Challenges and modification strategies of air-processed all-inorganic CsPbX3 perovskite films for efficient photovoltaics
Abstract
All-inorganic perovskites CsPbX3 (X: halogen ions) have gained significant attention for application in next generation photovoltaic technologies due to their superior thermal stability and excellent optoelectronic properties. Compared with fabrication in N2 glove boxes, ambient air processing could simplify the operation and reduce the fabrication cost, which is favorable for boosting the commercialization of perovskite solar cells (PSCs). However, the moisture in ambient air tends to cause the phase transformation of inorganic perovskite from the photoactive black phase to the photo-inactive yellow one, thus deteriorating the photovoltaic performance. Considering the obstacles from both the intrinsic structure instability and the external atmosphere, tremendous efforts have been made for pursuing high-efficiency and stable all-inorganic PSCs that can be processed in ambient air. In this review, we first analyze the challenges for fabricating CsPbX3 in ambient air from both the intrinsic characters and external atmosphere and then overview the progress of the air-fabricated CsPbX3 films for photovoltaic applications. The recently reported various modification strategies, including the compositional/precursor, solvent, additive, and interface engineering, for achieving high-quality and stable CsPbX3 films are comprehensively summarized. Finally, a brief conclusion and outlook is given to inspire more research interest on air-fabricated CsPbX3 photovoltaics. This review provides significant guidance for further optimizing the air-processible CsPbX3 films to boost the large-scale commercialization of cost-effective PSCs in the future.
Keywords
INTRODUCTION
Metal halide perovskites have garnered great attention for application in the photovoltaic field owing to their high absorption coefficient, low exciton binding energy and long carrier lifetime[1]. Recently, a high record power conversion efficiency (PCE) of over 26% has been achieved for the perovskite solar cells (PSCs)[2], which makes them competitive with conventional crystalline silicon solar cells. Nevertheless, the organic parts in the metal halide perovskites generally show the disadvantages of easy volatilization under high temperatures (over 200 °C), severely restricting the long-term operational and thermal stability of PSCs[3,4]. In contrast, the all-inorganic CsPbX3 (X: halogen ions) perovskite materials, with the inorganic cesium ions (Cs+) completely replacing the A-site organic ions, exhibit better thermal and light stability, rendering them suitable for practical applications[5-7]. In 2015, Eperon et al. first reported all-inorganic CsPbX3 PSCs with a PCE of 2.9%[8]. Soon after, more stable CsPbI3 perovskite quantum dot (QD) solar cells were developed, with an open-circuit voltage (VOC) of 1.23 V and a PCE over 10%[9]. Recently, Wang et al. developed a defect passivation strategy for CsPbI3 films and prepared PSCs with a PCE of 21.8%, which is a record efficiency for the all-inorganic CsPbX3 PSCs so far[10]. Although all-inorganic PSCs have presented great progress in the past years, their fabrication under inert atmosphere in a glove box hinders future large-scale production. By contrast, fabricating PSCs in ambient air is drawing more and more attention because of their easy operation and low fabrication cost[11]. However, the intrinsic structural instability and the degradation induced by the external environment remain huge challenges for fabricating high-quality CsPbX3 perovskite films in ambient conditions.
The quality of photoactive perovskite layers, including uniformity, crystallinity, surface roughness, defect density, structure stability, etc., which influence the carrier transport and recombination kinetics, determines the photovoltaic performance of the PSCs. To stabilize the crystal structure and raise the quality of air-processed CsPbX3 perovskite films, many strategies have been reported, such as crystallization regulation, composition or precursor engineering, surface modification with highly hydrophobic groups, etc. With these modifications, significant achievements in PCE have been realized in recent years. The efficiency evolution of air-processed all-inorganic PSCs is shown in Figure 1A. Therefore, it is meaningful to summarize the conducive strategies for improving the quality of air-processed all-inorganic perovskites in PSCs. Herein, we mainly focus on the challenges of all-inorganic PSCs fabricated in an ambient atmosphere and the most recently developed modification strategies. First, the instability issues are discussed from both the intrinsic characteristics and extrinsic atmosphere aspects. Subsequently, we summarize the modification strategies from composition and precursor, solvent, additive introduction, and interface engineering [Figure 1B]. Finally, a brief conclusion and outlook is given.
INSTABILITY ORIGIN
Although tremendous research has been conducted and significantly improved performance has been achieved on CsPbX3 PCSs, most devices are fabricated in glove boxes with strictly controlled low moisture and oxygen content owing to the intrinsic instability and the accelerated degradation process induced by air atmosphere. Understanding the influence factors and the underlying mechanisms for the instability of perovskites is necessary to obtain high-quality perovskite films and good-performance PSCs under ambient air. This section summarizes the potential factors contributing to the instability from intrinsic characteristics and external conditions in ambient air.
Intrinsic characteristics
The ideal three-dimensional (3D) CsPbX3 crystal structure is a cubic phase with an octahedron structure[5]. As shown in Figure 2A, the cation Pb2+ is located at the center of a regular octahedron of anion X-, and the inorganic cation Cs+ is surrounded by eight lead halide [PbI6]4- octahedra. Under this circumstance, the crystal structure of CsPbX3 presents a highly symmetrical feature. However, owing to the small radius of Cs cation, the Goldschmidt tolerance factor t of CsPbX3 is lower than the organic-inorganic counterparts as t can be determined by
Figure 2. (A) Schemes of the 3D cubic CsPbX3 perovskite structure. (B) Crystal structure of CsPbI3 perovskite with different phases and their relative phase transitions. Copyright from Ref[13]. (C) Morphologies of CsPbI3 films prepared under different RH conditions before and after annealing. Copyright from Ref[20].
where rA, rB, and rX are the ionic radii for cation A, cation B, and anion X, respectively[12]. As a result, the lead iodide octahedra is prone to distort and the corner-sharing [PbI6]4- octahedra tends to rotate, lowering its symmetry. For example, three types of photoactive black crystal polymorphs are reported for CsPbI3 along decreasing the temperature: the cubic (α-, Pm3m), tetragonal (β-, P4/mbm), and orthogonal black phases
Apart from the phase instability, ion migration would destroy the perovskite lattice and degrade the optoelectronic properties. As ionic materials with low formation energies and migration activation energies, the triggered tremendous vacancies provide pathways for ion migration to the adjacent contact layers, and this process more easily occurs under heat and light. It was reported that vacancy-assisted ion migration is one of the most common processes owing to the low defect formation energy in CsPbI3[14]. Such induced iodine vacancy defects may not aggravate the non-radiative recombination as shallow-level defects are mainly formed in this case. However, it will speed up the decomposition rate of the perovskite structure. Apart from the vacancy-assisted ion migration, the grain boundaries formed during fabrication may also facilitate the ion migration of the perovskite films compared to the interior of the grains[15,16]. Therefore, decreasing the number of grain boundaries is also considered an effective way to mitigate the ion migration. Although a “self-healing” process was reported to partially suppress the ion migration[17], the device’s photovoltaic performances, especially the hysteresis and long-term stability, are still far from satisfactory.
