Conductivity boosted BiVO4 for enhanced OER and supercapacitive performance: stability insights with modeling, predictions, and forecasting using machine learning technique
Abstract
To overcome the inherent limitations in the energy generation and storage properties of transition metal-based catalysts, it is crucial to develop processes that produce catalytic materials with high performance and long-lasting effectiveness. Herein, we synthesized Metal-Organic Framework (MOF)-derived BiVO4 by mixing two separately prepared MOFs of Bi and V using trimesic acid and terephthalic acid as linkers. The separately prepared monometallic MOFs were then mixed and carbonized in an inert atmosphere followed by oxidation in air which gives the sample BiVO4 with carbon (BVC). The prepared BVC electrode showed the overpotential 364 mV for oxygen evolution reaction at the current density of 10 mA cm-2. In addition, the obtained BVC supercapacitor possesses a high specific capacity of 134.17 mAh g-1 (483 C g-1) at 1 mA cm-2 current density. The aqueous and solid-state symmetric supercapacitor devices were also fabricated and achieved specific capacitance of 160.9 F g-1 and 109.8 F g-1 at 1 mA cm-2 current density, respectively. Moreover, the Long Short-Term Memory-based machine learning technique was employed to model, predict, and forecast the chronoamperometric stability of MOF-derived BVC electrodes for oxygen evolution reaction applications, and the capacitive retention and Coulombic efficiency BVC electrodes. The exceptional performance of the BVC electrodes is attributed to their porous structure containing conducting carbon, which offers enhanced conductivity, larger surface area and increased reactive sites for efficient electronic and ionic transfer. This novel approach to the synthesis of MOF-derived BVC has opened up new pathways for future energy storage and conversion.
Keywords
INTRODUCTION
Nowadays, a society without portable electronic devices is inconceivable, as these devices have significantly modernized the way of living by making tasks easier and faster. In recent times, the rapid upsurge in the use of such electronic devices in daily life has ignited the development of efficient and portable,
Due to the energy required at every step, the OER kinetics are sluggish, leading to a high overpotential. Noble metal-based materials are reported as high-performing electrocatalysts, but their scarcity and high cost limit them from being used as commercial catalysts[14]. Therefore, to overcome both the above-mentioned issues, stable, economical, highly abundant, and robust multifunctional electrocatalytic materials with a minimized overpotential of tetra-electronic water oxidation reaction (eq. 1) and high energy density especially at high power densities need to be developed[15].
Recently, a diverse range of materials based on Metal-Organic Frameworks (MOFs) have been developed and investigated for various electrochemical applications including LIBs[16,17], SCs[18], electrocatalytic hydrogen evolution reaction[19], OER[20], owing to their attractive features including high surface area, highly porous framework, and well-defined metal-ligand arrangement offering more active sites to make them the most promising candidates for electrochemical applications[21]. Furthermore, due to the synthetic tunability, MOFs serve as an ideal template material and precursor to develop carbon matrix incorporated metallic nanomaterials on pyrolysis under an inert atmosphere. These materials have carbon matrices with metal nanomaterials offering extra accessibility of active sites to an electrolyte, resulting in a larger contact area between them which proved to be crucial behind enhanced electronic properties[22,23]. Recently, extensive work has been undertaken focusing on the development of non-noble mono-/bi-metallic MOF-derived metal oxides, sulfides, phosphides, etc. and used to fabricate working electrodes in SC and OER/hydrogen evolution reaction (HER) applications[24]. Lately, BiVO4 (BV) has gained attention in electrochemical and electrocatalytic OER applications due to its excellent physic-chemical properties and stability. However, pristine BV was found to show poor electronic conductivity which could adversely affect the capacitive retention (CR) of SC devices and the rate of a water oxidation reaction in OER. One of the best remedies to minimize this problem is to adopt a MOF-derived synthesis of BV, in which calcination of Bi-MOF precursor in the presence of suitable vanadium salt produces BV/C composite having larger surface area, better conductivity, and more number of surface active sites than bare BV. There are very few reports based on this approach to BV synthesis and its use in SC and OER applications[25].
In response to the above-mentioned concerns, herein, for the first time, we report the unique solid-state approach to synthesizing MOF-derived bismuth vanadate/carbon (BVC) composite by mechanically mixing the separately prepared monometallic Bi-MOF (using trimesic acid as a linker) and V-MOF (using terephthalic acid as a linker) precursors. In this study, rather than following the traditional method (where Bi-MOF and vanadium metal salt serve as precursors to synthesize the BVC catalysts), we opted to utilize separately prepared monometallic Bi-MOF (CAU-17) and V-MOF (MIL-47) as the Bi and V precursors. The rationale behind utilizing the two different linkers is their distinct coordination properties with respective metal nodes, i.e., Bi and V. With this approach, it is possible to achieve tailored properties with controlled morphology while mitigating the common challenges of uneven metal distribution and agglomeration typically observed in bimetallic MOFs[26]. This alternative approach is expected to further improve the catalytic properties of BVC as the use of two different linkers with different sp2 characters could improve specific surface area and electronic conductivity considerably which in turn decide the number of surface active sites. The use of two monometallic MOFs instead of bimetallic MOFs to synthesize bimetallic oxide materials is proven to be efficient in developing high-performance catalytic activity materials[26]. The desired structure was confirmed through several structural and morphological spectroscopic characterizations of the prepared BVC electrode. This was subsequently compared with a pristine BV sample, both of which were then subjected to SC and OER tests. The stability of the electrodes was also measured and analyzed by using a Long Short-Term Memory (LSTM)-based machine learning approach. The CR and Coulombic efficiency (CE) of BV and BVC SC electrodes were modeled, predicted and forecasted using this technique. Such a modeling approach opens new avenues to predict and forecast the critical parameters of the energy devices[27].
