Polysulfide barrier comprising bismuth selenide nanocrystals well anchored within N-doped carbon microspheres for stable Li-S batteries
Abstract
The development of functional interlayers to effectively anchor lithium polysulfide and enhance the integrity of sulfur cathodes in lithium-sulfur (Li-S) batteries has received significant global consideration. However, identifying an interlayer that is both highly conductive and structurally robust remains a major challenge. This study presents the synthesis of three-dimensional nitrogen-doped carbon microspheres embedded with bismuth selenide nanocrystals (referred to as “three-dimensional (3D) Bi2Se3@N-C” microspheres) and evaluates their role as a polysulfide barrier for enhanced Li-S battery performance. The embedded Bi2Se3 nanocrystals within the microspheres provide numerous active spots for chemical captivity and electrocatalytic transformation of lithium polysulfide species. Moreover, the N-doped carbon framework facilitates speedy transfer of charge moieties, resulting in faster redox activity. Correspondingly, cells paired with 3D Bi2Se3@N-C microsphere modified separators exhibit excellent rate capability (297 mA h g-1 at 2.0 C-rate) and prolonged stable cycling performance at different
Keywords
INTRODUCTION
Lithium-sulfur batteries (LSBs) have garnered significant attention due to their high energy density (calculated at 2,600 Wh kg-1), plentiful sulfur (S) resources, and low cost[1-3]. Sulfur enables a multiple-electron (2e-) redox activity, offering a high capacity (theoretical value of 1,675 mA h g-1 at an average potential of approximately 2.2 V versus Li/Li+[4,5]. However, their commercialization is hindered by several challenges, including the poor electrical conductivity of sulfur (5 × 10-28 S m-1) and lithium sulfide (Li2Sx;
In this context, our group and others have demonstrated that separators functionalized with metal selenides exhibit promising electrochemical performance in LSBs, owing to their excellent electrocatalytic activity towards LiPS[20-23]. Despite the extensive exploration of chalcogenides, one particularly intriguing candidate has been largely overlooked: bismuth selenide (Bi2Se3). This material belongs to the A2VB3VI class (A = Sb, Bi; B = Se, Te) of degenerated n-type semiconductor material, characterized by a low bandgap of 0.3 eV[24-26]. While it has attracted significant interest in fields such as thermoelectric and optoelectronic devices, its potential application in LSBs remains unexplored[27]. The n-type electronic behavior of Bi2Se3 arises from Se deficiencies, which behave similarly to electron donors. Bi2Se3 features a layered structure composed of covalently stacked Se-Bi-Se-Bi-Se atomic layers. This unique structure, combined with its high electrical conductivity and mutable valences of Bi and Se, offers a significant catalytic perspective.
Here, we disclose the synthesis of three-dimensional (3D) nitrogen-doped carbon microspheres embedded with bismuth selenide nanocrystals (3D Bi2Se3@N-C microspheres) using a scalable spray pyrolysis procedure trailed by a selenization process. The potential of these prepared microspheres as an effective polysulfide barrier to enhance reaction kinetics in Li-S batteries is systematically evaluated. The embedded Bi2Se3 nanocrystals within the microspheres provide abundant chemisorption sites, enabling efficient chemical captivity and transformation of LiPS species. Moreover, the N-doped carbon framework ensures rapid charge transfer, facilitating faster redox processes. Electrochemical evaluations reveal that
EXPERIMENTAL
Synthesis of 3D Bi2Se3@N-C microspheres
Synthesis of 3D nitrogen-doped carbon (N-C) microspheres comprising well-grafted bismuth selenide nanocrystals (denoted as “3D Bi2Se3@N-C” microspheres) is achieved using an easily scalable spray pyrolysis technique assisted with a selenization process and applied as a multifunctional polysulfide barrier facing towards the sulfur cathode. Initially, spray solution was prepared using 0.025 M (2.42 g) of bismuth nitrate pentahydrate [Bi(NO3)3·5H2O, Junsei Chemical Co., Ltd. (JUNSEI), Mw = 485.07] dispersed in a blend of distilled water (100 mL) and nitric acid (15 mL, HNO3, JUNSEI, Mw = 63.01), resulted in a transparent solution. To the above solution, 0.05 M (1.71 g) of sucrose [C12H22O11, DAEJUNG Chemicals Metals (DAEJUNG), Mw = 342.3] as a carbon source and 1.5 g of polyvinylpyrrolidone (PVP, Mw = 40,000, DAEJUNG) as a nitrogen source were also added. The solution was allowed to stir gently overnight at ambient conditions. The obtained solution was transferred to an ultrasonic atomic nebulizer coupled with a vertically positioned quartz tube reactor. The ultrasonic nebulizer generates aqueous droplets which are subsequently passed through the preheated quartz tube reactor operated at 400 °C. A constant gas flow (N2, 20 L min-1) was maintained throughout the process. The as-sprayed powder was collected carefully and further heat-treated (selenization) at 350 °C for 6 h in a slightly reductive atmosphere (4% of H2/Ar) at a ramp rate of 5 °C min-1. Excess selenium powder (Se, 99.5%, SAMCHUN, 200 mesh) was used for complete selenization of the as-sprayed powder through the
RESULTS AND DISCUSSION
To validate the successful synthesis of 3D Bi2Se3@N-C microspheres, detailed physical characterizations were conducted at each step of the synthesis process. Field emission scanning electron microscopy
Figure 1. (A) FE-SEM micrograph; (B) XRD profile; (C) TEM picture; and (D) HR-TEM image of the 3D Bi2Se3@N-C microspheres.