External atmosphere
Although the stability of CsPbX3 perovskite films depends on the intrinsic characteristics, the external atmosphere, such as humid air, can accelerate the degeneration process. To develop complete air processing strategies for all-inorganic PSCs, the role of the environmental atmosphere for manufacturing CsPbX3 perovskite films should be highlighted. In this section, the instability caused by environmental factors, including water and oxygen, is discussed.
Impact of water
It is well known that water generally causes the degradation of organic-inorganic halide perovskites, which brings problems to preparing and storing PSCs[18]. Water molecules can be easily absorbed at the vacancy sites at the surface and grain boundaries of the perovskite films[19]. In a humid environment, water molecules lower the free-energy barrier of the phase transitions and the inevitable defects in the prepared CsPbX3 PSCs provide pathways for water invasion[19]. In those situations, water molecules adsorbed on CsPbX3 perovskite film would trigger the phase transition from α-phase to δ-phase and accelerate the degradation of perovskites in a humid environment.
In addition, the water molecules probably have a non-negligible influence on the crystalline structure and morphology of the CsPbX3 films during a series of thermodynamic and kinetic processes. It has been pointed out that a high humidity environment tends to result in CsPbI3 perovskite films with poor quality. Liang et al. contrasted the morphologies of the CsPbI3 films fabricated under different humidity conditions
The above experimental results demonstrate the detrimental effect of moisture on CsPbI3 perovskite films. However, an extremely low humidity environment is not a prerequisite for preparing all-inorganic perovskite films. Interestingly, some research even indicates that an appropriate amount of water could control the crystallization of all-inorganic perovskites for improving the photovoltaic performance[23].
Impact of oxygen
When processing in an open environment, oxygen would be unavoidable so its impact needs to be considered. For organic-inorganic hybrid perovskite, the degradation caused by oxygen has been reported, which involves both the deprotonation of the organic cation and the breaking of the local Pb-I octahedral structure via forming O-Pb bonds[24,25]. In contrast, for CsPbI3 with no organic components, the deprotonation of the organic components could be avoided. Tsvetkov et al. reported that the stability of inorganic perovskite can be influenced by the presence of gaseous O2, and the possible degradation pathways are as follows[26]:
This work also indicated that CsPbI3 is more sensitive to O2 and may undergo more severe degradation in the presence of O2 than CsPbCl3 and CsPbBr3. However, unlike water whose effects on all-inorganic perovskites have been widely explored, very limited reports are on the impact of oxygen[26,27]. Further studies on how oxygen influences inorganic perovskites and the underlying mechanisms still need to be investigated.
MODIFICATION STRATEGIES
Based on the above discussion, we conclude that the challenges of fabricating CsPbX3 in ambient air mainly stem from the intrinsic instability and moisture-assisted degradation. Therefore, to improve the perovskite film quality, most strategies focus on modulating the material itself and protecting the inorganic perovskite films against moisture invasion. Table 1 lists inorganic PSCs fabricated in air. This section comprehensively summarizes the recently reported effective modification strategies.
Summary of all-inorganic PSCs fabricated in air
Device structure | VOC[V] | JSC[mA cm-2] | FF [%] | PCE [%] | Fabrication condition | Ref. |
Compositional engineering | ||||||
FTO/c-TiO2/m-TiO2/CsPb0.9Sn0.1IBr2/carbon | 1.26 | 14.3 | 63 | 11.33 | RH < 90% | [28] |
FTO/TiO2/CsPbI3/spiro-OMeTAD/Au | 0.898 | 11.1 | 68 | 6.8 | RH: 0%-20% | [29] |
FTO/SnO2/CsPb0.8Ge0.2I2Br/P3HT/spiro-OMeTAD/Au | 1.27 | 12.15 | 70.1 | 10.8 | RH: - | [30] |
FTO/c-TiO2/m-TiO2/BaI2: CsPbI2Br/P3HT/Au | 1.21 | 15.45 | 79.45 | 14.85 | RH: - | [31] |
FTO/c-TiO2/m-TiO2/Cs0.99Rb0.01PbI2Br/P3HT/Au | 1.32 | 16.25 | 80.03 | 17.16 | RH: 20%-30% | [32] |
FTO/c-TiO2/m-TiO2/CsPb0.98La0.02I2Br/carbon | 1.12 | 11.66 | 61.24 | 8.03 | RH: 20%-30% | [33] |
FTO/c-TiO2/m-TiO2/ CsPb0.97Yb0.03I2Br /P3HT/Au | 1.267 | 15.94 | 76.35 | 15.41 | RH: - | [34] |
