary of the electrochemical properties of MXene/sulfur-based material composites as cathodes
Battery system | Cathode materials | Capacity | Cycle stability | Ref. |
ZIBs | VS2⊥V4C3Tx | 273.9 mA h g-1 at 1 A g-1 | 69.6% after 2,000 cycles at 5 A g-1 | [109] |
AIBs | Co9S8NP@NPC@MXene | 277 mA h g-1 at 0.1 A g-1 | 66.1% after 1,000 cycles at 1 A g-1 | [112] |
CoSe2@NPC@MXene | 288 mA h g-1 at 1 A g-1 | 76.4% after 300 cycles at 1 A g-1 | [112] | |
MIBs | S8-CNT-MXene | 530 mA h g‒1 at 0.05 A g‒1 | 83% after 25 cycles at 0.05 A g-1 | [116] |
Ti3C2@CoO | 1,540 mA h g‒1 at 0.1 A g‒1 | 35.1% after 70 cycles at 0.1 A g-1 | [117] | |
MoS2/Ti3C2Tx | 165 mA h g‒1 at 0.05 A g‒1 | 65.5% after 50 cycles at 0.05 A g-1 | [108] | |
CuS/d-Ti3C2Tx | 336.5 mA h g-1 at 0.05 A g-1 | 75% after 1,000 cycles at 1 A g-1 | [118] | |
VS4@Ti3C2/C | 492 mA h g‒1 at 0.05 A g‒1 | 80% after 900 cycles at 0.5 A g-1 | [119] |
MXene/selenium-based material composites as cathodes
Selenium-based material as cathodes
Selenium is less electronegative than oxygen and sulfur, facilitating the reduction of strong interactions between the host material and the intercalated ions. Therefore, selenium-based materials have a broad range of applications in MMIBs. Classical layered selenides have also received considerable attention. Their metal atoms covalently bond with adjacent selenium layers to form an interlayer structure (Se-M-Se), and the individual interlayer structures of Se-M-Se are connected via van der Waals forces. These properties allow rapid ion transport in the channels[120]. However, the electrochemical stability of metal selenides is poor, mainly owing to their low electrical conductivity, volume expansion, and dissolution of the cathode active material during cycling. In addition, stacking problems may occur in layered selenides. Pure VSe2 exhibits a discharge capacity of 159.6 mA h g-1 at 0.5 A g-1 in ZIBs[121]. Therefore, the combination of selenium-based materials with MXenes having high conductivity and large ion channels can inhibit the lattice distortion caused by anion insertion during cycling and contribute to the stability of the electrode structure.
MXene/selenium-based material composites as cathodes in AIBs
MXene/selenium-based material composites provide the possibility of advanced cathodes for AIBs. The 2D composite was prepared by mixing V2C powder with selenium (V2C@Se) at a mass ratio of 1:2 and calcining it at a temperature of 500 °C [Figure 15A][122]. After selenium doping, large amounts of V2+ were oxidized to V4+, and functional groups such as -O, -OH, -F and -Cl on the surface of V2C were reduced and replaced by selenium atoms[123]. Selenium is also involved in redox reactions, and reversible transformation of Se2- and Se2+ occurs during charging and discharging [Figure 15B]. Therefore, the reversible redox reactions on the V2C@Se cathode are mainly V2+/V3+, V4+/V5+, and Se2-/Se2+. The capacity of the V2C@Se reached
Figure 15. (A) Schematic of the preparation process of V2C@Se. (B) XPS spectra of Se 3d in V2C@Se during charging and discharging process. This figure is quoted with permission from Lv et al. Copyright (2022) Elsevier[122]. (C) Theoretical simulation analysis with
To explore the mechanism of poor cycling stability and low Coulombic efficiency in AIBs, Yuan et al. analyzed the effect of embedding common carriers (AlCl-4, Al2Cl-7, Al3+, and Cl-) in CoSe2 lattices via DFT[124]. As shown in Figure 15C, the insertion of AlCl-4 into the CoSe2 material required a large amount of energy (3.30 eV). In contrast, the energies required for the insertion of Al3+ and Cl- into the CoSe2 material were -3.27 and 0.99 eV, respectively, which did not cause large lattice distortions. Therefore, the growth of CoSe2 on the surface of MXene can enhance the performance of cathode materials by suppressing lattice distortion. The CoSe2@TiO2/Ti3C2 cathode provided a stable discharge capacity of 197 mA h g-1 at 0.2 A g-1, and a retaining capacity of 102 mA h g-1 after 500 cycles at 1.6 A g-1 (the capacity of CoSe2 cathode was only 25 mA h g-1).
MXene/selenium-based material composites as cathodes in MIBs
Utilizing metal-organic frames (MOFs) to induce metal selenides is an effective strategy for preparing cathode materials. The Ti3C2/CoSe2 heterostructure was prepared by the in-situ selenization of the Ti3C2/ZIF-67 composite [Figure 15D][125]. The O 1s spectrum revealed a peak at 529.1 eV, which was assigned to Co-O-Ti, suggesting a strong covalent bond between the Co atoms in CoSe2 and the oxygen-containing functional groups from Ti3C2. In the heterostructure Ti3C2/CoSe2, CoSe2 provides a high capacity for Mg-ion storage, and MXene acts as a structural skeleton and promotes rapid charge transfer at the interface. Therefore, electrodes exhibit an excellent rate performance (75.7 mA h g-1 at 1,000 m A g-1) and cycling stability (79% capacity retention after 500 cycles). Significantly, Ti3C2/CoSe2 matched with gel polymer electrolytes exhibits outstanding electrochemical performance (67.3 mA h g-1 at 50 mA g-1 over 70 cycles) in quasi-solid-state MIBs.