EXPERIMENTAL
Materials and reagents
Bismuth (III) nitrate pentahydrate [Bi(NO3)35H2O, Alfa Aesar], Vanadium (III)chloride (VCl3, Sigma Aldrich), Benzene-1,3,5-tricarboxylic acid (H3BTC, Sigma Aldrich), Benzene-1,4-dicarboxylic acid (H2BDC, Sigma Aldrich), Potassium hydroxide (KOH, SD Fine), Methanol (CH3OH, Spectrochem), and
Synthesis of CAU-17 (Bi-MOF)
To prepare Bismuth MOF (CAU-17), the 0.454 gm of Bi(NO3)3 5H2O and 0.957 gm of H3BTC were separately dissolved in a 30 mL mixture (1:1 V/V) of methanol and N, N-Dimethylformamide. The metal salt solution was then slowly added to the H3BTC solution and stirred for 15 min and then transferred into a 100 mL Teflon-lined stainless steel (SS) autoclave and heated at 120 ºC for 24 h. The reaction mixture was allowed to cool at room temperature and the obtained product was collected by centrifugation followed by washing with methanol. The washed product was dried at 80ºC overnight to obtain Bi-MOF.
Synthesis of MIL-47 (V-MOF)
To synthesize Vanadium MOF (MIL-47), 0.664 gm of H2BDC was dissolved in 50 mL N, N-Dimethylformamide and stirred until a clear solution was obtained. Once the solution was clear, it was placed in the dark, and 0.628 gm of VCl3 was added. The mixture was stirred for 30 min and ultrasonicated for 15 min in the dark (since VCl3 oxidizes under light). The solution was then transferred into a 100 mL Teflon-lined SS autoclave and heated at 120 ºC for 48 h, after which it was allowed to cool to room temperature. The obtained greenish product was collected by centrifugation at 4000 rpm, washed first with DMF then twice with methanol, and dried at 60 ºC for 12 h.
Synthesis of BV and BVC
The BV was synthesized by using obtained products from MOFs as a precursor for synthesis by mixing 1:1 (weight ratio) Bi-MOF and V-MOF, respectively, and grinding them in a mortar pestle for 15 min. This mixture was collected into a quartz crucible incorporated in a horizontal quartz tube furnace. The furnace was heated at a ramping rate of 4 ºC/min until it reached a final temperature of 450 ºC, where it was maintained for 120 min in air atmosphere and then allowed to cool naturally. BVC was synthesized by heating the Bi-MOF and V-MOF (1:1 weight ratio) mixture in an argon atmosphere at 700 ºC for 150 min, followed by calcination to 400 ºC for 120 min in the air at a ramping rate of 4 ºC/min. Scheme 1 displays a schematic illustration of all the steps involved in BV and BVC synthesis.
RESULTS AND DISCUSSION
Structural and morphological study
All the details on the instrumentation used for the physicochemical characterizations are provided in Supplementary Section 1.1. The phase formed and its crystal structure are characterized by X-ray diffraction (XRD) spectroscopy, as shown in Figure 1A. The diffraction peaks at 2θ 18.83°, 28.85°, 30.43°, 34.51°, 35.32°, 39.91°, 42.40°, 46.36°,47.05°, 50.12°, 53.35°, 55.70°, 58.46° and 59.23° corresponded to the (110), (121), (040), (200), (020), (211), (150), (132), (240), (202), (161), (215), (321) and (123) planes, respectively.
Figure 1. (A) X-ray diffraction patterns of the BV and BVC samples and the inset shows the (110) plane shifting; (B) The FTIR plots of BV and BVC; (C) N2 adsorption-desorption isotherms for BV and BVC (D) TGA of Bi V-MOF, BV, and BVC samples. BV: BiVO4; BVC: bismuth vanadate/carbon; FTIR: fourier-transform infrared spectroscopy; TGA: thermogravimetric analysis; MOF: metal-organic framework.
The crystalline monoclinic phase of BV and BVC was confirmed as all the peaks are in line with the planes of the JCPDS card No.14-0688[28,29]. No any distinct peak was observed in the XRD spectrum of BV and BVC due to minimal amount of carbon in BVC, but the hump from 15°-30° evidenced the presence of an amorphous carbon matrix. Interestingly, a slight shift in the peaks toward higher 2θ values was observed with the addition of carbon compared to pure bismuth vanadate. The peak along the (110) plane reveals an approximate 0.03° shift, confirming the effective incorporation of carbon in BV lattice. This peak shift in the case of BVC is due to stress and strain induced by the carbon via creating minor defects or distortion in BVC without damaging the overall structure. This shift evidences the intimate interaction between BV and carbon, thereby confirming the successful formation of the BVC composite. Additionally, the peak intensity of the BVC sample decreased with the carbon incorporation, compared to pristine BV. This decrease in intensity is attributed to local structural inhomogeneity, resulting in reduced crystallization. The crystallite size was determined using the full width at half maximum (FWHM) of the peak along the plane (110). In the case of the BV sample, the FWHM was 0.5401, while for the BVC sample, it was 0.5969 and the values are provided in Supplementary Table 1. Based on these FWHM values, the crystallite sizes were calculated using the Scherrer Supplementary Equation 1 yielding 14.908 nm for the BV sample and 13.489 nm for the BVC sample. Furthermore, the dislocations are one-dimensional crystalline defects that define the boundary between slipped and unslipped regions within a material. These defects disrupt the regular atomic arrangement found in a perfect crystal. The extent of defects in the material is quantified by a measure known as dislocation density (ρD). The ρD refers to the number of dislocations per unit volume of crystalline material. A smaller ρD combined with larger grain sizes indicates a more crystallization process and the larger ρD combined with smaller grain sizes indicates a more amorphization process[30]. The term microstrain (ε) is defined as the deformation of an object divided by its effective length. The ε and ρD were calculated using Supplementary Equations 2 and 3. The ε and ρD for the BV sample were
The prepared samples were characterized by using Fourier-transform infrared spectroscopy (FTIR), as shown in Figure 1B. The peak at 477 cm-1 is of Bi-O and 717 cm-1 and 828 cm-1 for V-O in VO4-3 confirming the synthesis of BV monoclinic phase in both BV and BVC samples[31,32]. The bands at 1,384 cm-1 and
The surface area of BVC and BV were recorded using Brunauer-Emmett-Teller surface (SBET) analysis, as shown in Figure 1C. The obtained surface area of BVC and BV were found to be 26.839 m2/g and
The thermogravimetric analysis (TGA) of Bi V-MOF, BV, and BVC is shown in Figure 1D. Four weight loss processes are observed for Bi V-MOF. The first occurs from room temperature to 122 ºC, primarily due to the elimination of coordinated water molecules. The second, from 122 ºC to 300 ºC, is attributed to the removal of volatile organic compounds. The third, from 300 ºC to 465 ºC, corresponds to the disintegration of the framework in the sample. The fourth, from 465 ºC onwards, involves the complete oxidation of carbon residues and the oxidation of MOFs. The BV sample showed weight loss of up to 125 ºC due to dehydration and removal of volatile organic compounds up to 310 ºC from 310 ºC; the peak drop is attributed to the quick change in phase of the material and the crystallization of material takes place. The BVC sample showed negligible weight loss (approximately 5%) beyond 465 ºC due to the loss of carbon remaining after carbonization at 700 ºC, confirming the presence of carbon in the BVC[26].