FTO/c-TiO2/m-TiO2/InCl3:CsPbI2Br/P3HT/Au | 1.334 | 16.34 | 80.1 | 17.45 | RH: - | [35] |
FTO/TiO2/InCl3:CsPbI2Br/P3HT/Au | 1.303 | 15.9 | 75.76 | 15.69 | RH: - | [22] |
FTO/c-TiO2/m-TiO2/CsPbI2.5Br0.5/P3HT/Au | 1.272 | 19.5 | 79 | 19.59 | RH: - | [36] |
Precursor engineering | ||||||
FTO/TiO2/CsPbI3/carbon | 0.79 | 18.5 | 65 | 9.5 | RH: 10%-20% | [37] |
FTO/TiO2/CsPbI2Br /PTAA/Au | 1.32 | 15.34 | 79.54 | 16.14 | RH: - | [38] |
ITO/SnO2/LiF/CsPbI2Br/PPAI/ spiro-OMeTAD/MoO3/Ag | 1.33 | 16.14 | 79.45 | 17.01 | RH: 25%-65% | [39] |
Solvent and anti-solvent engineering | ||||||
FTO/SnO2/CsPbI2Br/spiro-OMeTAD/MoO3/Ag | 1.30 | 17.67 | 74.18 | 17.10 | RH: 20%-70% | |
ITO/PEDOT:PSS/CsPbI3/ PCBM/Al | 1.05 | 18.66 | 73.3 | 14.4 | RH: - | [41] |
FTO/TiO2/CsPbI3/PTAA/Au | 1.11 | 18.31 | 77.97 | 15.91 | RH: - | [20] |
FTO/TiO2/CsPbI3/Spiro-OMeTAD/Au | 1.18 | 20.17 | 80.55 | 19.17 | RH: 60% | [42] |
Additive engineering | ||||||
FTO/c-TiO2/CsPbI3/spiro-OMeTAD/Ag | 0.66 | 11.92 | 52.47 | 4.13 | RH < 30% | [43] |
FTO/TiO2/CsPbI3/spiro-OMeTAD/Ag | 1.137 | 20.23 | 82.7 | 19.03 | RH: 5%-10% (dry box) | [44] |
FTO/SnO2/CsPbI3/spiro-OMeTAD/Au | 1.1 | 20.21 | 80.94 | 19 | RH: ~35% | [45] |
ITO/P3CT-N/CsPbI3/ PCBM/C60/BCP/Ag | 1.16 | 20.46 | 81.13 | 19.25 | RH: 40% | [46] |
ITO/P3CT-N/CsPbI3/ PCBM/C60/BCP/Ag | 1.176 | 20.1 | 80.04 | 18.93 | RH: 40% | [47] |
FTO/TiO2/CsPbI2Br/spiro-MeOTAD/Au | 1.28 | 16.56 | 81.64 | 17.26 | RH: - | [48] |
ITO/P3CT-N/CsPbI3/ PCBM/C60/BCP/Ag | 1.225 | 20.33 | 77.37 | 19.27 | RH: ~35% | [49] |
FTO/TiO2/CsPbI2Br/carbon | 1.25 | 14.47 | 80.1 | 14.49 | RH: 30%-70% | [50] |
ITO/P3CT-N/CsPbI3/ PCBM/C60/TPBi/Cu | 1.172 | 20.58 | 78.84 | 19.01 | RH: 40%-80% | [51] |
FTO/TiO2/CsPbI3/ spiro-OMeTAD/Au | 1.244 | 20.60 | 82.52 | 21.15 | annealing in air (RH: -) | [52] |
FTO/TiO2/SnO2/β-CsPbI3/γ-CsPbI3/P3HT/Au | 1.22 | 21.72 | 81.5 | 21.59 | β-CsPbI3 prepared in air (RH: 25%-30%) | [53] |
FTO/TiO2/CsPbI3/P3HT/Au | 1.16 | 20.99 | 83.1 | 20.25 | RH: - | [21] |
Interface engineering | ||||||
FTO/ZnO-ZnS/m-TiO2/CsPbI3/spiro-MeTAD/Au | 1.06 | 20.56 | 77.61 | 16.91 | RH: 10%-15% (dry box) | [54] |
FTO/TiO2/CsPbI3/MACl/ spiro-OMeTAD/Au | 1.198 | 20.59 | 82.5 | 20.37 | RH: 15%-30% | [55] |
ITO/P3CT-N/CsPbI3/ PCBM/C60/BCP/Ag | 1.213 | 20.44 | 80.37 | 19.84 | RH < 40% | [56] |
ITO/PEDOT:PSS/CsPbI3/PCBM/BCP/Ag | 1.02 | 19.12 | 79.18 | 15.45 | RH < 30% | [57] |
ITO/P3CT-N/CsPbI3/ PCBM/C60/TPBi/Ag | 1.18 | 20.84 | 81.90 | 20.18 | RH: 20%-40% | [58] |
FTO/TiO2/DCB/CsPbI3/ spiro-OMeTAD/Ag | 1.26 | 20.71 | 83.8 | 21.86 | RH: 30% | [59] |
Combined engineering | ||||||
FTO/TiO2/CsPbI3/spiro-OMeTAD/Ag | 1.11 | 20.23 | 82 | 18.4 | RH: < 10% | [60] |
ITO/PEDOT:PSS/CsPbI3/PCBM/BCP/Al | 0.95 | 19.39 | 71.57 | 13.21 | RH: < 55% | [61] |
FTO/TiO2/CsPbI3/spiro-OMeTAD/Au | 1.15 | 19.43 | 84.33 | 18.84 | RH: 30%-40% | [62] |
FTO/c-TiO2/m-TiO2/CsPb0.9Tb0.05I2.5Br0.5/P3HT/Au | 1.315 | 16.34 | 81.53 | 17.51 | RH: - | [63] |
Composition/precursor engineering
Increasing the tolerance factor of CsPbI3 by doping with other ions, e.g., a larger A-site cation, a smaller
Figure 3. (A) Schematic illustration of nucleation and crystal growth procedures of CsPb1-xTbxI2Br perovskite films and passivation by
For the A-site incorporation, it is difficult to seek an inorganic cation for substituting or partially substituting Cs+ due to its largest radius among the monovalent metals. However, Patil et al. found that Rb incorporation at the A-site helps obtain high-quality CsPbI2Br perovskite thin films with high compactness, uniform and pinhole-free morphology together with improved thermal stability, revealing that partial incorporation with Rb+ cation can stabilize black phase all-inorganic perovskites under ambient conditions[32].
Apart from the cation engineering, reasonable incorporation of relatively smaller anions in the X-site of the CsPbX3 perovskite material can also improve the structure stability. Substitution of I with Br is a mostly reported approach to increase the tolerance factor and improve the phase stability when processing in ambient air. However, the subsequently increased bandgap of the material is inevitably induced, resulting in lower light harvesting. Hence, adjusting the amount of Br doping and looking for more appropriate anions to balance the stability and the bandgap is of significant importance.