MOFs, as self-sacrificing templates, were reported to combine with TiVCTx MXene, resulting in the formation of a NiSe2-CoSe2@TiVCTx (NCSe@TiVC) heterostructure [Figure 15E][126]. The SEM images showed a unique 3D porous spherical structure with many active sites. DFT calculations and electrochemical impedance spectroscopy (EIS) proved that the conductivity of NCSe@TiVC was significantly improved compared with that of NiSe2-CoSe2. In addition, DFT was used to analyze the optimal Mg-ion adsorption sites in the structure and demonstrate that the as-prepared heterostructure promoted rapid charge transfer. NCSe@TiVC exhibited excellent electrochemical properties (136 mA h g-1 at 0.05 A g-1 after 100 cycles). It is worth noting that the assembled flexible pouch-cell device demonstrated excellent electrical performance (104 mA h g-1 at 0.1 A g-1 upon 200 cycles) when subjected to bending at various angles. The electrochemical properties of MXene/selenium-based material composites as cathodes are summarized in Table 4[122,124-126].
Summary of the electrochemical properties of MXene/selenium-based material composites as cathodes
Battery system | Cathode materials | Capacity | Cycle stability | Ref. |
AIBs | V2C@Se | 402.5 mA h g-1 at 1 A g-1 | 29.8% after 1,000 cycles at 1 A g-1 | [122] |
CoSe2@TiO2/Ti3C2 | 197 mA h g-1 at 0.2 A g-1 | 88% after 500 cycles at 1.6 A g-1 | [124] | |
MIBs | Ti3C2/CoSe2 | 75.7 mA h g-1 at 1 A g-1 | 79% after 500 cycles at 0.05 A g-1 | [125] |
NCSe@TiVC | 136 mA h g-1 at 0.05 A g-1 | 93.6% after 500 cycles at 0.5 A g-1 | [126] |
Other MXene composites as cathodes
In addition to the aforementioned materials, combinations of MXenes with other materials possessing numerous ion diffusion pathways and stable structures have also been explored for MMIB cathodes. Specifically, 2D layered double hydroxides (LDHs), iodine (I2), organic cathodes, and MOFs have been demonstrated to enhance the electrochemical performance of cathodes when combined with MXenes.
Other MXene composites as cathodes in ZIBs
LDHs are special layered materials consisting of multiple positively charged layers with anions in the middle, which can also be used as cathodes for ZIBs. Combining LDHs with MXene can help avoid a sharp drop in the capacity of the LDH cathode during charging and discharging. The 2D/2D Co-doped Ni-Mn-LDH/V2CTx MXene (CNMV) was prepared from positively charged Co-doped LDH and negatively charged 2D V2CTx MXene using simultaneous ion doping and electrostatic assembly methods[127]. The LDH nanoflowers in the CNMV composite were tightly wrapped around the delaminated MXene nanosheets
Figure 16. (A) Schematic representation for the synthesis of CNMV hybrid. This figure is quoted with permission from Zhang et al. Copyright (2021) Elsevier[127]. (B) Schematic illustration for the synthesis method of TAP/Ti3C2Tx. (C) Electrostatic potential of TAP molecule after storing Zn2+ and H+ ions. This figure is quoted with permission from Wang et al. Copyright (2022) John Wiley and Sons[130]. (D) SEM image of Cu-HHTP/MXene. (E) HRTEM image of Cu-HHTP/MXene. This figure is quoted with permission from Wang et al. Copyright (2022) John Wiley and Sons[31]. (F) Schematic illustration for preparation of polyaniline/MXene hydrogel by chemical oxidation polymerization. This figure is quoted with permission from Wang et al. Copyright (2024) Royal Society of Chemistry[134].
I2-Zn batteries have been studied extensively owing to their low cost and safety[128]. However, because elemental I2 is insulating, excellent substrate conductivity is required to ensure fast electron transport. In addition, the shuttle effect of the I species (I2, I-, and I-3) during cycling causes a loss of active material, leading to capacity decay. The emergence of MXenes has provided a new approach to realize high-performance I2-Zn batteries. The electrodeposited I2-Nb2CTx MXene material (eIM) was prepared by inserting I- ions into the MXene layers under an electric field and in-situ oxidization of I- into linear I2 molecules at 1.6 V[129]. In the eIM, linear I2 molecules were uniformly distributed on the highly conductive Nb2CTx MXene surface, providing more active sites and enabling fast electron transport. However, the nanoscale interlayer spacing of Nb2CTx MXene restricted the I species to the interlayer, reducing the loss of the active material. Therefore, the I2-Zn battery with eIM as the cathode has an excellent rate performance (205 mA h g-1 at 1.0 A g-1 and 143 mA h g-1 at 18 A g-1).
In addition to various inorganic materials, MXenes can be combined with organic cathodes to improve the electrochemical performance. Tri (aza) pentaenene (TAP), which has extended conjugated effects along the C=N chains, was injected into Ti3C2TX MXene, and the obtained TAP/Ti3C2TX composite is shown in Figure 16B[130]. Because of steric effects, the abundant C=N sites in the TAP molecules can selectively store H+ and Zn2+. Based on the electrostatic potential of the TAP molecule [Figure 16C], the C=N bonds with the lowest electrostatic potential in the blue region can store H+ and Zn2+ ions[131,132]. The calculation results based on the charge density difference and Bader charge showed charge depletion on the surface of Ti3C2Tx and charge accumulation on the surface of the TAP. This demonstrated the intimate electronic interactions between TAP and Ti3C2Tx, which maintained the structural stability of the TAP/Ti3C2Tx cathode. The TAP/Ti3C2Tx cathode exhibited a discharge capacity of 303 mA h g-1 at 0.04 A g-1 and remarkable cycle stability of 10,000 times at 1 A g-1.
MOFs are also used as advanced energy-storage materials owing to their large one-dimensional channel in MOFs[133]. The combination with MXenes is expected to enhance the electrical conductivity and structural stability. The Cu-HHTP/MXene heterostructure material consists of alternating stacks of Cu-HHTP MOF and MXene formed by electrostatic self-assembly[31]. The SEM [Figure 16D] and HRTEM images
Other MXene composites as cathodes in AIBs
Organic cathodes are also an important research topic for AIBs. Although most organic cathodes are environmentally friendly and have a long cycle life, they have a low specific capacity. Combining organic cathodes with MXenes is expected to increase battery capacity. The anthraquinone derivative
Figure 17. (A) Schematic representation of the synthetic process of MXene@BDTO. (B) SEM image of MXene@BDTO. (C) SEM image of BDTO powders. This figure is quoted with permission from Wu et al. Copyright (2022) Elsevier[135].