The as-sprayed Bi-salt/PVP/Sucrose composite microspheres were subjected to a selenization process under a mildly reductive atmosphere (4% of H2/Ar) at 350 °C for 6 h. The physical characterization results of the resulting microspheres are presented in Figure 1. The FE-SEM micrograph [Figure 1A] discloses that the spherical morphology of the selenized microspheres was retained (average diameter = 1.1 µm) even after the selenization process. Furthermore, the high-magnification FE-SEM image [inset of Figure 1A] reveals significant changes in surface characteristics. Unlike the smooth surface of the as-sprayed sample, the selenized microspheres exhibit a rougher texture, with the surface covered by well-formed nanocrystals, likely Bi2Se3. This observation aligns with the XRD analysis shown in Figure 1B, where sharp, distinct diffraction peaks correspond to the rhombohedral crystal structure of Bi2Se3 with a R
Based on the discussion above, Scheme 1 illustrates the detailed formation mechanism of the
Scheme 1. Representation of the detailed synthesis route for 3D Bi2Se3@N-C microspheres using spray pyrolysis technique (①-③) and their utilization as multifunctional polysulfide barrier (④). 3D: Three-dimensional.
The surface composition and bonding states within the 3D Bi2Se3@N-C microspheres were assessed via
Figure 2. (A) XPS survey; (B) deconvoluted Bi 4f spectrum; (C) deconvoluted Se 3d spectrum; (D) deconvoluted C 1s spectrum; (E) deconvoluted N 1s spectrum; and (F) TG profile of 3D Bi2Se3@N-C microspheres in an air atmosphere. XPS: X-ray photoelectron spectroscopy, TG: thermogravimetric, 3D: three-dimensional.
Before evaluating the electrochemical properties of the assembled Li-S cells, the prepared multifunctional barrier and the pristine separator were subjected to physical analysis and integrity tests. Supplementary Figure 5A demonstrates a digital image of the coated separator, which appears uniform, continuous, and free from cracks. The coated separator was sliced into round disks (ϕ = 19 mm), as displayed in Supplementary Figure 5B. The thickness of the coated functional separator [Supplementary Figure 5C] and the pristine separator [Supplementary Figure 5D] was measured using a digital thickness meter, revealing a coating layer approximately 5 µm thick. Besides, the mechanical robustness of the modified separator was evaluated through folding and bending tests, as shown in Supplementary Figure 5E. Remarkably, the coated separator regained its original shape after these tests, demonstrating excellent mechanical robustness. The active loading mass of the coated separator was determined to be 5.2 mg [Supplementary Figure 5F], corresponding to a surface mass density of approximately 0.4 mg cm-2. The FE-SEM micrograph of the uncoated Celgard 2400 separator, presented in Supplementary Figure 6A, reveals interconnected submicron-sized porous openings. These openings facilitate the uninterrupted transport of Li+ ions during redox reactions and enable efficient electrolyte percolation. Conversely, the FE-SEM micrograph of the functional polysulfide barrier coated with 3D Bi2Se3@N-C microsphere [Supplementary Figure 6B] displays a dense and uniform coating of the microspheres. Additionally, the thickness of the coating layer was observed to be approximately 5 µm, as confirmed by Supplementary Figure 6C, which aligns well with the values obtained from the digital thickness measurements. Furthermore, the coated separator was subjected to wettability tests, as shown in Supplementary Figure 7. As observed, a smaller contact angle [15.8°, Supplementary Figure 7A] for the coated separator compared to the pristine sample [39.7°, Supplementary Figure 7B] implies better wettability. Moreover, it was observed that the electrolyte absorption capability of the coated separator is approximately 63% higher than that of the pristine separator, as evident from the digital images presented in Supplementary Figure 7C-F, which clearly indicates a higher weight of the coated separator after soaking for a predetermined time in the electrolyte.