Precursor engineering is also a facile strategy for fabricating stable all-inorganic PSCs under ambient conditions. In earlier studies, the commonly used precursor solutions contain CsI and PbI2 for fabricating CsPbI3. Xiang et al. proposed a strategy to prepare air-stable α-CsPbI3 PSCs using HPbI3 to replace PbI2[37]. In 2020, Duan et al. proposed a new precursor system (HCOOCs, HPbI3, and HPbBr3) to prepare ambient-compatible, high-efficient and stable CsPbI2Br PSCs under high-humidity conditions (91% RH)
Solvent engineering
For fabricating inorganic PSCs, the most generally employed solvents are N,N-dimethylformamide (DMF) and dimethyl sulfoxide (DMSO) owing to the dissolvability of lead halides and cesium halides in other popular solvents[40]. Apart from the single DMF or DMSO solvent[31,35,64], mixed solvents of DMF and DMSO are also used, and the ratio between them has been adjusted to enhance the device performance[65,66]. In more recent work, methylammonium acetate (MAAc) was reported to be an excellent and eco-friendly solvent for CsPbX3 precursor solution in one-step humidity-insensitive fabrication[40,41]. The coordination interaction plays an important role in perovskite crystallization. In the MAAc system, the interaction is stronger than in the DMF:DMSO system due to strong N-H···I hydrogen bonds and Pb-O bonds. Wang et al. reported ambient processed CsPbI2.5Br0.5 PSCs using MAAc as a solvent, achieving a PCE of 17.10 % and VOC of 1.30 V
Figure 4. (A) Schematics of interaction in the precursor solutions using solvents of DMF: DMSO and MAAc, respectively. Copyright from Ref[40]. (B) Schematic illustration of the crystallization process for unbathed and anisole-bathed CsPbI3 perovskite films in ambient air. Copyright from Ref[62]. (C) Schematics of PHPS-containing anti-solvent treated CsPbI3 perovskite crystal growth when processing in ambient air. Copyright from Ref[42].
Owing to high boiling point of the frequently used DMF and DMSO solvents, the slow evaporation of solvent would readily cause the slow nucleation rate. It has been indicated that anti-solvents, including the widely employed chlorobenzene (CB), ethyl acetate (EA), etc., rapidly reduce the solubility of the precursor and accelerate the nucleation[67]. Adjusting the operation of anti-solvents during the spin-coating process of perovskite films can mediate the nucleation and grain growth of the perovskite films, thus modifying the morphology, film coverage and defect density[68,69]. Liang et al. conducted an anti-solvent hot substrate spin-coating method to construct air-fabricated CsPbI3 PSCs, realizing good optoelectronic properties similar to the N2-fabricated devices and a PCE of 15.91%[20]. Jeong et al. proposed an anisole antisolvent bathing strategy for preparing DMAI-assisted CsPbI3 films [Figure 4B][62]. During this process, rapid crystallization kinetics can be attributed to the formation of the Cs4PbI6-antisolvent adduct which lowers the transformation energy barrier, therefore resulting in a more uniform film with better coverage, fewer defects and pinholes. The obtained devices show a PCE of 18.84% under moisture processing conditions (30%-40% RH). Wang et al. used methyl acetate as an anti-solvent and introduced perhydropolysilazane (PHPS) into this anti-solvent to develop a curing-anti-solvent strategy for fabricating black-phase CsPbI3 perovskite films under ambient conditions [Figure 4C][42]. Under humid conditions, the hydrolyzed products of PHPS can adjust the grain growth by forming Lewis acid-base adducts. The pyrolysis-generated Si-O-Pb bonding to CsPbI3 grains can create a shield layer to hinder phase transition. The resulting PSCs show a remarkably improved PCE of 19.17%, even at RH 60%.
Additive engineering
Introducing additives into the perovskite precursor solutions can influence perovskite crystallization and film formation, passivate the defects, and tune the structure and energetics, either in N2 atmosphere or ambient air[70,71]. This section overviews the recent progress in additive engineering to improve the air-processed all-inorganic perovskite film quality and device performance.
Luo et al. first fabricated CsPbI3 PSCs under fully open-air conditions by adding HI, which improves the solubility of CsI and PbI2 precursors in DMF solution and helps CsPbI3 transform from the yellow non-perovskite to the black perovskite under low temperature[43]. After that, various additives are used to improve the air-processed film quality and the resultant effects on the PCE of devices have also been deeply investigated. DMAI is one of the most widely used additives for fabricating stable CsPbX3 and introducing HI and HPbI3 routes share the same mechanism due to the generated DMA through the reaction of HI with DMF[44,51,60,62]. Wang et al. investigated the role of DMAI in CsPbI3 perovskite films fabricated in a dry box with an accurately controlled humidity (5%~10% RH), which indicated that DMAI is a volatile additive to control the crystalline perovskite, rather than alloying into the crystal lattice of CsPbI3 perovskite
Figure 5. (A) Schematics for DMAI additive induced CsPbI3 perovskite formation and the device stability based on Cs-1.5DMAI perovskite with and without DMAI residues. Copyright from Ref[44]. (B) Schematics of hydrogen bonding-assisted CsPbI3 perovskite crystallization and the measured J-V curves of dopant-free P3HT-based CsPbI3 PSCs. Copyright from Ref[21]. (C) Schematic illustration of DMAI-assisted CsPbI3 perovskite formation and time-resolved photoluminescence decay spectra of tetragonal, coexistence phase and orthorhombic perovskite films. Copyright from Ref[72].
Based on the DMA-assisted route, Chang et al. introduced a multifunctional molecular additive Zn(C6F5)2 to obtain printable CsPbI3 films in ambient conditions [Figure 6A]. During printing, the contradiction between air-flow-assisted fast drying and low-quality film is reconciled, reducing trap density and improving energy level alignment[45]. Mali et al. fabricated β-CsPbI3 with additive Zn(C6F5)2 in ambient air with RH of 25%-30% and constructed a phase-heterojunction with γ-CsPbI3, therefore producing a 21.59% PCE for a small area-device (0.09 cm2) and an 18.43% PCE for a large area-device (18.08 cm2)[53]. Additionally, ionic liquid, with high ionic conductivity and good thermal stability[61,73,74], was used by
Figure 6. (A) Schematics of printed CsPbI3 films modified by Zn(C6F5)2. Copyright from Ref[45]. (B) Schematic illustration of defect passivation for CsPbI3 perovskites enabled by EMIMHSO4. Copyright from Ref[66]. (C) Schematic diagram of CsPbI2Br perovskite crystallization process via low-dimensional intermediate-assisted growth strategy and top-view scanning electron microscope images of the control and optimized perovskite films. Copyright from Ref[48]. (D) Schematics of the chlorination and solid protection of the perovskites with humidity-assisted strategy via Si-Cl. Copyright from Ref[47]. (E) Schematic illustration of CsPbI3 perovskite formation via humidity-assisted polymerization strategy by MMDS. Copyright from Ref[51].