Summary of the electrochemical properties of other MXene composites as cathodes
Battery system | Cathode materials | Capacity | Cycle stability | Ref. |
ZIBs | CNMV | 322.7 mA h g-1 at 0.2 A g-1 | 95.7% after 600 cycles at 1 A g-1 | [127] |
I2-Nb2CTx | 205 mA h g-1 at 1 A g-1 | 80% after 23,000 cycles at 6 A g-1 | [129] | |
TAP/Ti3C2Tx | 303 mA h g-1 at 0.04 A g-1 | 81.6% after 10,000 cycles at 1 A g-1 | [130] | |
Cu-HHTP/MXene | 260.1 mA h g-1 at 0.1 A g-1 | 92.5% after 1,000 cycles at 4 A g-1 | [31] | |
PANI/MXene | 219 mA h g-1 at 0.2 A g-1 | 88.3% after 5,000 cycles at 5 A g-1 | [134] | |
AIBs | MXene@BDTO | 229.8 mA h g-1 at 0.5 A g-1 | 58.7% after 500 cycles at 0.5 A g-1 | [135] |
CONCLUSIONS AND PERSPECTIVES
This review summarizes the progress of MXenes as cathodes for MMIBs. In MMIBs, cathode materials, as host materials for metal ion intercalation/deintercalation, encounter the following main challenge: The high charge density carried by multivalent metal ions can generate strong Coulombic interactions with the cathode material, leading to high energy barriers, which may induce adverse changes, such as irreversible phase transition, dissolution of active materials, and structural collapse of the cathode material. Therefore, it is crucial to improve the conductivity of charge carriers and expand the ion accommodation space to enhance cathode performance. MXenes, as 2D layered transition metal carbon/nitrides, have outstanding electrical conductivity that promotes charge transfer and accelerates electrochemical reaction kinetics. Moreover, their high surface area is conducive to increasing the number of active sites, which can accommodate more ions. In addition, MXenes exhibit other physicochemical properties, including superior mechanical properties, excellent chemical stability, and strong adjustability. Therefore, MXenes can serve not only as host materials for energy storage, but also as matrices that can be combined with other materials to enhance the electrical conductivity and increase the number of active sites of the cathode, thereby enhancing the electrochemical performance.
When MXenes are employed as the cathode material in MMIBs, the strong correlation between the properties of the charge carriers and the electrochemical properties of MXenes is a crucial aspect that must be considered. During the cycling process, the metal ions in the electrolyte enter the lattice interstices of MXenes. First, the metal ions must undergo desolvation at the electrolyte-electrode interface before intercalation. However, because of the high charge of multivalent ions, there is a strong interaction between multivalent ions and solvent molecules such as Zn(H2O)x2+, MgCl+, and AlCl-4, leading to slow ion diffusion, high interface transfer resistance, and low stability of the cathode structure. When metal ions enter the layered MXenes from the interface, owing to the difference in site energy and coordination preferences of multivalent metal ions in the cathode structure, the metal ions exhibit different migration energy barriers. In a layered MXene structure, Zn2+ has a common coordination number (4), whereas Mg2+ and Al3+ have a coordination number of 6. Therefore, the migration energy barrier of Zn ions is lower than that of Mg ions, whereas Al ions exhibit a high migration energy barrier owing to the strong electrostatic interactions caused by their high charge density. In addition, the electrostatic interactions between multivalent metal ions and MXenes are different, leading to ion hysteresis in the cathode, which triggers structural collapse and irreversible phase transitions, significantly affecting the battery cycle life.
Although significant progress has been made in the application of MXenes as cathodes, there is still room for further development. Therefore, based on our understanding of how to promote the advancement of MXenes, we propose several suggestions and directions.
1. The MXene cathodes in MMIBs are predominantly Ti3C2 and V2C. Consequently, it is necessary to explore other MXenes for potential application in MMIBs. Moreover, MXenes have many different terminal groups, such as F, S, Te, Se, and NH. Their terminal functional groups can be customized to meet specific performance requirements. However, the impact of terminal groups on performance has rarely been investigated. Therefore, the roles of different terminal groups on the cathodes of MMIBs should be explored, and more attention should be paid to other MXene composites. In the future, the combination of MXenes and other active materials, which may create unexpected effects, should be investigated further.
2. When MXenes or their composites are used as cathode materials, their stabilities must be considered. Owing to the strong hydrogen bonds and interlayer van der Waals forces of MXenes, they tend to agglomerate and stack during cycling, leading to a reduction in the number of active sites and an acceleration of the structural collapse of the composite. Moreover, MXenes are sensitive to water and oxygen and their surfaces are easily oxidized. Therefore, to ensure the stability of the cathode structure, it is necessary to focus on improving the stability of MXenes.
3. MXene application scenarios should be expanded, and additional MXene properties and functions should be explored. MXenes have great application potential, but research on their electrochemical performance over a wide range of temperatures is insufficient. In the future, additional applications of MXenes in high- and low-temperature environments should be explored. Moreover, the significant flexibility of MXenes is an outstanding advantage for flexible batteries. Thus, the design of flexible MXene composites could be improved by including more application scenarios in the future. In addition, areas such as microbatteries and degradable batteries will become future research hotspots, and the application of MXenes in these areas should also be considered.
4. Currently, most synthetic solutions for MXenes are based on HF or fluorine-based salt etching. These etchers cause environmental pollution and operational risks, which hinder the large-scale production of MXenes. In addition, fluorine-based etchers inevitably introduce the F element, which is harmful to green manufacturing and limits the application of MXenes. As research and synthesis technologies continue to advance, novel synthesis approaches, such as CVD, are being explored, and MXenes will have broader application prospects in the field of MMIBs. Notably, 3D MXenes are conducive for increasing the number of active sites of the material. Combining 3D MXenes with other materials can alleviate the volume expansion problem of cathode materials. Therefore, more attention should be paid to the applications of 3D MXenes in future studies.