Field Emission-Scanning Electron Microscopy (FE-SEM) was used to determine the morphology of the prepared samples. Figure 2 displays FE-SEM images of BVC and BV. The uneven distribution of the BV nanoparticles and agglomeration can be seen in Figure 2A and B. Figure 2C and D shows the micrographs of BVC and the uniformly distributed coral-like nanoparticles. Additionally, the diffusion length can be greatly shortened by the cavities and gaps created by uniformly distributed coral-like nanoparticles, facilitating easy ion intercalation across the material[36]. For the BV and BVC samples, the average particle size was determined and noted in histograms, and the average particle diameter was determined to be around 107 nm, and 75 nm, respectively, as shown in Figure 2E and F. The obtained results demonstrate that the incorporation of carbon affects the materials morphology and structure, which may further influence the electrochemical performance. The elemental mapping of BVC is shown in Figure 2G-K representing Bi, V, O, and C elements, respectively. The elemental mapping has confirmed the presence of doped carbon in the lattice of BV nanoparticles.
Figure 2. FE-SEM images of (A and B) BV; (C and D) FESEM of BVC; (E) Histogram for average particle size of BV; (F) Histogram for average particle size of BVC, and the elemental mapping of BVC sample with, (G) overlay, (H) Bi, (I) V, (J) O, and (K) C elements. BV: BiVO4; BVC: bismuth vanadate/carbon; FE-SEM: field emission-scanning electron microscopy.
To support the FE-SEM and morphology, the BV and BVC samples were further investigated using the High-Resolution Transmission Electron Microscopy (HRTEM) technique. HRTEM images of BV at low and high magnifications show the nanoparticle-like morphology in Figure 3A. It is possible to clearly identify the lattice fringes of BV nanoparticles from the higher-resolution magnified image [Figure 3B and C]. The lattice d-spacing of 0.311 nm was derived from the lattice fringes, which corresponded to the (121) plane of the BV and BVC at 28.85° nanoparticles. Likewise, HRTEM was used to examine the BVC material, as displayed in Figure 3D-F at various magnifications. It was illustrated that the BVC coral-like nanoparticles are irregularly arranged, with carbon nanoparticles positioned in between them. Furthermore, Figure 3F makes it easy to identify the lattice fringes associated with the BV nanoparticles in the BVC material. Based on the lattice fringes, the BVC coral-like nanoparticles (121) plane is represented by the measured interplanar spacing of 0.306 nm. According to the findings, the BVC d-values from the XRD pattern were likewise well-coordinated with HRTEM results[37,38].
Figure 3. HRTEM of (A-C) BV, and (D-F) BVC samples. BV: BiVO4; BVC: bismuth vanadate/carbon; HRTEM: high-resolution transmission electron microscopy.
The surface properties of the synthesized materials such as elemental composition and their bonding states (oxidation states) were studied by X-ray photoelectron spectroscopy (XPS) [Figure 4]. The survey spectrum of BV and BVC samples displays the peaks of each element present in the samples. The presence of Bismuth (4f), Vanadium (2p), Oxygen (1s), and Carbon (1s) at their respective positions of binding energy (BE) is confirmed by XPS survey spectra presented in Supplementary Figure 2. Figure 4A is the high-resolution XPS spectra of Bi 4f of BV and BVC samples showing two broad peaks of Bi3+. The BEs of 164.4 eV and 164.28 eV represent Bi 4f5/2, while 159.09 eV and 158.98 eV correspond to Bi 4f7/2, respectively. The difference between the BEs of both peaks agrees well with pure BV. The high-resolution XPS-spectra of V 2p [Figure 4B] were fitted into two peaks of V 2p3/2 and V 2p1/2 at the BEs of 517 eV and 524.3 eV for BV and 516.7 eV and 524 eV for BVC. The V 2p3/2 deconvoluted into two distinct peaks at the BE of 517 eV and 515.8 eV and 516.7 eV and 515.3 eV for BV and BVC, respectively, representing the presence of V5+ and V4+. Figure 4C represents the high-resolution spectra of O 1s which was fitted into three peaks at the BE 529.9 eV, 531 eV, and 532.7 eV for BV and 529.7 eV, 530.7 eV and 532.6 eV for BVC indexed for metal-oxygen bonds (Bi-O or V-O bonds), the hydroxide or crystal defects and the chemisorbed oxygen or surface adsorbed oxygen, respectively. Figure 4D represents the C 1s deconvoluted into two peaks at BEs of
Oxygen evolution reaction study
The electrocatalytic OER performance of the prepared catalyst was studied in 1M KOH solution. The standard three-electrode system was used for testing the electrodes and the details of electrochemical measurements are provided in Supplementary Section 1.2. Figure 5A represents the linear sweep voltammetry (LSV) at 1 mV s-1 of BVC and BV. The thermodynamic potential for water splitting is 1.23 V vs. reversible hydrogen electrode (RHE); the active OER catalyst should attain this potential at a 10 mA cm-2 current density[44]. The measured voltages were converted from Hg/HgO to the RHE scale using the Nernst Supplementary Equation 5. The overpotential (η10) calculated using Supplementary Equation 6 required to reach the current density of 10 mA cm-2 for MOF-derived BVC is 364 mV, which is much lesser than the 432 mV observed for BV. This indicates that BV alone has limited performance, but its efficiency and stability are significantly improved when modified with MOF-derived doped carbon.