The as-prepared 3D Bi2Se3@N-C microspheres were engaged as a polysulfide barrier by coating them onto a commercial Celgard separator, positioned to face the regular sulfur cathode. The assembled CR2032 coin cells were initially subjected to cyclic voltammetry (CV) tests at a scan rate of 0.1 mV s-1, and the first scans are presented in Figure 3A. For the cell incorporating the 3D Bi2Se3@N-C coated separator, the CV scan reveals two sharp and intense reduction peaks, labeled R1 and R2, centered at 2.31 V and 2.0 V, respectively. In contrast, the cell paired with a pristine separator exhibits broader and less intense reduction peaks located at 2.20 V and 1.96 V, respectively. Notably, the R1 peak corresponds to the reduction process to form high-order LiPSs (Li2S8 or Li2S6), which are further converted into middle-order polysulfides. The R2 peak signifies the subsequent reduction to form insoluble Li2S species[44,45]. During the reverse scan, two closely spaced oxidation peaks are observed for the 3D Bi2Se3@N-C coated separator, representing the reverse mechanism. These peaks correspond to the oxidation of Li2S to Li2S6 or Li2S8 at 2.38 V and further to elemental sulfur at around 2.44 V, completing the redox process[46,47]. Conversely, the cell with the pristine separator displays two unresolved oxidation peaks at significantly higher voltage values, 2.49 V and 2.52 V. It is noteworthy to mention that the cell utilizing the 3D Bi2Se3@N-C modified separator demonstrates the maximum redox current values, signifying enriched redox activities. This observation aligns with the individual peak voltage values reflected in Supplementary Figure 8A and respective voltage polarization values (the difference in voltage between the O and R2 peaks). Notably, the cell featuring the
Figure 3. (A) CV profiles of cells paired with coated/pristine separator at 0.1 mV s-1; (B) first three CV scans for cell paired with 3D Bi2Se3@N-C coated separator; (C) initial Galvanostatic charge-discharge voltage profile comparison at 0.05 C; (D) charge-discharge voltage profiles at different C-rates with 3D Bi2Se3@N-C coated separator; (E) rate performance at various C-rates; and (F) capacity utilization values for 3D Bi2Se3@N-C coated separator. CV: Cyclic voltammetry; 3D: three-dimensional.
To validate the CV results, the initial Galvanostatic charge-discharge (GCD) characteristics were examined at 0.05 C, as shown in Figure 3C. The voltage profiles for both the separator configurations exhibit similar shapes, with two distinct reduction voltage regions and a sloppy charging plateau, reflecting the multistep electrochemical processes. This observation aligns well with CV results. However, differences in the extent of the redox plateaus suggest varying extents of redox reactions within the cells. Notably, the cell incorporating the 3D Bi2Se3@N-C-modified separator demonstrates the lengthiest redox plateau and the lowermost polarization (ΔE). The ΔE values, presented in Supplementary Figure 8B, reveal that the
Rate capability tests were conducted at various current values ranging from 0.05-2.0 C, as shown in Figure 3D and E. The GCD curves at different C-rates for cell paired with 3D Bi2Se3@N-C coated separator exhibit well-defined charge-discharge redox plateaus even at 2.0 C-rate, highlighting superior performance compared to the cell with the pristine separator [Supplementary Figure 10]. For the 3D Bi2Se3@N-C modified separator, initial capacities of 1134 (0.05 C), 936 (0.1 C), 780 (0.2 C), 650 (0.3 C), 545 (0.5 C),
Stable cycling performance is a critical factor for the commercial viability of the prepared nanostructure. Figure 4A-C illustrates the cycling performance tests of the 3D Bi2Se3@N-C coated separator compared to the pristine separator. At a 0.1 C-rate [Figure 4A], 3D Bi2Se3@N-C-modified separator exhibits an early discharge capacity of 1,039 mA h g-1 and retains 83% (863 mA h g-1) after 100 continuous charge-discharge cycles, corresponding to an average capacity decay rate of just 0.16% per cycle. Moreover, the high Columbic efficiency (~99%) indicates highly reversible redox processes. Conversely, the pristine separator shows a significantly lower initial capacity of 510 mA h g-1 (30% of theoretical) and retains only 379 mA h g-1 (74% retention) after 100 cycles, corresponding