5. There are still many urgent problems that need to be solved for cathode materials in MMIBs. The electrochemical stability and capacity of multivalent ion batteries require further enhancement. This is the key to reducing the migration barrier of multicharged ions in MXenes. Defect engineering has been proven to be an effective strategy. Nevertheless, the loss of lattice oxygen and transformation of local structures can potentially lead to structural collapse. It is worth exploring future directions for accurately regulating the local structure to improve the material properties. The high charge density of multivalent metal ions results in high solvation energy. Employing MXenes with tunable functional group properties to achieve the rapid desolvation of metal ions at interfaces represents a promising direction. In addition, the energy-storage mechanism of cathode materials must be further explored.
6. The batteries studied mainly involved coin-type cells, which tend to be proof-of-concept and cannot accurately evaluate the effects of MXenes in practical applications. Therefore, future studies should examine other battery types, including pouch and cylindrical batteries. In addition, to achieve large-scale applications, researchers should focus on the electrochemical performance of batteries at low current densities and cathode materials with high loads and consider practical issues such as cost, safety, and environmental protection[98].
In summary, MXenes have broad application prospects as advanced cathodes in MMIBs. We hope that this review will help readers better understand the applications of MXenes as cathodes in MMIBs. We also hope that MXenes will overcome these challenges as soon as possible and achieve large-scale commercial applications.
DECLARATIONS
Authors’ contributions
Manuscript drafting and editing and technical support: Zhao X, Ruan C
Substantial contributions to conception and design of the study, and manuscript editing: Wang S, Liu H
Assistance in the design of the study, and administrative support: Sang Y
Availability of data and materials
Not applicable.
Financial support and sponsorship
This work is supported by the National Natural Science Foundation of China (Grant no. 92372118, 52072224).
Conflicts of interest
All authors declared that there are no conflicts of interest.
Ethical approval and consent to participate
Not applicable.
Consent for publication
Not applicable.
Copyright
© The Author(s) 2024.
REFERENCES
1. Zhang N, Chen X, Yu M, Niu Z, Cheng F, Chen J. Materials chemistry for rechargeable zinc-ion batteries. Chem Soc Rev 2020;49:4203-19.
2. Chao D, Zhou W, Xie F, et al. Roadmap for advanced aqueous batteries: from design of materials to applications. Sci Adv 2020;6:eaba4098.
3. Yao H, Yu H, Zheng Y, et al. Pre-intercalation of ammonium ions in layered δ-MnO2 nanosheets for high-performance aqueous zinc-ion batteries. Angew Chem Int Ed 2023;62:e202315257.
4. Zhao Y, Zhang S, Zhang Y, et al. Vacancy-rich Al-doped MnO2 cathodes break the trade-off between kinetics and stability for high-performance aqueous Zn-ion batteries. Energy Environ Sci 2024;17:1279-90.
5. Zhong C, Liu B, Ding J, et al. Decoupling electrolytes towards stable and high-energy rechargeable aqueous zinc-manganese dioxide batteries. Nat Energy 2020;5:440-9.
6. Li H, Zhang F, Wei W, et al. Promoting air stability of Li anode via an artificial organic/inorganic hybrid layer for dendrite-free lithium batteries. Adv Energy Mater 2023;13:2301023.
7. Wu F, Maier J, Yu Y. Guidelines and trends for next-generation rechargeable lithium and lithium-ion batteries. Chem Soc Rev 2020;49:1569-614.
8. Jing F, Liu Y, Shang Y, et al. Dual ions intercalation drives high-performance aqueous Zn-ion storage on birnessite-type manganese oxides cathode. Energy Stor Mater 2022;49:164-71.
9. Yang X, Wang X, Xiang Y, Ma L, Huang W. Asymmetric electrolytes design for aqueous multivalent metal ion batteries. Nanomicro Lett 2023;16:51.
10. Chen S, Zhao D, Chen L, et al. Emerging intercalation cathode materials for multivalent metal-ion batteries: status and challenges. Small Struct 2021;2:2100082.
11. Pan Z, Liu X, Yang J, et al. Aqueous rechargeable multivalent metal-ion batteries: advances and challenges. Adv Energy Mater 2021;11:2100608.
12. Liang Y, Dong H, Aurbach D, Yao Y. Current status and future directions of multivalent metal-ion batteries. Nat Energy 2020;5:646-56.
13. Liu Z, Qin L, Cao X, et al. Ion migration and defect effect of electrode materials in multivalent-ion batteries. Prog Mater Sci 2022;125:100911.
14. Zhang CJ, Anasori B, Seral-Ascaso A, et al. Transparent, flexible, and conductive 2D titanium carbide (MXene) films with high volumetric capacitance. Adv Mater 2017;29:1702678.
15. Amiri A, Chen Y, Bee Teng C, Naraghi M. Porous nitrogen-doped MXene-based electrodes for capacitive deionization. Energy Stor Mater 2020;25:731-9.
16. Ling Z, Ren CE, Zhao MQ, et al. Flexible and conductive MXene films and nanocomposites with high capacitance. Proc Natl Acad Sci USA 2014;111:16676-81.
17. An Y, Tian Y, Shen H, Man Q, Xiong S, Feng J. Two-dimensional MXenes for flexible energy storage devices. Energy Environ Sci 2023;16:4191-250.
18. Abid MZ, Rafiq K, Aslam A, Jin R, Hussain E. Scope, evaluation and current perspectives of MXene synthesis strategies for state of the art applications. J Mater Chem A 2024;12:7351-95.
19. Jing H, Yeo H, Lyu B, et al. Modulation of the electronic properties of MXene (Ti3C2Tx) via surface-covalent functionalization with diazonium. ACS Nano 2021;15:1388-96.
20. Tian Y, An Y, Feng J, Qian Y. MXenes and their derivatives for advanced aqueous rechargeable batteries. Mater Today 2022;52:225-49.
21. Liu H, Ma Y, Cao B, Zhu Q, Xu B. Recent progress of MXenes in aqueous zinc-ion batteries. Acta Phys Chim Sin 2023;39:2210027.
22. Liu P, Liu W, Liu K. Rational modulation of emerging MXene materials for zinc-ion storage. Carbon Energy 2022;4:60-76.
23. Javed MS, Mateen A, Ali S, et al. The emergence of 2D MXenes based Zn-ion batteries: recent development and prospects. Small 2022;18:e2201989.