Figure 5. (A) Linear sweep voltammetry (LSV) curves of as-prepared catalysts BV and BVC electrodes; (B) Corresponding Tafel slopes; (C) EIS Nyquist plots 1 MHz to 10 mHz; (D and E) Cyclic voltammograms of BV and BVC; (F) Current density with respect to scan rates of BV and BVC electrodes, i.e., double layered capacitance (Cdl); (G) The actual, predicted, and forecasted chronoamperometry (CA) curve of BVC in 1.0 M KOH; (H) LSV curves for OER measured before and after CA stability at 1 mV s-1 scan rate; (I) EIS Nyquist plots of the electrode before and after the stability test. BV: BiVO4; BVC: bismuth vanadate/carbon; OER: oxygen evolution reaction.
Figure 5B shows the Tafel slopes obtained from LSV curves, calculated using Supplementary Equation 7. BVC exhibits a lower Tafel slope of 51 mV dec-1, while BV shows a Tafel slope of 61 mV dec-1. The lower overpotential and Tafel slope indicate that BVC showcases better OER activity than BV. Moreover, in Figure 5C, electrochemical conductivity studies were conducted at an open circuit voltage employing electrochemical impedance spectroscopy (EIS) to examine their intrinsic transport properties. The equivalent circuit was developed by fitting the matched data. Each electrode shows discrete semicircles at high frequencies and a straight line at low frequencies. A semicircle radius is assigned to the charge-transfer resistance (Rct) in the electrochemical process between the electrolyte and the electrode. The smaller radius of the semicircle points to a lower Rct and a higher electron transfer rate. The curve’s intercept at the real axis or its starting point in the high-frequency region corresponds to the solution resistance (Rs). The Warburg impedance (Rw) values, which offer insights into the ion diffusion process, and the constant phase element (CPE) values, which detail the active interface of the electrodes, are presented in Supplementary Table 2. Rs is the intercept on the horizontal axis containing electrolyte resistance, contact resistance among the current collector and the active substances, and internal electrode resistance. The quasi-semicircle signifies the Rct determined by the electrostatic interactions and faradic redox process, which proceeds at the electrode and electrolyte interface. The slope of the straight line specifies Rw, signifying the charge diffusion kinetics process within the active material. The lower values of Rs and Rct of BVC (0.018 Ω and 0.5 Ω) compared to those of BV (0.003 Ω and 0.8 Ω) clearly confirm the accelerated charge transfer process in BVC, resulting in superior OER activity.
The electrochemical double-layer capacitance (Cdl) of the electrodes was calculated using cyclic voltammetry (CV) in a non-Faradic region of 0.924 V to 1.22 V vs. RHE. The CV curves were obtained from
Supercapacitor study
Electrochemical performance evaluation of BV and BVC electrodes
The electrochemical characteristics of the BVC and BV electrodes were investigated using CV, Galvanostatic Charge Discharge (GCD), and EIS. The three-electrode system was used, with BVC and BV as the working electrodes, a platinum wire as a counter electrode, and Hg/HgO as a reference electrode. The CV measurements of BV and BVC at scan rates from 100 mV s-1 to 10 mV s-1 in the potential window of 0 V to -1.0 V vs. Hg/HgO were measured in 1 M KOH as an electrolyte. In Figure 6A and B, the CV curves of both BV and BVC showing the oxidation and reduction peaks can be attributed to the reversible faradic process Bi+3 ↔ Bi0. The only reduction peak from -0.7 V to -0.85 V was due to Bi3+ to Bi0 and the couple of oxidation peaks from -0.5 V to -0.4 V and -0.375 to -0.275 were attributed to the Bi0 to Bi1+ and Bi1+ to Bi3+. The shifting of oxidation peaks towards positive potential and the shifting of reduction peaks to negative potential are attributed to the internal resistance (IR) drop factor, observed from the 100 mV s-1 to 10 mV s-1 scan rates, respectively[45]. The nature of CV for BVC and BV is the same as the presence of the carbon provides a higher surface area and accommodates more electrolyte ions with quick migration. To gain a better understanding of the performance, the cyclic voltammograms (CVs) of the bare SS electrode and BVC were compared in Supplementary Figure 3A. Additionally, the composition of the electrodes, with respect to the active material, activated carbon and binder, was varied during electrode fabrication; the optimized performance was evaluated. The resulting CVs were recorded and compared in Supplementary Figure 3B. Hence, the higher current response observed in BVC results in a higher specific capacity compared to BV.
Figure 6. (A and B) Comparative CV curves of BV and BVC; (C) Plot of the log of current density vs. log of scan rate for BV and BVC electrodes to calculate the “b” values in CV curves; (D) The plot of charge stored vs. reciprocal of square root of potential scan rate of BV and BVC; (E and F) Comparison of the surface and diffusive charge contribution at scan rates (5-100 mV s-1) in BV and BVC electrodes. BV: BiVO4; BVC: bismuth vanadate/carbon; CV: cyclic voltammetry.
The redox nature reveals the pseudocapacitive electrical activity of electrodes. Subsequently, the capacitive contribution of BVC and BV electrodes was calculated using the Power law Supplementary Equations 11 and 12 to determine the charges stored by the surface (involving non-faradic reactions adsorption-desorption of charges) and/or the diffusion charge storage (bulk intercalation process) from the CV curves of BV and BVC electrodes. The contribution of charge storage is calculated from the constant b (slope of linear graph) by plotting the log of scan rate (mV s-1) vs. log of current density line (A g-1). The b value of ~1 indicates a surface control process (non-faradic) dominance, while a b value of ~0.5 represents a diffusion control process (faradic) dominance. The b values in Figure 6C of BV and BVC are 0.56 and 0.6, respectively, indicating that the BVC electrode exhibits both surface-controlled and diffusion-controlled processes.