Regulating both the nucleation and crystal growth kinetics is also widely reported to realize high-quality and stable all-inorganic perovskite films under ambient conditions[48,50]. Yang et al. introduced imidazole halide (IMX: IMI and IMBr) into CsPbI2Br perovskite, which can form a low-dimensional intermediate phase to help improve the film quality in ambient air [Figure 6C][48].
Besides, by introducing effective additives, Pb-related defects could be passivated, further improving the PCE and stability of PSCs. Wang et al. prepared CsPbI3 perovskite films by introducing an additive
Considering the negative effect of moisture on the performance of CsPbX3 PSCs, constructing a hydrophobic layer to protect perovskite films from water invasion is an effective way to improve the stability[46,47,49]. For instance, Fu et al. introduced 3,5-difluorobenzoic acid hydrazide (FBJ), which features a stronger absorbance on CsPbI3 than water, to regulate traps and prevent external erosions, thus realizing a high Voc of 1.225 V for inverted CsPbI3 devices[49]. Differently, humidity-assisted strategy based on hydrolysis reaction to consume water has been developed by the same group in recent years. Moisture-sensitive
Interface engineering
Interfaces between perovskites and charge transport layers play an important role in PSCs with high PCE and stability since charge carriers need to be extracted and the moisture invasion generally happens from the surface. Thereby, modifying the interface is regarded as an effective approach to reducing defects, improving the energy alignment, increasing the charge extraction rate, enhancing the stability, etc., which render the high-performance air-processed inorganic PSCs[61,63].
Wang et al. fabricated thermodynamically stabilized β-CsPbI3 and developed a crack-filling modification strategy to improve the PCE of air-processed PSCs via surface treatment with choline iodide (CHI)
Figure 7. (A) Schematics of crack-filling interface engineering and the energy diagram of the CsPbI3 layers. Copyright from Ref[60]. (B) Schematic diagram of perovskite crystallization process before and after methyl ammonium chloride treatment and the J-V curves. Copyright from Ref[55]. (C) X-ray photoelectron spectroscopy results at the surface and 10 nm depth and schematic diagram of the electron-transport dynamics based on the reference and polished films. Copyright from Ref[56].
Additionally, the morphology and crystal structure of CsPbX3 films can be regulated by intermediate treatment before annealing. Yoon et al. dropped the solution of methyl ammonium chloride (MACl) successively to control the intermediate state of the crystallization process of CsPbI3 perovskites deposited in ambient air (RH: 15%-30%) [Figure 7B][55]. It is concluded that the crystallization process is accelerated, leading to a uniform and dense film with few pinholes. Together with the post-surface passivation with octyl ammonium iodide, the efficiency of PSCs is improved to 20.37%. Recently, Li et al. comparatively investigated the influence of intermediate- and post-treatment on the PCE of air-prepared inverted CsPbI3 PSCs[57]. Using formamidinium (FA) salts for intermediate treatment, crystallization rate is accelerated, and the shortened exposure time is found beneficial for water resistance. Compared to the post-treatment method, the films finely tuned by intermediate treatment show pinhole-free morphology with reduced defects and better energy level alignment, significantly enhancing PCE and stability.
In addition to molecular passivation at the film surface, Fu et al. reported that the 1,4-butanediamine (DAB) modification could polish the intrinsically formed lead-poor surface and eliminate the nonstoichiometric components [Figure 7C][56]. Therefore, the deep-energy-level p-type traps are reduced, improving charge transfer at the CsPbI3/PCBM interface. This polishing treatment promotes the efficiency to 19.84% and improves the operational stability to 1,000 h. Recently, Guo et al. introduced N,N′-diphenylthiourea (DPhTA) to construct a gradual CsPbI3/PbS heterojunction at the top of CsPbI3 surface, thus resulting in a Voc of 1.20 V and a PCE over 20%[58].
Besides, Qiu et al. proposed a strategy via dipolar chemical bridge (DCB) to facilitate the charge transport at the perovskite/TiO2 interface[59]. By employing 4-aminobutyric acid (CA) and 3-amino-1-propanesulfonic acid (SA) at the buried interface, the interfacial energy loss is dramatically reduced and the crystallinity of perovskites in air (RH = 30%) is improved. Therefore, the optimized CsPbI3 PSCs achieve a PCE of 21.86 % with a high Voc of 1.26 V.
CONCLUSION AND OUTLOOK
All-inorganic CsPbX3 perovskites, with Cs+ completely substituting organic components, possess remarkably improved thermal and light stability compared to their organic-inorganic hybrid counterparts. Despite tremendous attention given to the all-inorganic PSCs, most of the fabrication is conducted in a glovebox with an inert atmosphere, which is unfavorable for future large-scale production and commercialization. Fabricating CsPbX3 in ambient air would help realize cost-effective PSCs to further boost the practical applications. However, the intrinsic crystal structural instability and the deterioration by ambient air of inorganic perovskite pose huge challenges to obtaining high-quality perovskite films with high crystallinity, low defect density and stable structure. In this review, the instability origin is analyzed from both the intrinsic characteristics and external atmosphere. On this basis, the strategies for improving the quality of all-inorganic perovskite films to achieve efficient and stable photovoltaic devices under ambient conditions are summarized.