24. Wei C, Tao Y, An Y, et al. Recent advances of emerging 2D MXene for stable and dendrite-free metal anodes. Adv Funct Mater 2020;30:2004613.
25. Zheng S, Zhao W, Chen J, Zhao X, Pan Z, Yang X. 2D materials boost advanced Zn anodes: principles, advances, and challenges. Nanomicro Lett 2023;15:46.
26. Wang C, Pan Z, Chen H, Pu X, Chen Z. MXene-based materials for multivalent metal-ion batteries. Batteries 2023;9:174.
27. Liu H, Zhang X, Zhu Y, et al. Electrostatic self-assembly of 0D-2D SnO2 quantum dots/Ti3C2Tx MXene hybrids as anode for lithium-ion batteries. Nanomicro Lett 2019;11:65.
28. Xiong D, Shi Y, Yang HY. Rational design of MXene-based films for energy storage: progress, prospects. Mater Today 2021;46:183-211.
29. Bashir T, Zhou S, Yang S, et al. Progress in 3D-MXene electrodes for lithium/sodium/potassium/magnesium/zinc/aluminum-ion batteries. Electrochem Energy Rev 2023;6:5.
30. Liu Z, Zhang Y, Zhang HB, et al. Electrically conductive aluminum ion-reinforced MXene films for efficient electromagnetic interference shielding. J Mater Chem C 2020;8:1673-8.
31. Wang Y, Song J, Wong WY. Constructing 2D sandwich-like MOF/MXene heterostructures for durable and fast aqueous zinc-ion batteries. Angew Chem Int Ed 2023;62:e202218343.
32. Wang C, Chen S, Song L. Tuning 2D MXenes by surface controlling and interlayer engineering: methods, properties, and synchrotron radiation characterizations. Adv Funct Mater 2020;30:2000869.
33. Dall'agnese Y, Lukatskaya MR, Cook KM, Taberna P, Gogotsi Y, Simon P. High capacitance of surface-modified 2D titanium carbide in acidic electrolyte. Electrochem Commun 2014;48:118-22.
34. Chen C, Wang T, Zhao X, et al. Customizing hydrophilic terminations for V2CTx MXene toward superior hybrid-ion storage in aqueous zinc batteries. Adv Funct Mater 2024;34:2308508.
35. Naguib M, Kurtoglu M, Presser V, et al. Two-dimensional nanocrystals produced by exfoliation of Ti3AlC2. Adv Mater 2011;23:4248-53.
36. Pang SY, Wong YT, Yuan S, et al. Universal strategy for HF-free facile and rapid synthesis of two-dimensional MXenes as multifunctional energy materials. J Am Chem Soc 2019;141:9610-6.
37. Ghidiu M, Lukatskaya MR, Zhao MQ, Gogotsi Y, Barsoum MW. Conductive two-dimensional titanium carbide 'clay' with high volumetric capacitance. Nature 2014;516:78-81.
38. Wu M, He M, Hu Q, et al. Ti3C2 MXene-based sensors with high selectivity for NH3 detection at room temperature. ACS Sens 2019;4:2763-70.
39. Liu F, Zhou A, Chen J, et al. Preparation of Ti3C2 and Ti2C MXenes by fluoride salts etching and methane adsorptive properties. Appl Surf Sci 2017;416:781-9.
40. Liu D, Wang L, He Y, et al. Enhanced reversible capacity and cyclic performance of lithium-ion batteries using SnO2 interpenetrated MXene V2C architecture as anode materials. Energy Technol 2021;9:2000753.
41. Wang X, Garnero C, Rochard G, et al. A new etching environment (FeF3/HCl) for the synthesis of two-dimensional titanium carbide MXenes: a route towards selective reactivity vs. water. J Mater Chem A 2017;5:22012-23.
42. Urbankowski P, Anasori B, Makaryan T, et al. Synthesis of two-dimensional titanium nitride Ti4N3 (MXene). Nanoscale 2016;8:11385-91.
43. Natu V, Pai R, Sokol M, Carey M, Kalra V, Barsoum MW. 2D Ti3C2Tz MXene synthesized by water-free etching of Ti3AlC2 in polar organic solvents. Chem 2020;6:616-30.
44. Yang S, Zhang P, Wang F, et al. Fluoride-free synthesis of two-dimensional titanium carbide (MXene) using a binary aqueous system. Angew Chem Int Ed 2018;57:15491-5.
45. Li T, Yao L, Liu Q, et al. Fluorine-free synthesis of high-purity Ti3C2Tx (T=OH, O) via alkali treatment. Angew Chem Int Ed 2018;57:6115-9.
46. Li M, Lu J, Luo K, et al. Element replacement approach by reaction with lewis acidic molten salts to synthesize nanolaminated MAX phases and MXenes. J Am Chem Soc 2019;141:4730-7.
47. Jawaid A, Hassan A, Neher G, et al. Halogen etch of Ti3AlC2 MAX phase for MXene fabrication. ACS Nano 2021;15:2771-7.
48. Li J, Wang C, Yu Z, Chen Y, Wei L. MXenes for zinc-based electrochemical energy storage devices. Small 2023:e2304543.
49. Geng D, Zhao X, Chen Z, et al. Direct synthesis of large-area 2D Mo2C on in situ grown graphene. Adv Mater 2017;29:1702678.
50. Zhang F, Zhang Z, Wang H, et al. Plasma-enhanced pulsed-laser deposition of single-crystalline Mo2C ultrathin superconducting films. Phys Rev Mater 2017;1:034002.
51. Xiao X, Yu H, Jin H, et al. Salt-templated synthesis of 2D metallic MoN and other nitrides. ACS Nano 2017;11:2180-6.
52. Wang Q, Yuan H, Zhang M, et al. A highly conductive and supercapacitive MXene/N-CNT electrode material derived from a MXene-co-melamine precursor. ACS Appl Electron Mater 2023;5:2506-17.
53. Xu M, Lei S, Qi J, et al. Opening magnesium storage capability of two-dimensional MXene by intercalation of cationic surfactant. ACS Nano 2018;12:3733-40.