to a higher capacity decay rate of 0.27% per cycle. Similar trends are observed at higher C-rates. For example, at 0.5 C [Figure 4B], a 3D Bi2Se3@N-C-coated separator achieves a discharge capacity of 440 mA h g-1 after 200 cycles, compared to just 229 mA h g-1 for the pristine separator cell. At an even higher C-rate of 2.0 C [Figure 4C], the discharge capacities at the end of the 500th cycle are 219 mA h g-1 for the 3D Bi2Se3@N-C coated separator and only 62 mA h g-1 for the pristine separator. The excellent capacity retention, even after prolonged cycling, underscores the structural merits of the prepared sample. To further access practical viability, cells were assembled with high S-loading electrodes (2.5 and 3.5 mg cm-2). The cycling performance results at 0.3 C-rate are presented in Figure 4D. Even at high sulfur loadings, the cells demonstrate stable cycling up to 200 cycles. For instance, with a sulfur loading of 2.5 mg cm-2, the cell achieves an initial discharge capacity of 579 mA h g-1 and retains 58%
Figure 4. Cycling tests of cells featuring coated/pristine separators at (A) 0.1 C-rate; (B) 0.5 C-rate; (C) 2.0 C-rate; (D) cycling test of 3D Bi2Se3@N-C at 0.3 C coupled with high loading electrodes; and (E) detailed working mechanism of the 3D Bi2Se3@N-C modified separator. 3D: Three-dimensional.
The enhanced redox kinetics of the 3D Bi2Se3@N-C coated separator were analyzed by determining the diffusion coefficient (
Figure 5.
where Ip represents the redox peak current, n is the number of electrons involved, A is the electrode surface area (cm2), CLi is the concentration (mol L-1) of Li-ion, and v is the voltage scan rate (Vs-1). The CV curves for cell featuring the 3D Bi2Se3@N-C coated separator [Figure 5A] exhibit two distinct reduction peaks
Overall, the enhancement in electrochemical properties of cells retaining 3D Bi2Se3@N-C microspheres as a multifunctional polysulfide barrier firmly validates the structural supremacy of the prepared nanostructures that resulted in improved redox reaction kinetics via fast charge transfer characteristics, suppress polysulfide diffusion, and enhance active sulfur participation during electrochemical processes.
CONCLUSIONS
In conclusion, this work presents a comprehensive study on the synthesis of 3D Bi2Se3@N-C microspheres and their application as a multifunctional polysulfide barrier for improved LSB properties such as realistic rate capability and steady cycling performance across a range of C-rates, from low (0.1 C and 0.5 C) to high (2.0 C). The incorporation of Bi2Se3 nanocrystals ensures efficient anchoring and transformation of polysulfide, enhancing sulfur participation in electrochemical reactions. Furthermore, N-doping within the carbon framework expedites rapid charge transferal, accelerating redox activity. As a result, the cell equipped with a 3D Bi2Se3@N-C microsphere coated separator delivered remarkable performance, including high rate properties (297 mA h g-1 at 2.0 C-rate) and prolonged stable cycling performance at different
DECLARATIONS
Authors’ contributions
Substantial contributions to the conception and design of the study, data analysis and interpretation: Saroha, R.
Substantial contributions to the conception and design of the study, data interpretation, and blueprint preparation: Lee, J. S.
Data acquisition: Cho, S. W.
Administrative and material support, writing-review and editing: Cho, C.
Technical and material support, writing and review: Park, J. S.
Supervision of the work, administrative, technical, and material support, writing-review and editing:
Availability of data and materials
The rata data supporting the findings of this study are available within this Article and its Supplementary Materials file.
Financial support and sponsorship
This work was supported by the National Research Foundation of Korea (NRF) grant funded by the Korea government (MSIT) (Grant No. RS-2023-00217581, RS-2025-00556955). It was also supported by the Technology Development Program (RS-2023-00263878) funded by the Ministry of SMEs and Startups (MSS, Korea). Additionally, this research was supported by the Global - Learning & Academic Research Institution for Master’s, PhD students, and Postdocs (G-LAMP) Program of the NRF grant funded by the Ministry of Education (No. RS-2023-00285390).
