Varicose vein disease in the context of insulin resistance
Abstract
The prevalence of insulin resistance (IR) is growing every year, which determines the risks of developing type 2 diabetes and cardiovascular diseases. Currently, IR is not recognized as a risk factor for the development of varicose veins (VVs), but the connection between the two is tacitly obvious because obesity and diabetes are risk factors for VVs. In this review, we have attempted to highlight the common nature of these two conditions in the context of mitochondrial dysfunction, inflammation, endothelial dysfunction, and tissue hypertrophy, and spotlight the role of IR in the development of VVs. We conclude that IR can contribute to the appearance of VVs.
Keywords
INTRODUCTION
Insulin resistance (IR) is associated with many health problems, particularly cardiovascular diseases[1,2]. IR can be triggered by genetic factors[3], but most often, it arises from an unhealthy lifestyle. Key factors in acquired IR include sedentary lifestyle and obesity, both of which are strongly associated with obesity and diabetes[4]. IR is thought to be an adaptive protective mechanism of cells against the toxic effects of nutrients[5], with its primary impact on adipose tissue, muscle tissue, and the liver[6]. The intimal layer of blood vessels is responsible for the reaction of the vascular wall to blood factors. Endotheliocytes lining the inner surface of blood vessels respond to insulin by interacting with insulin receptors on their surface[7]. IR in the context of lipid metabolism disorders is considered a cause of atherosclerosis[8,9]. Additionally, IR has been linked to thrombogenic processes[10]. It is also associated with increased blood pressure[11] and inflammatory processes in blood vessels[12,13]. Notably, IR can affect not only individuals with common risk factors like obesity and age but also lean, young individuals[14].
The connection between IR and disorders of the cardiovascular system cannot be overlooked[1,15]. Typically, when discussing IR, pathologies such as microvascular disorders, atherosclerosis, and heart disease are highlighted as the primary cardiovascular manifestations of metabolic syndrome[16]. However, an essential component of blood circulation - the venous system - is often neglected. Although insulin has been shown to reduce the risk of varicose veins (VVs)[17], there are no other literature data available on this topic. In this review, we compare the processes associated with the two conditions - IR and varicose vein disease to highlight the potential role of IR in the development of VVs. To accomplish this, in February-September 2024 we conducted keyword searches using databases such as PubMed, Google Scholar, Scopus, and Research Gate.
COMMON INFORMATION ABOUT IR
Insulin resistance is a decreased sensitivity of tissues to insulin, requiring the pancreas to secrete more insulin to maintain normal blood glucose levels[18]. IR is a common condition underlying “metabolic syndrome”[19], which encompasses IR, visceral obesity, atherogenic dyslipidemia, and endothelial dysfunction[20]. While the hyperinsulinemic-euglycemic clamp is considered the gold standard for assessing insulin resistance, its complexity often leads to the use of the HOMA-IR (homeostasis model assessment of insulin resistance) instead. The HOMA-IR index is calculated as fasting insulin (μU/dL) multiplied by fasting blood glucose (mmol/L)/22.5[21].
The prevalence of IR varies significantly, ranging from 15.5% (520 out of 3,354 Danish residents aged 19-72 years, HOMA-IR > 2.5) to 46.5% (943 out of 2,026 Venezuelan residents with a mean age of 39.69 ± 15.37 years, HOMA2-IR ≥ 2)[22]. In a study by Fahed et al., 38.0% of 286 employees at the University of Notre Dame were found to have IR (HOMA-IR ≥ 2.5)[23]. IR is associated with several conditions, such as obesity, non-alcoholic fatty liver disease, prediabetes and type 2 diabetes, and polycystic ovary syndrome[24]. In cases of prediabetes, blood glucose levels range from 110 to 125 mg/dL (with 70-99 mg/dL considered normal). While these levels are not sufficient for a diabetes diagnosis, approximately 70% of individuals with prediabetes progress to diabetes[25]. According to statistics from CDC (Centers for Disease Control and Prevention, USA), 97.6 million Americans (38.0%) over the age of 18 have prediabetes, and 38.4 million Americans (11.6% of the US population) have type 2 diabetes[26]. In 2021, the global prevalence of diabetes was estimated at 10.5% (approximately 536.6 million people), projected to reach 12.2% by 2045[27].
Insulin is a pancreatic hormone that maintains blood glucose homeostasis[28]. By activating intracellular signaling pathways, insulin promotes glycogen synthesis in the liver[28,29] and muscles[30], inhibits lipolysis[31], and facilitates protein synthesis, as well as cell growth and proliferation[32]. It is secreted by the β-cells of the pancreas in response to rising blood glucose levels until these levels normalize. Typically, cells with insulin receptors effectively respond to this signal and absorb glucose[33]. However, certain genetic pathologies[3] and unfavorable environmental factors[34] can disrupt this process. Some researchers suggest that IR may be a protective response of cells against the toxic effects of excess nutrients[5]. According to this perspective, acquired IR results from nutrient overabundance, which diminishes cellular sensitivity to insulin and disrupts the processes regulated by it. Insulin is vital for normal energy metabolism[35], wound healing[36], and the proper functioning of the nervous system[37,38] and cardiovascular system[39], as well as for lipid metabolism[31,40] and calcium homeostasis[41].