The amount of charge stored in BV and BVC was quantified by the contribution of observed charge density (Qt), calculated using the modified Powers law for the surface pseudocapacitive process (Qs - Isurface) and battery-like diffusive process (Qd - Idiffusive). Qt is the total stored charge, Qs is the surface stored charge related to electric double-layer capacitors (EDLCs), and Qd is the diffusion stored charge, which occurs in the bulk. The Qd and Qs are dependent on the square root of the scan rate. To calculate the approximate values of Qs and Qd, the plot of total voltammetric charge (Qt, charge stored in C g-1) vs. the reciprocal square root of sweep rate is shown in Figure 6D. Supplementary Equations 13 and 14 were used to measure the contribution of Qd and Qs in total charge contribution. The proportion of Isurface and Idifussive charge stored contribution in BV and BVC is depicted in Figure 6E and F. The sky blue and red area represents the Idifussive (bulk) and Isurface (capacitive) charge storage contribution in the total current, respectively. The Isurface (capacitive) contribution emerges with higher scan rates. The sample BVC has an Isurface (capacitive) contribution of 76%, while BV has a contribution of 70% at 100 mV s-1. This confirms the effective redox kinetics for BVC are enhanced as the in-situ carbon incorporation takes place.
The GCD curves of BV and BVC electrodes at different current densities from 1 to 5 mA cm-2 between the potential window of 0 V to -1.0 V were recorded and displayed in Figure 7A and B. These curves exhibited non-linear charge-discharge behavior, representing pseudocapacitive properties of BV. The performance, in terms of specific capacity, for BV and BVC was calculated using Supplementary Equations 15 and 16 from the GCD curves. The obtained specific capacities of BV and BVC are 45.14 mAh g-1 (162.5 C g-1) and
Figure 7. (A and B) GCD curves at different current densities for BV and BVC electrodes; (C) Comparative GCD curves of corresponding electrodes at 1 mA cm-2 current density; (D) Plots of current density vs. specific capacity; (E and F) Actual, predicted, and forecast capacitive retention and Coulombic efficiency of BV and BVC electrodes. BV: BiVO4; BVC: bismuth vanadate/carbon; GCD: galvanostatic charge discharge.
Aqueous symmetric supercapacitor device study
Based on the excellent performance of BVC electrodes, the practical and commercial applicability of BVC was also tested by fabricating and testing the aqueous and solid-state type of symmetric SC (SSc).
The aqueous symmetric SC (ASc) device is fabricated and the steps in fabrication are provided in Supplementary Figure 4 where the BVC electrode acts as an anode and cathode in 1M KOH electrolyte. For improved SSc performance, it is important to attain the equilibrium of charge between the electrodes using the relationship q+ = q-, where q+ is the charge stored on the surface of the positive and q- is the charge stored on the negative electrode. The important parameters strongly influence the specific capacitance (SC), potential (ΔV) and the mass of the electrode material (m). The mass on the cathode and anode is balanced and the performance ASc is measured in 1M KOH electrolyte by taking the CV from 0 V to 1.4 V voltage window. The CV curves of the ASc device are recorded at various scan rates (10 mV s-1 to 100 mV s-1), as represented in Figure 8A. The shape of CV indicates that high current density does not cause distortion on the electrode, indicating the stability of BVC material at 100 mV s-1. This finding emphasizes the rate capabilities of an ASc device, exhibiting its ability to maintain high scan rates while remaining highly reversible.
Figure 8. (A) CV curves at different current densities for BVC aqueous symmetric supercapacitor (ASc) device; (B) GCD curves of the ASc device; (C) Bar diagram for specific capacitance at various current densities; (D) Ragone plots of specific energy and specific power; (E) Actual, predicted, and forecast capacitive retention for 2000 cycles; (F) EIS Nyquist plots of before and after stability of the ASc device. BVC: Bismuth vanadate/carbon; CV: cyclic voltammetry; GCD: galvanostatic charge discharge; EIS: electrochemical impedance spectroscopy.
The GCD measurements of the fabricated ASc device were also recorded at 1 to 5 mA cm-2 current densities at 0 V to 1.4 V voltage window represented in Figure 8B. The pseudocapacitive behavior can be observed and the SC was evaluated from the GCD curves with the highest SCs of 160.9 F g-1 at 1 mA cm-2 current density using Supplementary Equation 17. The SC of the device from GCD curves from 1 mA cm-2 to
The ASc device is further used for actual practical applications to demonstrate the capability of the material to store and rapidly release energy by rotating a mini fan with two leaves. This application gives the materials SE, SP, and discharge behavior. The demonstration of fan rotation is shown in Figure 9 and in Supplementary Video 2. The device is charged at 2.8V for 30 s as the two devices are connected in series and it takes 54 s to discharge by rotating the leaf of a fan.
Soid-state symmetric supercapacitor device study
The performance of ASc is promising. To support these results, a solid-state SSc is also constructed; as compared to aqueous electrolyte-based devices, solid-state devices offer an extensive range of applications due to advantages such as easy handling, no spillage, and great performance consistency. SSc was fabricated using BVC as both the anode and cathode material on a 5 × 5 cm2 SS sheet. The fabrication process is provided in Supplementary Section 1.3, with polyvinyl alcohol in 1 M KOH (PVA-KOH) as the gel electrolyte. The preparation of the gel electrolyte is described in Supplementary Section 1.4. The electrochemical performance of the device is tested within a 0 V to 1.4 V voltage window, similar to ASc, after optimizing the voltage range from 1.2 V to 1.6 V at a 50 mV s-1 scan rate. The CV analysis is performed at various scan rates (10 mV s-1 to 100 mV s-1) within a 0 V to 1.4 V voltage window, yielding a perfectly symmetrical shape, as depicted in Figure 10A, which indicates the accurate mass and charge balance between the electrodes.
Figure 10. (A) CV curves at different current densities for BVC Solid-state symmetric supercapacitor (SSc) device; (B) GCD curves of the SSc device; (C) Bar diagram for specific capacitance at various current densities; (D) Ragone plots of specific energy and specific power; (E) Actual, predicted, and forecast capacitive retention for 2000 cycles; (F) EIS Nyquist plots of before and after stability of the SSc device. BVC: Bismuth vanadate/carbon; CV: cyclic voltammetry; GCD: galvanostatic charge discharge; EIS: electrochemical impedance spectroscopy.