54. Miao Z, Zhang F, Zhao H, et al. Tailoring local electrolyte solvation structure via a mesoporous molecular sieve for dendrite-free zinc batteries. Adv Funct Mater 2022;32:2111635.
55. Kang Y, Zhang F, Li H, et al. Modulating the electrolyte inner solvation structure via low polarity co-solvent for low-temperature aqueous zinc-ion batteries. Energy Environ Mater 2024:e12707.
56. Du M, Zhang F, Zhang X, et al. Calcium ion pinned vanadium oxide cathode for high-capacity and long-life aqueous rechargeable zinc-ion batteries. Sci China Chem 2020;63:1767-76.
57. Miao Z, Du M, Li H, et al. Constructing nano-channeled tin layer on metal zinc for high-performance zinc-ion batteries anode. EcoMat 2021;3:e12125.
58. Wei W, Zhang F, Li H, et al. Modulating the solvation structure and electrode interface through phosphate additive for highly reversible zinc metal anode. Chem Eng J 2024;485:149944.
59. Miao Z, Liu Q, Wei W, et al. Unveiling unique steric effect of threonine additive for highly reversible Zn anode. Nano Energy 2022;97:107145.
60. Wang C, Xie H, Chen S, et al. Atomic cobalt covalently engineered interlayers for superior lithium-ion storage. Adv Mater 2018;30:e1802525.
61. Li M, Li X, Qin G, et al. Halogenated Ti3C2 MXenes with electrochemically active terminals for high-performance zinc ion batteries. ACS Nano 2021;15:1077-85.
62. Liu Y, Jiang Y, Hu Z, et al. In-situ electrochemically activated surface vanadium valence in V2C MXene to achieve high capacity and superior rate performance for Zn-ion batteries. Adv Funct Mater 2021;31:2008033.
63. Li X, Li M, Yang Q, et al. In situ electrochemical synthesis of MXenes without acid/alkali usage in/for an aqueous zinc ion battery. Adv Energy Mater 2020;10:2001791.
64. Guan J, Shao L, Yu L, et al. Two-dimensional Mg0.2V2O5·nH2O nanobelts derived from V4C3 MXenes for highly stable aqueous zinc ion batteries. Chem Eng J 2022;443:136502.
65. Zhu X, Wang W, Cao Z, et al. Zn2+-intercalated V2O5·nH2O derived from V2CTx MXene for hyper-stable zinc-ion storage. J Mater Chem A 2021;9:17994-8005.
66. Zhu X, Cao Z, Li X, et al. Ion-intercalation regulation of MXene-derived hydrated vanadates for high-rate and long-life Zn-Ion batteries. Energy Stor Mater 2022;45:568-77.
67. Sha D, Lu C, He W, et al. Surface selenization strategy for V2CTx MXene toward superior Zn-ion storage. ACS Nano 2022;16:2711-20.
68. Elia GA, Marquardt K, Hoeppner K, et al. An overview and future perspectives of aluminum batteries. Adv Mater 2016;28:7564-79.
69. Wang Y, Gu H, Lu Y, Zhang W, Li Z. The synergistic effect of Lewis acidic etching V4C3(MXene)@CuSe2/CoSe2 as an advanced cathode material for aluminum batteries. J Mater Sci Technol 2024;177:205-13.
70. Zhao S, Dall’agnese Y, Chu X, Zhao X, Gogotsi Y, Gao Y. Electrochemical interaction of Sn-containing MAX phase (Nb2SnC) with Li-ions. ACS Energy Lett 2019;4:2452-7.
71. Zhao MQ, Xie X, Ren CE, et al. Hollow MXene spheres and 3D macroporous MXene frameworks for Na-ion storage. Adv Mater 2017;29:1702410.
72. Wu Y, Sun Y, Zheng J, Rong J, Li H, Niu L. MXenes: advanced materials in potassium ion batteries. Chem Eng J 2021;404:126565.
73. Li J, Zeng F, El-Demellawi JK, et al. Nb2CTx MXene cathode for high-capacity rechargeable aluminum batteries with prolonged cycle lifetime. ACS Appl Mater Interfaces 2022;14:45254-62.
74. Wang L, Wang J, Ouyang B. Computational investigation of MAX as intercalation host for rechargeable aluminum-ion battery. Adv Energy Mater 2023;13:2302584.
75. VahidMohammadi A, Hadjikhani A, Shahbazmohamadi S, Beidaghi M. Two-dimensional vanadium carbide (MXene) as a high-capacity cathode material for rechargeable aluminum batteries. ACS Nano 2017;11:11135-44.
76. Yu X, Wang B, Gong D, Xu Z, Lu B. Graphene nanoribbons on highly porous 3D graphene for high-capacity and ultrastable Al-ion batteries. Adv Mater 2017;29:1604118.
77. Shen F, Sun Z, Zhao L, et al. Triggering the phase transition and capacity enhancement of Nb2O5 for fast-charging lithium-ion storage. J Mater Chem A 2021;9:14534-44.
78. Liu F, Liu Y, Zhao X, Liu X, Fan LZ. Pursuit of a high-capacity and long-life Mg-storage cathode by tailoring sandwich-structured MXene@carbon nanosphere composites. J Mater Chem A 2019;7:16712-9.
79. Zhu J, Shi R, Liu Y, et al. 3D interwoven MXene networks fabricated by the assistance of bacterial celluloses as high-performance cathode material for rechargeable magnesium battery. Appl Surf Sci 2020;528:146985.
80. Zhang Y, Li D, Li J, et al. Flexible TiVCTx MXene film for high-performance magnesium-ion storage device. J Colloid Interface Sci 2024;657:550-8.
81. Zhao X, Zhang F, Li H, et al. Dynamic heterostructure design of MnO2 for high-performance aqueous zinc-ion batteries. Energy Environ Sci 2024;17:3629-40.
82. Song M, Tan H, Chao D, Fan HJ. Recent advances in Zn-ion batteries. Adv Funct Mater 2018;28:1802564.
83. Xie M, Zhang X, Wang R, et al. Mn-O bond engineering mitigating Jahn-Teller effects of manganese oxide for aqueous zinc-ion battery applications. Chem Eng J 2024;494:152908.