A reduced cellular response to insulin may indicate an organism’s susceptibility to diseases such as atherosclerosis[42], thromboembolism[43,44], cardiomyopathy[45], Alzheimer’s disease[46], multiple sclerosis[47], dementia[48], visual impairment[49], lipodystrophy[19], non-alcoholic fatty liver disease[50,51], rheumatoid arthritis[52,53], polycystic ovary syndrome[54], breast cancer[55], and pancreatic cancer[56]. IR is a common condition that is often diagnosed controversially[21]. The following causes of acquired IR are assumed: physical inactivity[57], obesity[58], potential hormonal changes[59], and excessive consumption of saturated fat[60]. Once type 2 diabetes develops, patients face an increased risk of cardiovascular mortality[61], and this condition is a leading cause of lower limb amputation[62]. Conditions associated with IR (such as metabolic syndrome) impact patients’ quality of life[16] and escalate healthcare costs[63,64].
COMMON INFORMATION ABOUT VVs
Chronic venous disorders include the entire spectrum of morphological and functional disorders within the venous system. Chronic venous disease (CVeD) refers to long-term morphological and functional disorders that manifest through symptoms and/or signs necessitating examination and/or care[65]. The prevalence of CVeD is high; a review by Salim et al. analyzed pooled data from 19 studies across different continents, revealing the following prevalence rates of clinical classes (according to the CEAP classification[66]): C0 (no visible or palpable signs of venous disease): 9%, C1 (telangiectasias or reticular veins): 26%, C2 (VVs): 19%, C3 (edema): 8%, C4 (skin and subcutaneous tissue changes secondary to chronic venous disease): 4%, C5 (healed venous ulcers): 1%, and C6 (active venous ulcers): 0.42%[67]. VVs are characterized by twisted and dilated veins in the lower extremities. If left untreated, this condition can lead to complications such as superficial vein thrombosis, deep vein thrombosis, and venous ulcers[68]. The progression rate of stage C2 is 22%, while the annual incidence of VVs ranges from 0.2% to 2.3%[69]. Classes C3-C6 are designated as chronic venous insufficiency, which indicates advanced CVeD characterized by functional disorders of the venous system, leading to swelling, skin changes, or venous ulcers[65]. In this review, the term VVs encompasses not only the C2 class of CVeD but also represents the broader concept of varicose transformation of the veins in the lower extremities.
Blood flow from the lower extremities is facilitated by a complex system of veins, which function effectively due to the presence of bicuspid valves, muscle contraction that counteracts gravity, and high hydrostatic venous pressure (up to 100 mm Hg)[70]. The venous wall consists of three layers[71]: the tunica (t.) intima - the inner layer, composed of endothelial cells that line the basement membrane. This layer is in direct contact with the blood and ensures selective permeability of the venous wall. The t. media - the middle layer, made up of smooth muscle cells (SMCs), which regulate pressure within the vein lumen. The
Risk factors for VVs include family history (genetic factors), advanced age, female gender, pregnancy, obesity, physical inactivity, smoking, hypertension, hormone replacement therapy/oral contraceptives, diabetes mellitus, orthopedic injuries, lifestyles characterized by prolonged standing or sedentary behavior, as well as superficial vein thrombosis and low fiber intake[73-75]. The researchers highlight the connections between type 2 diabetes (characterized by impaired glucose tolerance and insulin resistance) and CVeD, including thrombogenesis, proinflammatory state, collagen structure disorders, and endothelial dysfunction[76,77]. Studies indicate that patients with CVeD are twice as likely to have diabetes mellitus compared to the general population; however, a higher incidence of more severe stages of CVeD (specifically C5 and C6, according to the CEAP) has not yet been established[76]. A cohort study in Taiwan showed that the overall incidence of venous thromboembolism is higher in patients with diabetes than in the general population, with these patients also facing a higher risk of developing deep vein thrombosis and pulmonary embolism[78].
The additive genetic component of CVeD is 17.3%[79], suggesting that other risk factors contribute 82.7% to the development of the disease. Additionally, the influence of factors such as gender and age on the progression of clinical status is estimated to account for 10.7%[79].
MITOCHONDRIA AND CALCIUM METABOLISM IN IR AND VVs
Mitochondrial dysfunction significantly contributes to IR[80]. Researchers relate IR to disruptions in mitochondrial dynamics and a decrease in the number of functional mitochondria[81], which are provoked by the changes in mitochondrial calcium homeostasis[82]. Endoplasmic reticulum stress, potentially arising from hypoxia or oxidative damage, leads to calcium release, causing cell death or, if the stimulus is insufficient, mitochondrial dysfunction[83]. In IR, apoptotic processes are intensified[84] and provoked by fatty acids (FAs) metabolites (such as diacylglycerol and ceramide)[85], alongside an observed accumulation of Ca2+ in the cytoplasm, disrupting mitochondrial dynamics[81]. Mitochondria typically undergo fission, a process that allows for the removal of dysfunctional parts of these organelles through mitophagy. However, in the case of IR, dysfunctional mitochondria persist, hindering the development of new, healthy mitochondria. Mitochondrial fission reduces the activity of the p38 MAPK pathway and increases the activity of insulin receptor substrate 1 (IRS-1) and AKT serine/threonine kinase 1 (AKT). In this case, biogenesis is disrupted and the formation of new competent mitochondria is reduced, likely linked to reduced expression of PGC-1α, a transcription coactivator that regulates genes involved in energy metabolism[81].