The GCD analysis was used to evaluate the SCs of the device and the recorded GCD from 1 to 5 mA cm-2 current densities at 0 V to 1.4 V voltage window plotted in Figure 10B. The symmetrical charge and discharge curve indicated the steady charge transfer during charging and discharging. The obtained SC of the device is 109.8 F g-1 at 1 mA cm-2 current density [Figure 10C]. The Specific Energy (SE) and Specific Power (SP) of the SSc device are calculated and plotted in the Ragone plot Figure 10D. The device exhibits a high SE of 29.91 Wh kg-1 SE at a 5.3 kW kg-1 SP, which remains at 21.83 Wh kg-1 SE at a 26.9 kW kg-1. The SE and SP of the device are compared with literature values and shown in the Ragone plot [Supplementary Figure 5]. The Ragone plot indicates that this material showed excellent SP and SE when compared to existing literature. The cyclic stability of the fabricated SSc was investigated for 2000 charging-discharging cycles at 5 mA cm-2 with 86.36% CE. The observed CRs of 98.62% (actual), 98.64% (predicted), and 97.56% (forecasted) for the next 500 cycles are depicted in Figure 10E. The EIS measurements of before and after cyclic stability of the SSc are represented in Figure 10F. The circuit parameters are represented in Supplementary Table 5.
Similar to the ASc device, the SSc is used in practical applications by rotating a fan to record discharge behavior, as shown in Figure 11 and in Supplementary Video 3. The device is charged at 2.8 V for 30 s while the two devices are connected in series, and it discharges by rotating the fan for 33 s.
Figure 11. Photographs of the practical application of rotating the fan using the SSc device of BVC in charging and discharging mode. BVC: Bismuth vanadate/carbon; SSc: solid-state symmetric supercapacitor.
Overall, the conductivity of the BV nanoparticles, doped with adventitious carbon and synthesized through a simple and efficient MOF-derived approach, exhibited exceptional performance in OER and SC applications within flexible ASc and SSc devices. This remarkable performance can be attributed to several factors: (i) the successful incorporation of an optimal amount of sp2 hybridized carbon into the BV matrix, resulting in improved electronic conductivity; (ii) the uniform distribution of coral-like BV nanoparticles with diminished particle size and elevated RF values, which significantly reduce the charge-carrier diffusion length by generating oxygen vacancies; (iii) an enhanced electrochemically active surface area, as indicated by increased Cdl and ECSA values; and (iv) lower EIS values (Rs and Rct), which facilitate effective interactions between the working electrode material and the electrolyte. Furthermore, the LSTM-based machine learning approach effectively models, predicts, and forecasts the OER and supercapacitive performance of BVC electrodes.
CONCLUSIONS
In summary, we demonstrated a new approach to synthesize multifunctional MOF-derived BV/carbon composite using monometallic Bi and V MOFs via solid-state grinding and high-temperature pyrolysis. The well-characterized samples were then utilized to test the multifunctional behavior of the as-prepared samples toward electrochemical OER and SC applications. The BVC sample showed exceptional performance for OER with an overpotential of 364 mV at the current density of 10 mA cm-2 and the SC has a high specific capacity of 134.17 mAh g-1 (483 C g-1) at 1 mA cm-2 current density. The constructed ASc device shows a SC of 160.9 F g-1 at a current density of 1 mA cm-2 and excellent stability with a CR of 96.25%. Similarly, the SSc device shows a SC of 109.8 F g-1 at a 1 mA cm-2 current density along with a 98.62% CR. The LSTM-based machine learning technique successfully models, predicts, and forecasts the chronoamperometric stability of MOF-derived BVC electrodes and CR and CE of BV and BVC electrodes. The as-designed aqueous and solid-state devices have been successfully applied to rotate a two-blade fan. The excellent performance of BVC indicates its enhanced physicochemical properties due to carbon doping. This synthesis method for bimetallic MOFs has opened up new pathways for creating similar materials for multifunctional applications.
DECLARATIONS
Author’s contributions
Writing - original draft, methodology, investigation, conceptualization: Chaudhari, S. A.
Writing - review and editing, formal analysis: Patil, V. V.
Investigation, data curation: Jadhav, V. A.
Data curation, formal analysis: Thorat, P.
Data curation, formal analysis: Sutar, S. S.
Writing - review and editing, data curation, formal analysis: Dongale, T. D.
Writing - review and editing, data curation: Parale, V.
Formal analysis: Patil, V.
Writing - review and editing, supervision, conceptualization: Mhamane, D. S.
Writing - review and editing, supervision, funding acquisition, conceptualization: Mali, M. G.
Writing - review and editing, supervision, funding acquisition, conceptualization: Park, H. H.
All authors reviewed the results and approved the final manuscript version.
Availability of data and materials
The rata data supporting the findings of this study are available within this Article and its Supplementary Materials. Further data are available from the corresponding authors upon request.
Financial support and sponsorship
Funding for this work was provided through the Brain Pool Program supported by the Ministry of Science and ICT via the National Research Foundation of Korea (Grant No: RS-2024-00444441). This work was supported by the National Research Foundation of Korea (NRF), funded by the Korea government (MSIT) (RS-2020-NR049541). Author Chaudhari, S. A. acknowledges the Mahatma Jyotiba Phule Research and Training Institute (MAHAJYOTI), Nagpur (An Autonomous Institute of the Other Backward Class Bahujan Welfare Department, Government of Maharashtra), for awarding the MAHAJYOTI FELLOW honor and offering financial support under the Mahatma Jyotiba Phule Research Fellowship-2022 (MAHAJYOTI2022/Ph.D. Fellow/ 1002(829).
Conflicts of interest
All authors declared that there are no conflicts of interest.
Ethical approval and consent to participate
Not applicable.
Consent for publication
Not applicable.
Copyright
© The Author(s) 2025.
Supplementary Materials
REFERENCES
1. Patil, V. V.; Pujari, S. S.; Bhosale, S. B.; et al. Hydrous and amorphous cobalt phosphate thin-film electrodes synthesized by the SILAR method for high-performing flexible hybrid energy storage devices. Energy. Fuels. 2022, 36, 12791-806.