Despite great progress in recent years, the PCE and stability of inorganic PSCs remain far from satisfactory and severe issues persist to be solved. For example, as the film properties and resultant device performance are significantly influenced by the ambient moisture, it is still challenging to widen the operating humidity window, which may complicate the operation procedure and consequently increase the fabrication costs. Therefore, more effective pathways are expected to optimize the optoelectronic properties and stability of CsPbX3 perovskite films under ambient conditions with high humidity. For instance, multifunctional agents, which can effectively modulate the crystallization process and simultaneously passivate the defects of air-processed CsPbX3 are required to be extensively explored. The property of water resistance still needs to be improved by developing more effective hydrophobic agents or through water-induced cross-linking strategy to consume water, especially at the film surface and grain boundaries. To further enhance the long-term stability of devices, applying dopant-free hole transport layers in regular devices is also essential. Apart from innovative techniques or agents for optimizing the CsPbX3 perovskite film quality and device performance, understanding the individual effects of moisture and oxygen deeply and systematically is crucial for further enhancing the PCE and stability of the PSCs. For example, advanced characterizations, such as in-situ Grazing Incidence Wide Angle X-ray Scattering (GIWAXS) and in-situ photoluminescence (PL) during film formation, together with operando studies on solar cells, etc., help understand the formation and degradation mechanisms under different environmental humidity or gas atmosphere. With great efforts, we believe the photovoltaic performance of CsPbX3 PSCs fabricated in ambient air will be significantly promoted, which will pave the way for low-cost production of efficient and stable PSCs.
DECLARATIONS
Authors’ contributions
Contribute equally to this work: Cao L, Tong Y
Investigation and original drafting: Cao L
Conceptualization and re-writing: Wang K
Revision: Tong Y
Discussing and commentary: Cao L, Tong Y, Wang K, Wang H
Supervision: Tong Y, Wang H, Wang K
Availability of data and materials
Not applicable.
Financial support and sponsorship
This work was financially supported by the National Natural Science Foundation of China (No. 52002327), Natural Science Foundation of Chongqing, China (CSTB2022NSCQ-MSX0926, CSTB2022NSCQ-MSX1335), and the Fundamental Research Funds for the Central Universities (G2022KY0608).
Conflicts of interest
All authors declared that there are no conflicts of interest.
Ethical approval and consent to participate
Not applicable.
Consent for publication
Not applicable.
Copyright
© The Author(s) 2024.
REFERENCES
1. Green MA, Ho-baillie A, Snaith HJ. The emergence of perovskite solar cells. Nat Photon 2014;8:506-14.
2. National Renewable Energy Laboratory. Best research-cell efficiency chart. Available from: https://www.nrel.gov/pv/cell-efficiency.html [Last accessed on 27 May 2024].
3. Park N, Grätzel M, Miyasaka T, Zhu K, Emery K. Towards stable and commercially available perovskite solar cells. Nat Energy 2016;1:16152.
4. Boyd CC, Cheacharoen R, Leijtens T, McGehee MD. Understanding degradation mechanisms and improving stability of perovskite photovoltaics. Chem Rev 2019;119:3418-51.
5. Tian J, Xue Q, Yao Q, Li N, Brabec CJ, Yip H. Inorganic halide perovskite solar cells: progress and challenges. Adv Energy Mater 2020;10:2000183.
6. Kye YH, Yu CJ, Jong UG, Chen Y, Walsh A. Critical role of water in defect aggregation and chemical degradation of perovskite solar cells. J Phys Chem Lett 2018;9:2196-201.
7. Wang K, Tong Y, Cao L, et al. Progress of inverted inorganic cesium lead halide perovskite solar cells. Cell Rep Phys Sci 2023;4:101726.
8. Eperon GE, Paternò GM, Sutton RJ, et al. Inorganic caesium lead iodide perovskite solar cells. J Mater Chem A 2015;3:19688-95.
9. Swarnkar A, Marshall AR, Sanehira EM, et al. Quantum dot-induced phase stabilization of α-CsPbI3 perovskite for high-efficiency photovoltaics. Science 2016;354:92-5.
10. Wang Z, Tian Q, Zhang H, et al. Managing multiple halide-related defects for efficient and stable inorganic perovskite solar cells. Angew Chem Int Ed 2023;62:e202305815.
11. Yan L, Huang H, Cui P, et al. Fabrication of perovskite solar cells in ambient air by blocking perovskite hydration with guanabenz acetate salt. Nat Energy 2023;8:1158-67.
12. Zhang J, Hodes G, Jin Z, Liu SF. All-inorganic CsPbX3 perovskite solar cells: progress and prospects. Angew Chem Int Ed 2019;58:15596-618.
13. Steele JA, Jin H, Dovgaliuk I, et al. Thermal unequilibrium of strained black CsPbI3 thin films. Science 2019;365:679-84.
14. Huang Y, Yin W, He Y. Intrinsic point defects in inorganic cesium lead iodide perovskite CsPbI 3. J Phys Chem C 2018;122:1345-50.
15. deQuilettes DW, Vorpahl SM, Stranks SD, et al. Solar cells. Impact of microstructure on local carrier lifetime in perovskite solar cells. Science 2015;348:683-6.
16. Shao S, Abdu-aguye M, Sherkar TS, et al. The effect of the microstructure on trap-assisted recombination and light soaking phenomenon in hybrid perovskite solar cells. Adv Funct Mater 2016;26:8094-102.
17. Cappel UB, Svanström S, Lanzilotto V, et al. Partially reversible photoinduced chemical changes in a mixed-ion perovskite material for solar cells. ACS Appl Mater Interfaces 2017;9:34970-8.
18. Bella F, Griffini G, Correa-Baena JP, et al. Improving efficiency and stability of perovskite solar cells with photocurable fluoropolymers. Science 2016;354:203-6.
19. Lin J, Lai M, Dou L, et al. Thermochromic halide perovskite solar cells. Nat Mater 2018;17:261-7.
20. Liang L, Li Z, Zhou F, et al. The humidity-insensitive fabrication of efficient CsPbI3 solar cells in ambient air. J Mater Chem A 2019;7:26776-84.
21. Li M, Wang S, Ma X, et al. Hydrogen-bonding-facilitated dimethylammonium extraction for stable and efficient CsPbI3 solar cells with environmentally benign processing. Joule 2023;7:2595-608.
22. Mali SS, Patil JV, Steele JA, Rondiya SR, Dzade NY, Hong CK. Implementing dopant-free hole-transporting layers and metal-incorporated CsPbI2Br for stable all-inorganic perovskite solar cells. ACS Energy Lett 2021;6:778-88.
23. Zhang X, Bai X, Wu H, et al. Water-assisted size and shape control of CsPbBr3 perovskite nanocrystals. Angew Chem Int Ed 2018;57:3337-42.