Due to a decrease in insulin’s ability to regulate lipolysis, FAs accumulate in the inner mitochondrial membrane, leading to their oxidation in peroxisomes and microsomes. In turn, increases the production of reactive oxygen species (ROS)[85], which is presumed to cause IR[86]. When energy substrates are in excess during IR, cells experience a deficiency in adenosine triphosphates (ATPs). This reduction in ATP levels during IR is probably associated with an adaptive thermoregulatory mechanism activated by excess FAs in the body, which favors heat production over ATP synthesis (uncoupling of the oxidation and phosphorylation processes)[85].
We have demonstrated that in varicose veins, compared to non-varicose veins, the amount of mitochondrial DNA is decreased, and the mitochondrial membrane potential is reduced[87]. A single mitochondrion can contain multiple copies of DNA; however, the overall amount of mitochondrial DNA is generally indicative of the number of mitochondria within a cell. These findings suggest an impairment in both mitochondrial biogenesis and function in VVs, potentially classifying VVs as a form of secondary mitochondrial dysfunction. Additionally, differential expression of several genes regulating the mitochondrial function has been observed in VVs. For example, a decrease in OXA1L gene mRNA levels has been reported in VVs compared to non-varicose veins[88]. The OXA1L protein participates in the assembly of mitochondrial respiratory chain complexes by inserting proteins encoded by mitochondria and the nucleus into the mitochondrial membrane. This process is required for the oxidative phosphorylation mechanism[89,90]. Furthermore, an increase in the expression of the BCL-2 gene and a decrease in the expression of the BAX gene at the mRNA and protein levels have been observed in varicose SMCs compared to non-varicose SMCs[91]. The BCL-2 protein is localized in the membranes of mitochondria, endoplasmic reticulum, and the nucleus, where it helps preventing oxidative damage. Conversely, the BAX protein interacts with the mitochondrial voltage-dependent anion channel, leading to a loss of the membrane potential and the release of cytochrome C[92]. The BCL-2/BAX ratio determines cell survival or death after an apoptotic stimulus[93]. The absence of differential expression of each of those genes in whole VV segments (regardless of cell type related to a certain layer of the vein wall)[88] may reflect their multidirectional expression in different cell types. In patients with polycystic ovary syndrome (the condition related to IR), who did not receive growth hormone treatment, the expression of the BAX and BCL-2 genes in granulosa cells is increased and decreased, respectively[94]. Furthermore, BNIP-3 gene expression at the mRNA level was shown to be increased in VVs compared to non-varicose veins[95]. BNIP-3 is a mitochondrial protein and a proapoptotic factor associated with mitochondrial dysfunction[96].
The loci rs2911463 (PIEZO1), rs2861819 (PPP3R1), and rs28558138 (STIM2) are associated with VVs[97]. PIEZO1 is a cation channel activated by a mechanical stimulus: tension or shear stress. Its activation has been shown to enhance mitochondrial respiration and glycolysis in endothelial cells, stimulating ATP production[98]. In addition, PIEZO1 has been implicated in the regulation of insulin sensitivity[99]. PPP3R1 plays an important role in the adaptive regulation of body weight and energy metabolism to a high-fat, high-sugar diet and exercise[100]. It has also been found to increase Ca2+ influx, which promotes the aging of mesenchymal stem cells[101] and is involved in the pathogenesis of Alzheimer’s disease[102], a condition linked to IR condition. STIM2 is a key regulator of cytosolic calcium concentration[103] and is also involved in the development of Alzheimer’s disease[104].
We cannot definitely claim that mitochondrial dysfunction in VVs is directly related to IR, but both conditions (IR and VVs) share similarities in mitochondrial biogenesis, calcium homeostasis, and energy metabolism.