2. Reddy, C. V.; Reddy, I. N.; Ravindranadh, K.; et al. Effect of noble metal ions dopants on solar photoelectrochemical water splitting and electrochemical supercapacitive performance of BiVO4 hollow tubes. Sol. Energy. Mater. Sol. Cells. 2021, 226, 111056.
3. Ismail KB, Arun Kumar M, Jayavel R, Arivanandhan M, Mohamed Ismail MA. Enhanced electrochemical performance of the MoS2/Bi2S3 nanocomposite-based electrode material prepared by a hydrothermal method for supercapacitor applications. RSC. Adv. 2023, 13, 24272-85.
4. Jiao, S.; Zhou, A.; Wu, M.; Hu, H. Kirigami patterning of MXene/bacterial cellulose composite paper for all-solid-state stretchable micro-supercapacitor arrays. Adv. Sci. 2019, 6, 1900529.
5. Liu, H.; Liu, X.; Wang, S.; Liu, H.; Li, L. Transition metal based battery-type electrodes in hybrid supercapacitors: a review. Energy. Storage. Mater. 2020, 28, 122-45.
6. Dubal, D. P.; Patil, D. R.; Patil, S. S.; Munirathnam, N. R.; Gomez-Romero, P. BiVO4 fern architectures: a competitive anode for lithium-ion batteries. ChemSusChem 2017, 10, 4163-9.
7. Devi, N.; Ray, S. S. Performance of bismuth-based materials for supercapacitor applications: a review. Mater. Today. Commun. 2020, 25, 101691.
8. Shinde, N. M.; Shinde, P. V.; Mane, R. S.; Ho, K. K. Solution-method processed Bi-type nanoelectrode materials for supercapacitor applications: a review. Renew. Sustainable. Energy. Rev. 2021, 135, 110084.
9. Cao, Z.; Fu, J.; Wu, M.; Hua, T.; Hu, H. Synchronously manipulating Zn2+ transfer and hydrogen/oxygen evolution kinetics in MXene host electrodes toward symmetric Zn-ions micro-supercapacitor with enhanced areal energy density. Energy. Storage. Mater. 2021, 40, 10-21.
10. Isacfranklin, M.; Deepika, C.; Ravi, G.; Yuvakkumar, R.; Velauthapillai, D.; Saravanakumar, B. Nickel, bismuth, and cobalt vanadium oxides for supercapacitor applications. Ceram. Int. 2020, 46, 28206-10.
11. Patil, S. S.; Dubal, D. P.; Tamboli, M. S.; et al. Ag:BiVO4 dendritic hybrid-architecture for high energy density symmetric supercapacitors. J. Mater. Chem. A. 2016, 4, 7580-4.
12. Deeloed, W.; Priamushko, T.; Čížek, J.; Suramitr, S.; Kleitz, F. Defect-engineered hydroxylated mesoporous spinel oxides as bifunctional electrocatalysts for oxygen reduction and evolution reactions. ACS. Appl. Mater. Interfaces. 2022, 14, 23307-21.
13. Xie, X.; Du, L.; Yan, L.; et al. Oxygen evolution reaction in alkaline environment: material challenges and solutions. Adv. Funct. Mater. 2022, 32, 2110036.
14. Heckel, S.; Wittmann, M.; Reid, M.; Villa, K.; Simmchen, J. An account on BiVO4 as photocatalytic active matter. Acc. Mater. Res. 2024, 5, 400-12.
15. Kubba, D.; Ahmed, I.; Kour, P.; et al. LaCoO3 perovskite nanoparticles embedded in NiCo2O4 nanoflowers as electrocatalysts for oxygen evolution. ACS. Appl. Nano. Mater. 2022, 5, 16344-53.
16. Shen, M.; Ma, H. Metal-organic frameworks (MOFs) and their derivative as electrode materials for lithium-ion batteries. Coord. Chem. Rev. 2022, 470, 214715.
17. Lin, W.; Zhao, S.; Lu, B.; Jiang, F.; Lu, Z.; Xu, Z. Structures, performances and applications of green biomass derived carbon in lithium-ion batteries. Energy. Mater. 2024, 4, 400078.
18. Chettiannan, B.; Dhandapani, E.; Arumugam, G.; Rajendran, R.; Selvaraj, M. Metal-organic frameworks: a comprehensive review on common approaches to enhance the energy storage capacity in supercapacitor. Coord. Chem. Rev. 2024, 518, 216048.
19. Li, J.; Jia, J.; Wang, D.; Dong, H.; Zhu, M. Recent research progress of MOFs-based heterostructures for photocatalytic hydrogen evolution. Chem. Eng. J. 2024, 498, 155194.
20. Qi, Q.; Zhang, C.; Hu, J. Triggered factors and structure-activity relationship in the dynamic reconstruction processing of MOF for the alkaline oxygen evolution reaction. Coord. Chem. Rev. 2025, 522, 216235.
21. Khan, M. S.; Li, Y.; Yang, L.; et al. Improving capacitive deionization performance through tailored iodine-loaded ZIF-8 composites. Desalination 2024, 579, 117486.
22. Li, S.; Gao, Y.; Li, N.; Ge, L.; Bu, X.; Feng, P. Transition metal-based bimetallic MOFs and MOF-derived catalysts for electrochemical oxygen evolution reaction. Energy. Environ. Sci. 2021, 14, 1897-927.
23. Du, J.; Li, F.; Sun, L. Metal-organic frameworks and their derivatives as electrocatalysts for the oxygen evolution reaction. Chem. Soc. Rev. 2021, 50, 2663-95.
24. Zhu, Z.; Duan, J.; Chen, S. Metal-organic framework (MOF)-based clean energy conversion: recent advances in unlocking its underlying mechanisms. Small 2024, 20, 2309119.
25. Reddy C, Neelakanta Reddy I, Koutavarapu R, Reddy KR, Kim D, Shim J. Novel BiVO4 nanostructures for environmental remediation, enhanced photoelectrocatalytic water oxidation and electrochemical energy storage performance. Solar. Energy. 2020, 207, 441-9.