24. Aristidou N, Sanchez-Molina I, Chotchuangchutchaval T, et al. The role of oxygen in the degradation of methylammonium lead trihalide perovskite photoactive layers. Angew Chem Int Ed 2015;54:8208-12.
25. Aristidou N, Eames C, Sanchez-Molina I, et al. Fast oxygen diffusion and iodide defects mediate oxygen-induced degradation of perovskite solar cells. Nat Commun 2017;8:15218.
26. Tsvetkov DS, Mazurin MO, Sereda VV, Ivanov IL, Malyshkin DA, Zuev AY. Formation thermodynamics, stability, and decomposition pathways of CsPbX3 (X = Cl, Br, I) photovoltaic materials. J Phys Chem C 2020;124:4252-60.
27. Liu SC, Li Z, Yang Y, et al. Investigation of oxygen passivation for high-performance all-inorganic perovskite solar cells. J Am Chem Soc 2019;141:18075-82.
28. Liang J, Zhao P, Wang C, et al. CsPb0.9Sn0.1IBr2 based all-inorganic perovskite solar cells with exceptional efficiency and stability. J Am Chem Soc 2017;139:14009-12.
29. Jena AK, Kulkarni A, Sanehira Y, Ikegami M, Miyasaka T. Stabilization of α-CsPbI3 in ambient room temperature conditions by incorporating Eu into CsPbI3. Chem Mater 2018;30:6668-74.
30. Yang F, Hirotani D, Kapil G, et al. All-inorganic CsPb1-xGexI2Br perovskite with enhanced phase stability and photovoltaic performance. Angew Chem Int Ed 2018;57:12745-9.
31. Mali SS, Patil JV, Hong CK. Hot-air-assisted fully air-processed barium incorporated CsPbI2Br perovskite thin films for highly efficient and stable all-inorganic perovskite solar cells. Nano Lett 2019;19:6213-20.
32. Patil JV, Mali SS, Hong CK. A-site rubidium cation-incorporated CsPbI2Br all-inorganic perovskite solar cells exceeding 17% efficiency. Solar RRL 2020;4:2000164.
33. Chen S, Zhang T, Liu X, et al. Lattice reconstruction of La-incorporated CsPbI2Br with suppressed phase transition for air-processed all-inorganic perovskite solar cells. J Mater Chem C 2020;8:3351-8.
34. Patil JV, Mali SS, Park DW, Hong CK. Novel ytterbium-doped CsPbI2Br thin-films-based inorganic perovskite solar cells toward improved phase stability. Mater Today Chem 2021;22:100557.
35. Mali SS, Patil JV, Shinde PS, de Miguel G, Hong CK. Fully air-processed dynamic hot-air-assisted M:CsPbI2Br (M: Eu2+, In3+) for stable inorganic perovskite solar cells. Matter 2021;4:635-53.
36. Patil JV, Mali SS, Hong CK. Holmium rare earth metal ion incorporated and ambient-air processed all-inorganic γ-CsPbI2.5Br0.5 perovskite solar cells yielding high efficiency and stable performance. J Mater Chem A 2023;11:21312-21.
37. Xiang S, Fu Z, Li W, et al. Highly air-stable carbon-based α-CsPbI3 perovskite solar cells with a broadened optical spectrum. ACS Energy Lett 2018;3:1824-31.
38. Duan C, Cui J, Zhang M, et al. Precursor engineering for ambient-compatible antisolvent-free fabrication of high-efficiency CsPbI2Br perovskite solar cells. Adv Energy Mater 2020;10:2000691.
39. Yue Y, Yang R, Zhang W, Cheng Q, Zhou H, Zhang Y. Cesium cyclopropane acid-aided crystal growth enables efficient inorganic perovskite solar cells with a high moisture tolerance. Angew Chem Int Ed 2024;63:e202315717.
40. Wang X, Ran X, Liu X, et al. Tailoring component interaction for air-processed efficient and stable all-inorganic perovskite photovoltaic. Angew Chem Int Ed 2020;59:13354-61.
41. Saparbaev A, Zhang M, Kuvondikov V, et al. High-performance CsPbI3 perovskite solar cells without additives in air condition. Solar Energy 2021;228:405-12.
42. Wang T, Yang Q, Chen Y, et al. Stitching perovskite grains with perhydropoly(Silazane) anti-template-agent for high-efficiency and stable solar cells fabricated in ambient air. Energy Environ Mater 2023;6:e12554.
43. Luo P, Xia W, Zhou S, et al. Solvent engineering for ambient-air-processed, phase-stable CsPbI3 in perovskite solar cells. J Phys Chem Lett 2016;7:3603-8.
44. Wang Y, Liu X, Zhang T, et al. The role of dimethylammonium iodide in CsPbI3 perovskite fabrication: additive or dopant? Angew Chem Int Ed 2019;58:16691-6.
45. Chang X, Fang J, Fan Y, et al. Printable CsPbI3 perovskite solar cells with PCE of 19% via an additive strategy. Adv Mater 2020;32:e2001243.
46. Fu S, Li X, Wan J, Zhang W, Song W, Fang J. In situ stabilized CsPbI3 for air-fabricated inverted inorganic perovskite photovoltaics with wide humidity operating window. Adv Funct Mater 2022;32:2111116.
47. Fu S, Zhang W, Li X, Guan J, Song W, Fang J. Humidity-assisted chlorination with solid protection strategy for efficient air-fabricated inverted CsPbI3 perovskite solar cells. ACS Energy Lett 2021;6:3661-8.
48. Yang S, Wen J, Liu Z, et al. A key 2D intermediate phase for stable high-efficiency CsPbI2Br perovskite solar cells. Adv Energy Mater 2022;12:2103019.
49. Fu S, Sun N, Le J, et al. Tailoring defects regulation in air-fabricated CsPbI3 for efficient inverted all-inorganic perovskite solar cells with Voc of 1.225 V. ACS Appl Mater Interfaces 2022;14:30937-45.
50. Xu S, Kang C, Huang Z, et al. Dual-functional quantum dot seeding growth of high-quality air-processed CsPbI2Br film for carbon-based perovskite solar cells. Solar RRL 2022;6:2100989.
51. Lu C, Li X, Guo X, et al. Efficient inverted CsPbI3 perovskite solar cells fabricated in common air. Chem Eng J 2023;452:139495.