INFLAMMATION IN IR AND VVs
Inflammation is a key indicator and contributing factor of IR[105]. One of the primary signs of IR is inflammation within adipose tissue, where hypertrophy and hyperplasia are accompanied by the secretion of cytokines and increased recruitment of immune cells[106]. Obesity-induced lipid accumulation activates the JNK and NF-kB pathways[107], leading adipocytes to secrete proinflammatory cytokines such as CCL2[108], TNF-α[109], and IL-6[110]. High levels of CCL2 promote the infiltration of monocytes into adipose tissue, where these cells differentiate into adipose tissue macrophages[111]. In turn, macrophages secrete TNF-α, IL1β, IL-6, and IL-8, which contribute to increased lipolysis (release of FAs), induction of matrix metalloproteinases (MMPs), and inhibition of type 2 collagen synthesis[112,113]. Beyond macrophages, various other immune cells, including mast cells, neutrophils, CD-34 cells, B cells, Th1, and CD8 T cells, are implicated in IR[106]. In a human induced pluripotent stem cell model of hepatic IR, TNFα and IL1β have been shown to promote inflammation and IR[114]. TNF-α increases serine phosphorylation of IRS1/2, which reduces GLUT4 expression[115] and increases IL-6 secretion. IL-6, in turn, inhibits the transcription of the IRS-1, GLUT-4 and PPAR-γ (peroxisome proliferator-activated receptor gamma) genes, thereby impairing insulin-stimulated glucose transport. IL-6 expression in fat cells increases approximately 15-fold during IR[113]. In human skeletal muscle myoblasts, IL-6 has been found to enhance the expression of Toll-like receptor 4 (TLR-4), which triggers the innate immune response through STAT3 activation[116]. Interestingly, the inhibition of PIEZO1 in mouse adipocytes has been shown to promote TLR4-mediated inflammation and induce IR[99]. A pathway for hepatic IR has been proposed, in which STAT3 is activated through mTOR, which, in turn, increases the expression of SOCS3 (suppressor of cytokine signaling 3), promoting the inhibition of insulin signaling[117]. Moreover, IFNγ has been identified as a type 2 diabetes-specific atherogenic factor that suppresses the antiatherogenic proteins APOE and C3, predisposing macrophages to increased cholesterol accumulation[118].
Inflammation in the venous wall can occur due to the activation of endothelial cells, triggered by changes in shear stress and the onset of initial inflammatory processes. In response, the endothelium increases the adhesion and migration of leukocytes through the venous wall[119]. Compared to normal endothelial cells, venous endothelial cells from patients with VVs show increased expression of cell surface adhesion molecules, such as CD146 and ICAM-1[120]. Interestingly, in patients with diabetes and obesity who lost weight through exercise and a balanced low-calorie diet, the levels of adhesion molecules ICAM-1 and VCAM-1 decrease[121]. It has also been demonstrated that senescent human umbilical vein endothelial cells treated with serum from patients with VVs produce increased amounts of ICAM-1, VCAM-1, P-selectin, uPA, PAI-1, and ET-1 via TGF-β[122]. PAI-1, a fibrinolysis inhibitor, is elevated in IR due to visceral fat accumulation, and this effect may be further amplified by TNF-α and TGF-β. Elevated PAI-1 levels are also linked to atherothrombosis in the context of IR[123]. ICAM-1, a cell surface glycoprotein on endothelial cells, is activated in response to inflammatory stimuli, facilitating leukocyte transmigration across the endothelium. ICAM-1 expression is induced by TNF-α or IL-1β[124]. VCAM-1 also mediates the binding of leukocytes to the endothelium, which may be enhanced by increased levels of circulating cytokines (TNF-α, IL-6) and lipoproteins[13]. Leukocytes adhering to the vein wall release TGF-β and proinflammatory cytokines, triggering pathological cascades[119]. TGF-β regulates cell proliferation, differentiation, and growth, and plays a role in diabetic fibrosis across various tissues[125]. Blood samples from the site of VVs show significantly increased concentrations of IL-6, IL-8, and CCL2, suggesting that the surrounding tissue (including fat) may activate inflammation in the vein wall[126].
In addition to the aforementioned information, an increase in the expression of the CX3CR1 gene[88] and a decrease in the expression of the IL-8 gene[127], which is associated with inflammation, were observed in VVs. CX3CR1 is a transmembrane protein and chemokine involved in leukocyte adhesion and migration, and its expression is elevated in diabetic patients[128]. IL-8, a key mediator of the inflammatory response that directs neutrophils to infection sites, plays a role in the endothelial dysfunction observed in IR[129]. There is also a genetic link between VVs and inflammation, involving the previously mentioned variants: rs2861819 (PPP3R1), rs11135046 (EBF1), rs9880192 (GATA2), and rs12625547 (NFATC2)[97]. EBF1 is a transcription factor that stimulates B cell differentiation and is associated with cardiovascular and metabolic risk[130]. GATA2, another transcription factor, is involved in hematopoiesis and the regulation of endocrine cell lines. Activation of the Wnt pathway in human adipocytes has been shown to induce GATA2 expression, while reducing GLUT4 expression, which characterizes IR[131]. NFATC2, a nuclear factor expressed by activated T cells, plays a critical role in the induction of gene transcription during immune responses. In TLR4 knockout models, NFATC2 promotes mitochondrial metabolic reprogramming through its translocation to mitochondria, potentially alleviating oxidative stress and chronic inflammation during IR[132].
ENDOTHELIAL DYSFUNCTION IN IR AND VVs
The endothelium, which lines the entire inner surface of blood vessels, plays a crucial role in regulating blood circulation. Its functions include maintaining the barrier between the bloodstream and surrounding tissues, controlling vascular tone, regulating hemostasis, promoting the formation of new blood vessels, facilitating hormone transport, and recruiting neutrophils. Endothelial dysfunction is marked by decreased vasodilation and increased proinflammatory and prothrombic properties. The damaged endothelium becomes excessively permeable, allowing harmful substances (such as toxins, etc.) to infiltrate the surrounding tissues[133].