26. Chaudhari, S.; Patil, V.; Jadhav, V.; et al. Linker encouraged solid state synthesis of MOF derived Z-scheme NiCo2O4/NiO/C toward efficient removal of organic and inorganic pollutants from water. Langmuir 2024, 40, 19804-15.
27. Sonkawade, A. R.; Mahajan, S. S.; Shelake, A. R.; et al. The g-C3N4/rGO composite for high-performance supercapacitor: synthesis, characterizations, and time series modeling and predictions. Int. J. Hydrogen. Energy. 2024, 87, 1416-26.
28. Kolhe, N. D.; Walekar, L. S.; Kadam, A. N.; et al. MOF derived in-situ construction of core-shell Z-scheme BiVO4@ -Fe2O3-CF nanocomposites for efficient photocatalytic treatment of organic pollutants under visible light. J. Clean. Prod. 2023, 420, 138179.
29. Packiaraj, R.; Venkatesh, K.; Devendran, P.; Bahadur, S. A.; Nallamuthu, N. Structural, morphological and electrochemical studies of nanostructured BiVO4 for supercapacitor application. Mater. Sci. Semicond. Process. 2020, 115, 105122.
30. Mathad, S. N.; Jadhav, R. N.; Patil, N. D.; Puri, V. Structural and mechanical properties of Sr+2-doped bismuth manganite thick films. Int. J. Self-Propag. High-Temp. Synth. 2013, 22, 180-4.
31. Orimolade, B. O.; Arotiba, O. A. An exfoliated graphite-bismuth vanadate composite photoanode for the photoelectrochemical degradation of acid orange 7 dye. Electrocatalysis 2019, 10, 429-35.
32. Samsudin, M. F. R.; Jayabalan, P. J.; Ong, W.; Ng, Y. H.; Sufian, S. Photocatalytic degradation of real industrial poultry wastewater via platinum decorated BiVO4/g-C3N4 photocatalyst under solar light irradiation. J. Photochem. Photobiol. A. Chem. 2019, 378, 46-56.
33. Biswas, M. R. U. D.; Ho, B. S.; Oh, W. Eco-friendly conductive polymer-based nanocomposites, BiVO4/graphene oxide/polyaniline for excellent photocatalytic performance. Polym. Bull. 2020, 77, 4381-400.
34. Sajid, M. M.; Khan, S. B.; Javed, Y.; et al. Facile synthesis of Se/BiVO4 heterojunction composite and evaluation of synergetic reaction mechanism for efficient photocatalytic staining of organic dye pollutants in wastewater under visible light. J. Mater. Sci. Mater. Electron. 2020, 31, 19599-612.
35. Phanichphant, S.; Nakaruk, A.; Chansaenpak, K.; Channei, D. Evaluating the photocatalytic efficiency of the BiVO4/rGO photocatalyst. Sci. Rep. 2019, 9, 16091.
36. Patil, V. V.; Kumar, N.; Salunkhe, R. R.; et al. Crystallinity transformation engineering of hydrous cobalt nickel phosphate cathodes for hybrid supercapacitor devices: extrinsic/battery to intercalation type pseudocapacitors. Chem. Eng. J. 2024, 485, 150055.
37. Subramanyam, P.; Khan, T.; Neeraja, S. G.; Suryakala, D.; Subrahmanyam, C. Plasmonic Bi nanoparticle decorated BiVO4/rGO as an efficient photoanode for photoelectrochemical water splitting. Int. J. Hydrogen. Energy. 2020, 45, 7779-87.
38. Patil, S. S.; Dubal, D. P.; Deonikar, V. G.; et al. Fern-like rGO/BiVO4 hybrid nanostructures for high-energy symmetric supercapacitor. ACS. Appl. Mater. Interfaces. 2016, 8, 31602-10.
39. Biesinger, M. C. Accessing the robustness of adventitious carbon for charge referencing (correction) purposes in XPS analysis: insights from a multi-user facility data review. Appl. Surf. Sci. 2022, 597, 153681.
40. Wu, J.; Chen, Y.; Pan, L.; et al. Multi-layer monoclinic BiVO4 with oxygen vacancies and V4+ species for highly efficient visible-light photoelectrochemical applications. Appl. Catal. B. Environ. 2018, 221, 187-95.
41. Guo, M.; Wang, Y.; He, Q.; et al. Enhanced photocatalytic activity of S-doped BiVO4 photocatalysts. RSC. Adv. 2015, 5, 58633-9.
42. Nguyen, T. D.; Bui, Q. T. P.; Le, T. B.; et al. Co2+ substituted for Bi3+ in BiVO4 and its enhanced photocatalytic activity under visible LED light irradiation. RSC. Adv. 2019, 9, 23526-34.
43. Mir, R. A.; Pandey, O. P. Role of morphological features and oxygen vacancies on electrocatalytic oxygen evolution reaction (OER) activity and pseudocapacitance performance of BiVO4 structures. Appl. Phys. Lett. 2021, 118, 253902.
44. Patil, V. V.; Pujari, S. S.; Bhosale, S. B.; et al. SILAR synthesized binder-free, hydrous cobalt phosphate thin film electrocatalysts for OER application: annealing effect on the electrocatalytic activity. Int. J. Energy. Res. 2023, 2023, 5570480.
Cite This Article

How to Cite
Download Citation
Export Citation File:
Type of Import
Tips on Downloading Citation
Citation Manager File Format
Type of Import
Direct Import: When the Direct Import option is selected (the default state), a dialogue box will give you the option to Save or Open the downloaded citation data. Choosing Open will either launch your citation manager or give you a choice of applications with which to use the metadata. The Save option saves the file locally for later use.
Indirect Import: When the Indirect Import option is selected, the metadata is displayed and may be copied and pasted as needed.
About This Article
Special Issue
Copyright
Data & Comments
Data
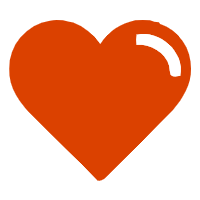
Comments
Comments must be written in English. Spam, offensive content, impersonation, and private information will not be permitted. If any comment is reported and identified as inappropriate content by OAE staff, the comment will be removed without notice. If you have any queries or need any help, please contact us at [email protected].