Conflicts of interest
All authors declared that there are no conflicts of interest.
Ethical approval and consent to participate
Not applicable.
Consent for publication
Not applicable.
Copyright
© The Author(s) 2025.
Supplementary Materials
REFERENCES
1. Manthiram, A.; Fu, Y.; Su, Y. S. Challenges and prospects of lithium-sulfur batteries. Acc. Chem. Res. 2013, 46, 1125-34.
2. Zhou, L.; Li, H.; Wu, X.; et al. Double-shelled Co3O4/C nanocages enabling polysulfides adsorption for high-performance lithium-sulfur batteries. ACS. Appl. Energy. Mater. 2019, 2, 8153-62.
3. Zhao, M.; Li, B. Q.; Zhang, X. Q.; Huang, J. Q.; Zhang, Q. A perspective toward practical lithium-sulfur batteries. ACS. Cent. Sci. 2020, 6, 1095-104.
4. Wang, Z.; Wang, B.; Yang, Y.; et al. Mixed-metal-organic framework with effective lewis acidic sites for sulfur confinement in high-performance lithium-sulfur batteries. ACS. Appl. Mater. Interfaces. 2015, 7, 20999-1004.
5. Wang, P.; Zeng, R.; You, L.; et al. Graphene-like matrix composites with Fe2O 3 and Co3O4 as cathode materials for lithium-sulfur batteries. ACS. Appl. Nano. Mater. 2020, 3, 1382-90.
6. Pope, M. A.; Aksay, I. A. Structural design of cathodes for Li-S batteries. Adv. Energy. Mater. 2015, 5, 1500124.
7. Kim, J.; Lee, D.; Jung, H.; Sun, Y.; Hassoun, J.; Scrosati, B. An advanced lithium-sulfur battery. Adv. Funct. Mater. 2013, 23, 1076-80.
8. He, G.; Evers, S.; Liang, X.; Cuisinier, M.; Garsuch, A.; Nazar, L. F. Tailoring porosity in carbon nanospheres for lithium-sulfur battery cathodes. ACS. Nano. 2013, 7, 10920-30.
9. Li, Z.; Jiang, Y.; Yuan, L.; et al. A highly ordered meso@microporous carbon-supported sulfur@smaller sulfur core-shell structured cathode for Li-S batteries. ACS. Nano. 2014, 8, 9295-303.
10. Sun, L.; Wang, D.; Luo, Y.; et al. Sulfur embedded in a mesoporous carbon nanotube network as a binder-free electrode for high-performance lithium-sulfur batteries. ACS. Nano. 2016, 10, 1300-8.
11. Chulliyote, R.; Hareendrakrishnakumar, H.; Joseph, M. G. Hierarchical porous carbon material with multifunctionalities derived from honeycomb as a sulfur host and laminate on the cathode for high-performance lithium-sulfur batteries. ACS. Sustainable. Chem. Eng. 2019, 7, 19344-55.
12. Zhang, F.; Li, Z.; Cao, T.; et al. Multishelled Ni2P microspheres as multifunctional sulfur host 3D-printed cathode materials ensuring high areal capacity of lithium-sulfur batteries. ACS. Sustainable. Chem. Eng. 2021, 9, 6097-106.
13. Park, J.; Yu, B. C.; Park, J. S.; et al. Tungsten disulfide catalysts supported on a carbon cloth interlayer for high performance Li-S battery. Adv. Energy. Mater. 2017, 7, 1602567.
14. Liu, Z.; Sun, L.; Liu, X.; Lu, Q. Stabilization strategies of lithium metal anode toward dendrite-free lithium-sulfur batteries. Chem. Eur. J. 2024, 30, e202402032.
15. Song, B.; Su, L.; Liu, X.; et al. An examination and prospect of stabilizing Li metal anode in lithium-sulfur batteries: a review of latest progress. Electron 2023, 1, e13.
16. Song, J.; Xu, T.; Gordin, M. L.; et al. Nitrogen-doped mesoporous carbon promoted chemical adsorption of sulfur and fabrication of high-areal-capacity sulfur cathode with exceptional cycling stability for lithium-sulfur batteries. Adv. Funct. Mater. 2014, 24, 1243-50.