The activation of insulin receptors on the surface of endotheliocytes is necessary to regulate the function of these cells. Insulin controls blood flow and facilitates its delivery to peripheral tissues[134,135] through the activation of AKT, which subsequently activates endothelial nitric oxide synthase (eNOS)[7]. Nitric oxide (NO), produced by eNOS, is an endogenous vasodilator and inhibitor of thrombogenesis[136], giving insulin vasodilatory properties. Additionally, insulin stimulates angiogenesis and promotes wound healing by stimulating the secretion of vascular endothelial growth factor (VEGF)[137,138], and prevents endothelial cell apoptosis[139]. Studies on murine knockouts of the insulin receptor gene have shown a significant increase in baseline systolic blood pressure and impaired natriuresis[140], indicating the role of IR in arterial hypertension. High blood pressure triggers a proinflammatory and prothrombic state in the endothelium, increases vascular stiffness, and heightens the number and activation of platelets[141]. IR increases the tendency to thrombus formation[10]; endothelial dysfunction provoked by IR can lead to an increased level of circulating PAI-1 in plasma due to its enhanced synthesis in endothelial cells. In addition to being highly correlated with IR, elevated levels of circulating PAI-1 predict the development of diabetes[142]. It is worth noting that deep vein thrombosis is a known risk factor for the development of VVs[143]. Furthermore, IR in other tissues negatively affects endothelial function. For example, IR in adipose tissue is associated with dyslipidemia (an abnormal increase in plasma levels of free fatty acids and triglycerides) characterized by an imbalance between high-density lipoprotein and low-density lipoprotein (LDL), favoring the latter[144]. LDL, when oxidized through interaction with ROS, binds to lectin-like oxidized LDL receptor-1 on the surface of endothelial cells and is absorbed, which enhances the production of MMPs and the adhesion of leukocytes to the endothelium, and downregulates eNOS[1].
In the previous chapter, we discussed endothelial dysfunction in VVs, which is directly related to inflammation. Normally, laminar shear stress enhances the expression and activity of eNOS, leading to increased NO production, which, among other effects, inhibits SMC proliferation. VVs are characterized by reduced shear stress that contributes to endothelial dysfunction and pathological remodeling of the venous wall[145]. Our recent study demonstrated that oscillatory shear stress on the endothelium results in epigenome-wide methylation changes in endothelial cells and other cell types in adjacent layers of the venous wall[146]. Additionally, the hypoxic environment caused by blood stagnation negatively affects the venous endothelium. It has been shown that HIF-1α and HIF-2α genes encoding the α-subunits of hypoxia-inducible factors are upregulated in VVs[95]. HIF-α is elevated in response to decreased oxygen concentrations. Increased expression of HIF target genes - GLUT-1, CA9, BNIP-3, and VEGF - has also been reported[95]. While VEGF normally supports endothelial function and regulates vascular growth, its dysregulation, with abnormally high levels, leads to aberrant angiogenesis[147]. In addition to endothelial damage in VVs, neoangiogenesis is commonly observed[148]. We assume that high circulating insulin levels may also contribute to the upregulation of VEGF in VVs, as a higher HOMA-IR index is predictive of increased serum VEGF levels[149]. Furthermore, studies indicate that VVs exhibit elevated levels of VEGF-A (mRNA and protein) and increased expression of the VEGFR2 gene encoding the VEGF receptor, which may be a marker of, or lead to, abnormal extracellular matrix metabolism[150,151]. In VVs, increased mRNA levels of genes encoding proteins from the cellular communication network family -CCN1, CCN2, and CCN5 - have been reported[88]. Members of this family play a regulatory role and are involved in endothelial cell adhesion. Conversely, decreased expression of the VCL gene, which encodes a protein that protects VE-cadherin junctions in endothelial cells and strengthens the endothelial barrier[127,152]. Additionally, the rs247749 locus associated with the AGGF1 gene, which encodes an angiogenic factor promoting endothelial cell proliferation, has been identified as a genetic risk factor for VVs[153]. Notably, overexpression of Aggf1 has been demonstrated to lead to hepatic steatosis in mice, causing IR[154].
TISSUE HYPERTROPHY IN IR AND VVs
Normally, insulin activates and maintains the balance of two signaling pathways: PI3K-AKT (metabolic arm: glucose transport, protection against apoptosis, oxidative stress and inflammation, inhibition of lipolysis, glycogen and protein synthesis) and MAPK (mitogenic arm: cell growth, hypertrophy and fibrosis, inflammation). However, in the presence of IR, this balance shifts toward the mitogenic arm, leading to disturbances in the cardiovascular system[155]. Both the PI3K-AKT and MAPK pathways are upregulated in patients with venous reflux[74]. Thus, the processes associated with VVs may be linked to both IR of the venous endothelium itself and the hyperinsulinemia caused by IR in other tissues.