84. Zhao Y, Zhang P, Liang J, et al. Uncovering sulfur doping effect in MnO2 nanosheets as an efficient cathode for aqueous zinc ion battery. Energy Stor Mater 2022;47:424-33.
85. Zhu X, Cao Z, Wang W, et al. Superior-performance aqueous zinc-ion batteries based on the in situ growth of MnO2 nanosheets on V2CTX MXene. ACS Nano 2021;15:2971-83.
86. Peng Q, Guo J, Zhang Q, et al. Unique lead adsorption behavior of activated hydroxyl group in two-dimensional titanium carbide. J Am Chem Soc 2014;136:4113-6.
87. Wang Y, Liu L, Wang Y, Qu J, Chen Y, Song J. Atomically coupled 2D MnO2/MXene superlattices for ultrastable and fast aqueous zinc-ion batteries. ACS Nano 2023;17:21761-70.
88. Shi M, Wang B, Chen C, Lang J, Yan C, Yan X. 3D high-density MXene@MnO2 microflowers for advanced aqueous zinc-ion batteries. J Mater Chem A 2020;8:24635-44.
89. Wu L, Mei Y, Liu Y, et al. Interfacial synthesis of strongly-coupled δ-MnO2/MXene heteronanosheets for stable zinc ion batteries with Zn2+-exclusive storage mechanism. Chem Eng J 2023;459:141662.
90. Shi M, Wang B, Shen Y, et al. 3D assembly of MXene-stabilized spinel ZnMn2O4 for highly durable aqueous zinc-ion batteries. Chem Eng J 2020;399:125627.
91. Du M, Miao Z, Li H, Sang Y, Liu H, Wang S. Strategies of structural and defect engineering for high-performance rechargeable aqueous zinc-ion batteries. J Mater Chem A 2021;9:19245-81.
92. Zhang F, Sun X, Du M, et al. Weaker interactions in Zn2+ and organic ion-pre-intercalated vanadium oxide toward highly reversible zinc-ion batteries. Energy Environ Mater 2021;4:620-30.
93. Du M, Liu C, Zhang F, et al. Tunable layered (Na,Mn)V8O20·nH2O cathode material for high-performance aqueous zinc ion batteries. Adv Sci 2020;7:2000083.
94. Dong W, Du M, Zhang F, et al. In situ electrochemical transformation reaction of ammonium-anchored heptavanadate cathode for long-life aqueous zinc-ion batteries. ACS Appl Mater Interfaces 2021;13:5034-43.
95. Zhang X, Xue F, Sun X, et al. High-capacity zinc vanadium oxides with long-term cyclability enabled by in-situ electrochemical oxidation as zinc-ion battery cathode. Chem Eng J 2022;445:136714.
96. Du M, Miao Z, Li H, et al. Oxygen-vacancy and phosphate coordination triggered strain engineering of vanadium oxide for high-performance aqueous zinc ion storage. Nano Energy 2021;89:106477.
97. Zhang F, Du M, Miao Z, et al. Oxygen vacancies and N-doping in organic-inorganic pre-intercalated vanadium oxide for high-performance aqueous zinc-ion batteries. InfoMat 2022;4:e12346.
98. Zhang F, Kang Y, Zhao X, et al. Boosting charge carrier transport by layer-stacked MnxV2O6/V2C heterostructures for wide-temperature zinc-ion batteries. Adv Funct Mater 2024:2402071.
99. Liu C, Xu W, Mei C, Li MC, Xu X, Wu Q. Highly stable H2V3O8/Mxene cathode for Zn-ion batteries with superior rate performance and long lifespan. Chem Eng J 2021;405:126737.
100. Liang P, Xu T, Zhu K, et al. Heterogeneous interface-boosted zinc storage of H2V3O8 nanowire/Ti3C2Tx MXene composite toward high-rate and long cycle lifespan aqueous zinc-ion batteries. Energy Stor Mater 2022;50:63-74.
101. Xiao B, Chen J, Hu C, et al. 2D dynamic heterogeneous interface coupling endowing extra Zn2+ storage. Adv Funct Mater 2023;33:2211679.
102. Liu H, Jiang L, Cao B, et al. Van der Waals interaction-driven self-assembly of V2O5 nanoplates and MXene for high-performing zinc-ion batteries by suppressing vanadium dissolution. ACS Nano 2022;16:14539-48.
103. Zheng J, Xu T, Xia G, Cui WG, Yang Y, Yu X. Water-stabilized vanadyl phosphate monohydrate ultrathin nanosheets toward high voltage Al-ion batteries. Small 2023;19:e2207619.
104. Hu P, Zhu T, Wang X, et al. Highly durable Na2V6O16·1.63H2O nanowire cathode for aqueous zinc-ion battery. Nano Lett 2018;18:1758-63.
105. Geng L, Lv G, Xing X, Guo J. Reversible electrochemical intercalation of aluminum in Mo6S8. Chem Mater 2015;27:4926-9.
106. Hu Y, Ye D, Luo B, et al. A binder-free and free-standing cobalt sulfide@carbon nanotube cathode material for aluminum-ion batteries. Adv Mater 2018;30:1703824.
107. Shuai H, Liu R, Li W, et al. Recent advances of transition metal sulfides/selenides cathodes for aqueous zinc-ion batteries. Adv Energy Mater 2023;13:2202992.
108. Xu M, Bai N, Li HX, Hu C, Qi J, Yan XB. Synthesis of MXene-supported layered MoS2 with enhanced electrochemical performance for Mg batteries. Chin Chem Lett 2018;29:1313-6.
109. Mao Y, Bai J, Lin S, et al. Two birds with one stone: V4C3 MXene synergistically promoted VS2 cathode and zinc anode for high-performance aqueous zinc-ion batteries. Small 2024;20:e2306615.
110. Zhang Y, Cao Z, Liu S, et al. Charge-enriched strategy based on MXene-based polypyrrole layers toward dendrite-free zinc metal anodes. Adv Energy Mater 2022;12:2103979.