17. Chung, S.; Manthiram, A. Bifunctional separator with a light-weight carbon-coating for dynamically and statically stable lithium-sulfur batteries. Adv. Funct. Mater. 2014, 24, 5299-306.
18. Xu, R.; Lu, J.; Amine, K. progress in mechanistic understanding and characterization techniques of Li-S batteries. Adv. Energy. Mater. 2015, 5, 1500408.
19. Liu, Y.; Elias, Y.; Meng, J.; et al. Electrolyte solutions design for lithium-sulfur batteries. Joule 2021, 5, 2323-64.
20. Saroha, R.; Choi, H. H.; Cho, J. S. Boosting redox kinetics using rationally engineered cathodic interlayers comprising porous rGO-CNT framework microspheres with NiSe2-core@N-doped graphitic carbon shell nanocrystals for stable Li-S batteries. Chem. Eng. J. 2023, 473, 145391.
21. Saroha, R.; Ka, H. S.; Park, G. D.; Cho, C.; Kang, D.; Cho, J. S. Long-term stability of lithium-sulfur batteries via synergistic integration of nitrogen-doped graphitic carbon-coated cobalt selenide nanocrystals within porous three-dimensional graphene-carbon nanotube microspheres. J. Power. Sources. 2024, 592, 233893.
22. Wang, L.; Meng, X.; Wang, X.; Zhen, M. Dual-conductive CoSe2@TiSe2 -C heterostructures promoting overall sulfur redox kinetics under high sulfur loading and lean electrolyte. Small 2023, 19, 2300089.
23. Lu, J.; Luo, S.; Qi, Z.; et al. Recent advances in transition metal chalcogenide derivatives from metal-organic frameworks for lithium-sulfur batteries. Cell. Rep. Phys. Sci. 2024, 5, 102028.
24. Shirodkar, S. N.; Dev, P. Nonlinear hybrid surface-defect states in defective Bi2Se3. J. Phys. Chem. C. 2022, 126, 11833-9.
25. Zhang, H.; Liu, C.; Qi, X.; Dai, X.; Fang, Z.; Zhang, S. Topological insulators in Bi2Se3, Bi2Te3 and Sb2Te3 with a single Dirac cone on the surface. Nature. Phys. 2009, 5, 438-42.
26. Xia, Y.; Qian, D.; Hsieh, D.; et al. Observation of a large-gap topological-insulator class with a single Dirac cone on the surface. Nature. Phys. 2009, 5, 398-402.
27. Hu, L.; Zhu, T.; Liu, X.; Zhao, X. Point defect engineering of high-performance bismuth-telluride-based thermoelectric materials. Adv. Funct. Mater. 2014, 24, 5211-8.
28. Singh, S.; Sahoo, R. K.; Shinde, N. M.; et al. Asymmetric faradaic assembly of Bi2O3 and MnO2 for a high-performance hybrid electrochemical energy storage device. RSC. Adv. 2019, 9, 32154-64.
29. Wang, K.; Shao, C.; Li, X.; Miao, F.; Lu, N.; Liu, Y. Heterojunctions of p-BiOI nanosheets/n-TiO2 nanofibers: preparation and enhanced visible-light photocatalytic activity. Materials 2016, 9, 90.
30. El-makaty, F. M.; Nawaz, M.; Shakoor, R.; Hammuda, A.; Youssef, K. M. Microstructural effect on the corrosion behavior of n- and p-type bismuth tellurides fabricated by induction melting. Mater. Charact. 2023, 202, 112987.
31. Cho, W. S.; Hong, D. M.; Dong, W. J.; et al. Porously reduced 2-dimensional Bi2O2CO3 petals for strain-mediated electrochemical CO2 reduction to HCOOH. Energy. Environ. Mater. 2024, 7, e12490.
32. Abzal, S. M.; Khatua, S.; Kalyan, K.; et al. Exploring the electrochemical performance of layered Bi2Se3 hexagonal platelets as the anode material for lithium-ion batteries. Phys. Chem. Chem. Phys. 2024, 26, 25418-29.
33. Gao, L.; Li, H.; Ren, W.; et al. Patterning Bi2Se3 single-crystalline thin films on Si(111) substrates using strong oxidizing acids. RSC. Adv. 2017, 7, 32294-9.