There is a connection between cardiac hypertrophy and IR[156], as well as between IR and the hypertrophy and hyperplasia of adipose tissue[157]. VVs are characterized by hypertrophy (with local atrophy) of the venous wall, with disorganization of the t. media, thickening of the t. intima, and the transition of SMCs to a synthetic and proliferative phenotype[158]. Surendran et al. demonstrated hypertrophy and hyperplasia of SMCs in VVs, which was associated with upregulation of the FoxC2-Dll4 pathway[159]. Additionally, evidence indicates that insulin can increase the expression of the FOXC2 protein in mesenchymal stem cells, which regulates the expression of genes associated with IR (GLUT4, PAI-1, UCP-1)[160]. In VVs, there is an increased expression of genes involved in proliferation, including TIMP1, TMEM158, CHRDL2, EFEMP1, and TGF-β[127]. Levels of Timp1 (tissue inhibitor of matrix metalloproteinase 1) have been found to be elevated in the serum and adipose tissue of diet-induced obese mice[161]. TGF-β plays a crucial role in the pathogenesis of VVs, serving as a link between inflammation and remodeling of the venous wall[74]. However, the study by Bruczko-Goralewska et al. reported a significant reduction in TGF-β protein levels in VVs compared to veins of patients with chronic limb ischemia[151]. TGF-β disrupts the regulation of fibronectin and collagen, MMPs and their tissue inhibitors (TIMPs)[74], and influences the expression and activity of growth factors. An imbalance in the MMPs/TIMPs ratio results in decreased elasticity and increased distensibility of the venous wall[162]. In obesity, regulation of MMPs and TIMPs in adipose tissue is disrupted, which plays a significant role in metabolic disorders. Notably, membrane-type 1 matrix metalloproteinase can modulate insulin sensitivity by cleaving insulin receptors[163].
We observed an increase in the expression of the MFAP5 gene, which encodes a microfibril-associated component of the extracellular matrix, in VVs. This increase correlated with the hypomethylated status of two loci located in the regulatory regions of this gene[88]. Among other layers of the venous wall investigated, the t. intima appeared to be the main contributor to hypomethylation at one of these loci in VVs[164]. MFAP5 is known to bind growth factors (TGFβ, BMP)[165]; it promotes angiogenesis[166], enhances the stabilization of type 1 procollagen[167], and correlates with IR markers in adipose tissue[168]. Additionally, perivascular adipose tissue (PVAT), which provides mechanical support and is in close contact with the
CONCLUSION
In this review, we have highlighted the evident connection between VVs and IR in processes such as mitochondrial dysfunction, inflammation, endothelial dysfunction, and tissue hypertrophy. Consequently, we conclude that IR can contribute to the development of VVs. We believe this original insight will draw researchers’ attention to these interrelations and may lead to meaningful concepts. In our opinion, future research on this topic could be performed in a wide variety of scientific fields, including epidemiology, molecular genetics, physiology, and clinical investigations. Ultimately, this research could: (1) enable clinicians to take appropriate and timely measures for patients with VVs (which, in fact, can serve as markers of existing IR, thereby potentially alleviating symptoms of CVeD) to early diagnose IR and reduce the possible risks of further complications; and (2) empower patients to (a) prevent the development of metabolic syndrome by controlling circulating insulin levels; and (b) reduce the risk of VVs by making lifestyle adjustments.
DECLARATIONS
Authors’ contributions
Made substantial contributions to the conception, conceptualization, and design of the study: Korolenya V, Smetanina M, Filipenko M
Performed literature data acquisition and analysis; wrote the manuscript (original draft): Korolenya V
Wrote the manuscript (review & editing): Korolenya V, Smetanina M
Provided administrative, technical, and material support: Smetanina M, Filipenko M
Availability of data and materials
Not applicable.
Financial support and sponsorship
This work was supported by the Program of Fundamental Scientific Research of the Russian Federation (PFSR RF) “Fundamental Basics of Health Preservation” (No. 121031300045-2).
Conflicts of interest
All authors declared that there are no conflicts of interest.
Ethical approval and consent to participate
Not applicable.
Consent for publication
Not applicable.
Copyright
© The Author(s) 2024.
REFERENCES
1. Kosmas CE, Bousvarou MD, Kostara CE, Papakonstantinou EJ, Salamou E, Guzman E. Insulin resistance and cardiovascular disease. J Int Med Res 2023;51:3000605231164548.
2. Freeman AM, Acevedo LA, Pennings N. Insulin resistance. StatPearls; 2024. Available from: https://www.ncbi.nlm.nih.gov/books/NBK507839/ [Last accessed on 19 Oct 2024].
3. Brown AE, Walker M. Genetics of insulin resistance and the metabolic syndrome. Curr Cardiol Rep 2016;18:75.
5. Nolan CJ, Ruderman NB, Kahn SE, Pedersen O, Prentki M. Insulin resistance as a physiological defense against metabolic stress: implications for the management of subsets of type 2 diabetes. Diabetes 2015;64:673-86.
7. Zeng G, Nystrom FH, Ravichandran LV, et al. Roles for insulin receptor, PI3-kinase, and Akt in insulin-signaling pathways related to production of nitric oxide in human vascular endothelial cells. Circulation 2000;101:1539-45.