52. Wang J, Che Y, Duan Y et al. 21.15%-efficiency and stable γ -CsPbI3 perovskite solar cells enabled by an acyloin ligand. Adv Mater 2023;35:2210223.
53. Mali SS, Patil JV, Shao J, et al. Phase-heterojunction all-inorganic perovskite solar cells surpassing 21.5% efficiency. Nat Energy 2023;8:989-1001.
54. Chen R, Hui Y, Wu B, et al. Moisture-tolerant and high-quality α-CsPbI3 films for efficient and stable perovskite solar modules. J Mater Chem A 2020;8:9597-606.
55. Yoon SM, Min H, Kim JB, Kim G, Lee KS, Seok SI. Surface engineering of ambient-air-processed cesium lead triiodide layers for efficient solar cells. Joule 2021;5:183-96.
56. Fu S, Le J, Guo X, et al. Polishing the lead-poor surface for efficient inverted CsPbI3 perovskite solar cells. Adv Mater 2022;34:e2205066.
57. Li T, Li W, Wang K, et al. Interface engineering with formamidinium salts for improving ambient-processed inverted CsPbI3 photovoltaic performance: intermediate- vs post-treatment. ACS Appl Mater Interfaces 2023;15:51350-9.
58. Guo X, Lu C, Zhang W, et al. In situ surface sulfidation of CsPbI3 for inverted perovskite solar cells. ACS Energy Lett 2024;9:329-35.
59. Qiu J, Mei X, Zhang M, et al. Dipolar chemical bridge induced CsPbI3 perovskite solar cells with 21.86 % efficiency. Angew Chem Int Ed 2024;63:e202401751.
60. Wang Y, Dar MI, Ono LK, et al. Thermodynamically stabilized β-CsPbI3-based perovskite solar cells with efficiencies >18%. Science 2019;365:591-5.
61. Wang K, Su Z, Chen Y, et al. Dual bulk and interface engineering with ionic liquid for enhanced performance of ambient-processed inverted CsPbI3 perovskite solar cells. J Mater Sci Technol 2022;114:165-71.
62. Jeong W, Jang G, Ma S, et al. Unraveling the antisolvent bathing effect on CsPbI3 crystallization under ambient conditions. Adv Funct Mater 2022;32:2207342.
63. Mali SS, Patil JV, Rondiya SR, et al. Terbium-doped and dual-passivated γ-CsPb(I1-xBrx)3 inorganic perovskite solar cells with improved air thermal stability and high efficiency. Adv Mater 2022;34:e2203204.
64. Rico-yuson CA, Danwittayakul S, Kumar S, Hornyak GL, Bora T. Sequential dip-coating of CsPbBr3 perovskite films in ambient conditions and their photovoltaic performance. J Mater Sci 2022;57:10285-98.
65. Wang K, Gao C, Xu Z, et al. In-situ hot oxygen cleansing and passivation for all-inorganic perovskite solar cells deposited in ambient to breakthrough 19% efficiency. Adv Funct Mater 2021;31:2101568.
66. Du Y, Tian Q, Chang X, et al. Ionic liquid treatment for highest-efficiency ambient printed stable all-inorganic CsPbI3 perovskite solar cells. Adv Mater 2022;34:e2106750.
67. Dong C, Han X, Zhao Y, Li J, Chang L, Zhao W. A green anti-solvent process for high performance carbon-based CsPbI2Br all-inorganic perovskite solar cell. Solar RRL 2018;2:1800139.
68. Wang Y, Wu J, Zhang P, et al. Stitching triple cation perovskite by a mixed anti-solvent process for high performance perovskite solar cells. Nano Energy 2017;39:616-25.
69. Xu W, Ji R, Liu P, et al. In situ-fabricated perovskite nanocrystals for deep-blue light-emitting diodes. J Phys Chem Lett 2020;11:10348-53.
70. Zhang F, Zhu K. Additive engineering for efficient and stable perovskite solar cells. Adv Energy Mater 2020;10:1902579.
71. Patil JV, Mali SS, Hong CK. Reducing defects of all-inorganic γ-CsPbI2Br thin films by ethylammonium bromide additives for efficient perovskite solar cells. ACS Appl Mater Interfaces 2022;14:25576-83.
72. Jiang Y, Xu T, Du H, et al. Organic-inorganic hybrid nature enables efficient and stable CsPbI3-based perovskite solar cells. Joule 2023;7:2905-22.
73. Bai S, Da P, Li C, et al. Planar perovskite solar cells with long-term stability using ionic liquid additives. Nature 2019;571:245-50.
Cite This Article
Export citation file: BibTeX | EndNote | RIS
OAE Style
Cao L, Tong Y, Wang H, Wang K. Challenges and modification strategies of air-processed all-inorganic CsPbX3 perovskite films for efficient photovoltaics. Energy Mater 2024;4:400055. http://dx.doi.org/10.20517/energymater.2023.136
AMA Style
Cao L, Tong Y, Wang H, Wang K. Challenges and modification strategies of air-processed all-inorganic CsPbX3 perovskite films for efficient photovoltaics. Energy Materials. 2024; 4(5): 400055. http://dx.doi.org/10.20517/energymater.2023.136
Chicago/Turabian Style
Li Cao, Yu Tong, Hongqiang Wang, Kun Wang. 2024. "Challenges and modification strategies of air-processed all-inorganic CsPbX3 perovskite films for efficient photovoltaics" Energy Materials. 4, no.5: 400055. http://dx.doi.org/10.20517/energymater.2023.136
ACS Style
Cao, L.; Tong Y.; Wang H.; Wang K. Challenges and modification strategies of air-processed all-inorganic CsPbX3 perovskite films for efficient photovoltaics. Energy Mater. 2024, 4, 400055. http://dx.doi.org/10.20517/energymater.2023.136
About This Article
Special Issue
Copyright
Data & Comments
Data
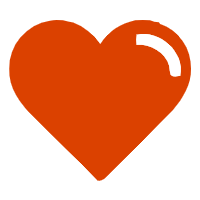
Comments
Comments must be written in English. Spam, offensive content, impersonation, and private information will not be permitted. If any comment is reported and identified as inappropriate content by OAE staff, the comment will be removed without notice. If you have any queries or need any help, please contact us at support@oaepublish.com.