111. Tian Y, An Y, Yang Y, Xu B. Robust nitrogen/selenium engineered MXene/ZnSe hierarchical multifunctional interfaces for dendrite-free zinc-metal batteries. Energy Stor Mater 2022;49:122-34.
112. Yao L, Ju S, Yu X. Rational surface engineering of MXene@N-doped hollow carbon dual-confined cobalt sulfides/selenides for advanced aluminum batteries. J Mater Chem A 2021;9:16878-88.
113. Ohno S, Zeier WG. Toward practical solid-state lithium-sulfur batteries: challenges and perspectives. ACC Mater Res 2021;2:869-80.
114. Sun R, Hu J, Shi X, et al. Water-soluble cross-linking functional binder for low-cost and high-performance lithium-sulfur batteries. Adv Funct Mater 2021;31:2104858.
115. Xiao Z, Li Z, Meng X, Wang R. MXene-engineered lithium-sulfur batteries. J Mater Chem A 2019;7:22730-43.
116. Kaland H, Håskjold Fagerli F, Hadler-Jacobsen J, et al. Performance study of MXene/carbon nanotube composites for current collector- and binder-free Mg-S batteries. ChemSusChem 2021;14:1864-73.
117. Xu H, Zhu D, Zhu W, et al. Rational design of high concentration electrolytes and MXene-based sulfur host materials toward high-performance magnesium sulfur batteries. Chem Eng J 2022;428:131031.
118. Cheng Z, Xu Y, Zhang X, et al. An interfacial covalent bonding coupled ultrafine CuS-nanocrystals/MXene heterostructure for efficient and durable magnesium storage. J Mater Chem A 2023;11:12176-84.
119. Zhu J, Zhang X, Gao H, et al. VS4 anchored on Ti3C2 MXene as a high-performance cathode material for magnesium ion battery. J Power Sources 2022;518:230731.
120. Xu N, Wu KH, Miao QS, Zhou XM, Sheng LZ. Application of metal selenide anode materials in sodium-ion batteries. J Changsha Univ Sci Technol 2024;21:1-11.
121. Narayanasamy M, Hu L, Kirubasankar B, Liu Z, Angaiah S, Yan C. Nanohybrid engineering of the vertically confined marigold structure of rGO-VSe2 as an advanced cathode material for aqueous zinc-ion battery. J Alloy Compd 2021;882:160704.
122. Lv W, Wu G, Li X, Li J, Li Z. Two-dimensional V2C@Se (MXene) composite cathode material for high-performance rechargeable aluminum batteries. Energy Stor Mater 2022;46:138-46.
123. Li Z, Wang X, Zhang W, Yang S. Two-dimensional Ti3C2@CTAB-Se (MXene) composite cathode material for high-performance rechargeable aluminum batteries. Chem Eng J 2020;398:125679.
124. Yuan Z, Lin Q, Li Y, Han W, Wang L. Effects of multiple ion reactions based on a CoSe2/MXene cathode in aluminum-ion batteries. Adv Mater 2023;35:e2211527.
125. Liu F, Wang T, Liu X, Jiang N, Fan LZ. High-performance heterojunction Ti3C2/CoSe2 with both intercalation and conversion storage mechanisms for magnesium batteries. Chem Eng J 2021;426:130747.
126. Zhang Y, Cao JM, Yuan Z, et al. TiVCTx MXene/chalcogenide heterostructure-based high-performance magnesium-ion battery as flexible integrated units. Small 2022;18:e2202313.
127. Zhang Y, Cao J, Li J, et al. Self-assembled cobalt-doped NiMn-layered double hydroxide (LDH)/V2CTx MXene hybrids for advanced aqueous electrochemical energy storage properties. Chem Eng J 2022;430:132992.
128. Pan H, Li B, Mei D, et al. Controlling solid-liquid conversion reactions for a highly reversible aqueous zinc-iodine battery. ACS Energy Lett 2017;2:2674-80.
129. Li X, Li N, Huang Z, et al. Enhanced redox kinetics and duration of aqueous I2/I- conversion chemistry by MXene confinement. Adv Mater 2021;33:e2006897.
130. Wang X, Liu Y, Wei Z, et al. MXene-boosted imine cathodes with extended conjugated structure for aqueous zinc-ion batteries. Adv Mater 2022;34:e2206812.
131. Tie Z, Liu L, Deng S, Zhao D, Niu Z. Proton insertion chemistry of a zinc-organic battery. Angew Chem Int Ed 2020;59:4920-4.
132. Na M, Oh Y, Byon HR. Effects of Zn2+ and H+ association with naphthalene diimide electrodes for aqueous Zn-ion batteries. Chem Mater 2020;32:6990-7.
133. Nam KW, Park SS, Dos Reis R, et al. Conductive 2D metal-organic framework for high-performance cathodes in aqueous rechargeable zinc batteries. Nat Commun 2019;10:4948.
134. Wang Y, Song J, Wong WY. 3D nanostructured conductive PANI/MXene hydrogels for durable aqueous Zn-ion batteries. J Mater Chem A 2024;12:943-9.
135. Wu G, Lv C, Lv W, Li X, Zhang W, Li Z. Anthraquinone derivatives supported by Ti3C2(MXene) as cathode materials for aluminum-organic batteries. J Energy Chem 2022;74:174-83.
Cite This Article

How to Cite
Download Citation
Export Citation File:
Type of Import
Tips on Downloading Citation
Citation Manager File Format
Type of Import
Direct Import: When the Direct Import option is selected (the default state), a dialogue box will give you the option to Save or Open the downloaded citation data. Choosing Open will either launch your citation manager or give you a choice of applications with which to use the metadata. The Save option saves the file locally for later use.
Indirect Import: When the Indirect Import option is selected, the metadata is displayed and may be copied and pasted as needed.
About This Article
Copyright
Data & Comments
Data
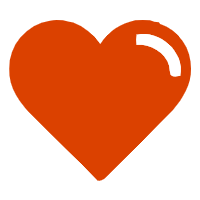
Comments
Comments must be written in English. Spam, offensive content, impersonation, and private information will not be permitted. If any comment is reported and identified as inappropriate content by OAE staff, the comment will be removed without notice. If you have any queries or need any help, please contact us at [email protected].