8. Cai X, Sun J, Jin A, et al. Association of insulin resistance with intra- and extra-cranial atherosclerotic burden in the nondiabetic community population. Neurobiol Dis 2023;186:106268.
9. Min J, Weitian Z, Peng C, et al. Correlation between insulin-induced estrogen receptor methylation and atherosclerosis. Cardiovasc Diabetol 2016;15:156.
10. Barale C, Russo I. Influence of cardiometabolic risk factors on platelet function. Int J Mol Sci 2020;21:623.
11. da Silva AA, do Carmo JM, Li X, Wang Z, Mouton AJ, Hall JE. Role of hyperinsulinemia and insulin resistance in hypertension: metabolic syndrome revisited. Can J Cardiol 2020;36:671-82.
12. Olefsky JM, Glass CK. Macrophages, inflammation, and insulin resistance. Annu Rev Physiol 2010;72:219-46.
14. Šebeková K, Gurecká R, Csongová M, Koborová I, Repiská G, Podracká Ľ. Lean insulin-resistant young adults display increased cardiometabolic risk: a retrospective cross-sectional study. Diabetes Res Clin Pract 2022;185:109217.
15. Stewart AJ, Tuncay E, Pitt SJ, Rainbow RD. Editorial: insulin resistance and cardiovascular disease. Front Endocrinol 2023;14:1266173.
16. Tune JD, Goodwill AG, Sassoon DJ, Mather KJ. Cardiovascular consequences of metabolic syndrome. Transl Res 2017;183:57-70.
17. Huang K, Shen R, Chen Q, et al. Insulin product decreases risk of varicose vein: evidence from a mendelian randomization study. Vasc Investig Ther 2021;4:75-82.
18. Lee SH, Park SY, Choi CS. Insulin resistance: from mechanisms to therapeutic strategies. Diabetes Metab J 2022;46:15-37.
19. Angelidi AM, Filippaios A, Mantzoros CS. Severe insulin resistance syndromes. J Clin Invest 2021;131:142245.
21. Tahapary DL, Pratisthita LB, Fitri NA, et al. Challenges in the diagnosis of insulin resistance: focusing on the role of HOMA-IR and tryglyceride/glucose index. Diabetes Metab Syndr 2022;16:102581.
22. Bermudez V, Salazar J, Martínez MS, et al. Prevalence and associated factors of insulin resistance in adults from maracaibo city, venezuela. Adv Prev Med 2016;2016:9405105.
23. Fahed M, Abou Jaoudeh MG, Merhi S, et al. Evaluation of risk factors for insulin resistance: a cross sectional study among employees at a private university in Lebanon. BMC Endocr Disord 2020;20:85.
24. Thomas DD, Corkey BE, Istfan NW, Apovian CM. Hyperinsulinemia: an early indicator of metabolic dysfunction. J Endocr Soc 2019;3:1727-47.
25. Alvarez S, Coffey R, Mathias PM, Algotar AM. Prediabetes. StatPearls; 2024. Available from: https://www.ncbi.nlm.nih.gov/books/NBK459332/ [Last accessed on 19 Oct 2024].
26. Center for Disease Control and Prevention. National diabetes statistics report. Available from: https://www.cdc.gov/diabetes/php/data-research/index.html [Last accessed on 19 Oct 2024].
27. Sun H, Saeedi P, Karuranga S, et al. IDF diabetes Atlas: global, regional and country-level diabetes prevalence estimates for 2021 and projections for 2045. Diabetes Res Clin Pract 2022;183:109119.
28. Holst JJ, Gasbjerg LS, Rosenkilde MM. The role of incretins on insulin function and glucose homeostasis. Endocrinology 2021;162:bqab065.
30. Bouskila M, Hirshman MF, Jensen J, Goodyear LJ, Sakamoto K. Insulin promotes glycogen synthesis in the absence of GSK3 phosphorylation in skeletal muscle. Am J Physiol Endocrinol Metab 2008;294:E28-35.
31. Sancar G, Liu S, Gasser E, et al. FGF1 and insulin control lipolysis by convergent pathways. Cell Metab 2022;34:171-83.e6.
32. Haeusler RA, McGraw TE, Accili D. Biochemical and cellular properties of insulin receptor signalling. Nat Rev Mol Cell Biol 2018;19:31-44.
33. Röder PV, Wu B, Liu Y, Han W. Pancreatic regulation of glucose homeostasis. Exp Mol Med 2016;48:e219.
34. Flores-Viveros KL, Aguilar-Galarza BA, Ordóñez-Sánchez ML, et al. Contribution of genetic, biochemical and environmental factors on insulin resistance and obesity in Mexican young adults. Obes Res Clin Pract 2019;13:533-40.
35. Guo CA, Guo S. Insulin receptor substrate signaling controls cardiac energy metabolism and heart failure. J Endocrinol 2017;233:R131-43.
36. Wang J, Xu J. Effects of topical insulin on wound healing: a review of animal and human evidences. Diabetes Metab Syndr Obes 2020;13:719-27.
37. Craft S, Baker LD, Montine TJ, et al. Intranasal insulin therapy for Alzheimer disease and amnestic mild cognitive impairment: a pilot clinical trial. Arch Neurol 2012;69:29-38.