34. Edmonds, M. T.; Hellerstedt, J. T.; Tadich, A.; et al. Stability and surface reconstruction of topological insulator Bi2Se3 on exposure to atmosphere. J. Phys. Chem. C. 2014, 118, 20413-9.
35. Kong, D.; Cha, J. J.; Lai, K.; et al. Rapid surface oxidation as a source of surface degradation factor for Bi2Se3. ACS. Nano. 2011, 5, 4698-703.
36. Dharmadhikari, V. S.; Sainkar, S.; Badrinarayan, S.; Goswami, A. Characterisation of thin films of bismuth oxide by X-ray photoelectron spectroscopy. J. Electron. Spectrosc. Relat. Phenom. 1982, 25, 181-9.
37. Wu, J.; Zhao, J.; Vaidhyanathan, B.; et al. Rapid microwave-assisted bulk production of high-quality reduced graphene oxide for lithium ion batteries. Materialia 2020, 13, 100833.
38. Varodi, C.; Pogăcean, F.; Cioriță, A.; et al. Nitrogen and sulfur co-doped graphene as efficient electrode material for L-cysteine detection. Chemosensors 2021, 9, 146.
39. Begum, H.; Ahmed, M. S.; Jeon, S. New approach for porous chitosan-graphene matrix preparation through enhanced amidation for synergic detection of dopamine and uric acid. ACS. Omega. 2017, 2, 3043-54.
40. Ismagilov, Z. R.; Shalagina, A. E.; Podyacheva, O. Y.; et al. Structure and electrical conductivity of nitrogen-doped carbon nanofibers. Carbon 2009, 47, 1922-9.
41. Sun, H.; Xiao, M.; Zhu, F. Nitrogen doped porous carbon with high rate performance for lithium ion storage. J. Electroanal. Chem. 2023, 932, 117254.
42. Nulu, A.; Nulu, V.; Sohn, K. Y. N-doped CNTs wrapped sulfur-loaded hierarchical porous carbon cathode for Li-sulfur battery studies. RSC. Adv. 2024, 14, 2564-76.
43. Lee, J. Y.; Kim, N. Y.; Shin, D. Y.; et al. Nitrogen-doped graphene-wrapped iron nanofragments for high-performance oxygen reduction electrocatalysts. J. Nanopart. Res. 2017, 19, 98.
44. Li, Y.; Jiang, T.; Yang, H.; et al. A heterostuctured Co3S4/MnS nanotube array as a catalytic sulfur host for lithium-sulfur batteries. Electrochim. Acta. 2020, 330, 135311.
45. Tang, H.; Yao, S.; Xue, S.; et al. In-situ synthesis of carbon@Ti4O7 non-woven fabric as a multi-functional interlayer for excellent lithium-sulfur battery. Electrochim. Acta. 2018, 263, 158-67.
46. Yu, R.; Chung, S.; Chen, C.; Manthiram, A. An ant-nest-like cathode substrate for lithium-sulfur batteries with practical cell fabrication parameters. Energy. Storage. Mater. 2019, 18, 491-9.
47. Xiang, Y.; Li, J.; Lei, J.; et al. Advanced Separators for lithium-ion and lithium-sulfur batteries: a review of recent progress. ChemSusChem 2016, 9, 3023-39.
48. Kim, G.; Lee, Y.; Park, J.; et al. Enhanced performance of lithium-sulfur battery cathode via composition optimization using modified MWCNTs as a conductive material and poly (acrylic acid) as a binder. Int. J. Electrochem. Sci. 2023, 18, 100217.
Cite This Article
How to Cite
Download Citation
Export Citation File:
Type of Import
Tips on Downloading Citation
Citation Manager File Format
Type of Import
Direct Import: When the Direct Import option is selected (the default state), a dialogue box will give you the option to Save or Open the downloaded citation data. Choosing Open will either launch your citation manager or give you a choice of applications with which to use the metadata. The Save option saves the file locally for later use.
Indirect Import: When the Indirect Import option is selected, the metadata is displayed and may be copied and pasted as needed.
About This Article
Copyright
Data & Comments
Data
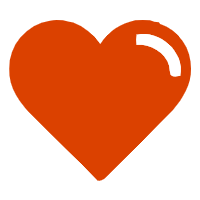
Comments
Comments must be written in English. Spam, offensive content, impersonation, and private information will not be permitted. If any comment is reported and identified as inappropriate content by OAE staff, the comment will be removed without notice. If you have any queries or need any help, please contact us at [email protected].