38. Dodd GT, Tiganis T. Insulin action in the brain: roles in energy and glucose homeostasis. J Neuroendocrinol 2017;29:e12513.
39. Salt IP. Examining the role of insulin in the regulation of cardiovascular health. Future Cardiol 2013;9:39-52.
40. Smith GI, Shankaran M, Yoshino M, et al. Insulin resistance drives hepatic de novo lipogenesis in nonalcoholic fatty liver disease. J Clin Invest 2020;130:1453-60.
42. Di Pino A, DeFronzo RA. Insulin resistance and atherosclerosis: implications for insulin-sensitizing agents. Endocr Rev 2019;40:1447-67.
43. Van Schouwenburg IM, Mahmoodi BK, Veeger NJ, et al. Insulin resistance and risk of venous thromboembolism: results of a population-based cohort study. J Thromb Haemost 2012;10:1012-8.
44. Vayá A, Martínez-Triguero ML, España F, Todolí JA, Bonet E, Corella D. The metabolic syndrome and its individual components: its association with venous thromboembolism in a Mediterranean population. Metab Syndr Relat Disord 2011;9:197-201.
45. Jia G, Whaley-Connell A, Sowers JR. Diabetic cardiomyopathy: a hyperglycaemia- and insulin-resistance-induced heart disease. Diabetologia 2018;61:21-8.
46. Sędzikowska A, Szablewski L. Insulin and insulin resistance in Alzheimer's disease. Int J Mol Sci 2021;22:9987.
47. Ayromlou H, Hosseini S, Khalili M, et al. Insulin resistance is associated with cognitive dysfunction in multiple sclerosis patients: a cross-sectional study. J Neuroendocrinol 2023;35:e13288.
48. Kim HK, Song J. Hypothyroidism and diabetes-related dementia: focused on neuronal dysfunction, insulin resistance, and dyslipidemia. Int J Mol Sci 2022;23:2982.
49. Faiq MA, Sengupta T, Nath M, et al. Ocular manifestations of central insulin resistance. Neural Regen Res 2023;18:1139-46.
50. Tanase DM, Gosav EM, Costea CF, et al. The intricate relationship between type 2 diabetes mellitus (T2DM), insulin resistance (IR), and nonalcoholic fatty liver disease (NAFLD). J Diabetes Res 2020;2020:3920196.
51. Marušić M, Paić M, Knobloch M, Liberati Pršo AM. NAFLD, insulin resistance, and diabetes mellitus type 2. Can J Gastroenterol Hepatol 2021;2021:6613827.
52. Giles JT, Danielides S, Szklo M, et al. Insulin resistance in rheumatoid arthritis: disease-related indicators and associations with the presence and progression of subclinical atherosclerosis. Arthritis Rheumatol 2015;67:626-36.
53. Diaz-Rubio GI, Corona-Meraz FI, Madrigal-Ruiz PM, et al. CCR2/CCL2 and CMKLR1/RvE1 chemokines system levels are associated with insulin resistance in rheumatoid arthritis. PLoS One 2021;16:e0246054.
54. Zhao H, Zhang J, Cheng X, Nie X, He B. Insulin resistance in polycystic ovary syndrome across various tissues: an updated review of pathogenesis, evaluation, and treatment. J Ovarian Res 2023;16:9.
55. Rose DP, Vona-Davis L. The cellular and molecular mechanisms by which insulin influences breast cancer risk and progression. Endocr Relat Cancer 2012;19:R225-41.
56. Toledo FGS, Chari S, Yadav D. Understanding the contribution of insulin resistance to the risk of pancreatic cancer. Am J Gastroenterol 2021;116:669-70.
57. Pierre N, Appriou Z, Gratas-Delamarche A, Derbré F. From physical inactivity to immobilization: dissecting the role of oxidative stress in skeletal muscle insulin resistance and atrophy. Free Radic Biol Med 2016;98:197-207.
58. Racette SB, Evans EM, Weiss EP, Hagberg JM, Holloszy JO. Abdominal adiposity is a stronger predictor of insulin resistance than fitness among 50-95 year olds. Diabetes Care 2006;29:673-8.
59. De Paoli M, Zakharia A, Werstuck GH. The role of estrogen in insulin resistance: a review of clinical and preclinical data. Am J Pathol 2021;191:1490-8.
60. Andrade MIS, Oliveira JS, Leal VS, et al. Prevalence of insulin resistance and association with metabolic risk factors and food consumption in adolescents - Recife/Brazil. Rev Paul Pediatr 2020;38:e2019016.
61. Ning F, Tuomilehto J, Pyörälä K, Onat A, Söderberg S, Qiao Q. DECODE Study Group. Cardiovascular disease mortality in Europeans in relation to fasting and 2-h plasma glucose levels within a normoglycemic range. Diabetes Care 2010;33:2211-6.
62. Margolis DJ, Jeffcoate W. Epidemiology of foot ulceration and amputation: can global variation be explained? Med Clin North Am 2013;97:791-805.
63. Boudreau DM, Malone DC, Raebel MA, et al. Health care utilization and costs by metabolic syndrome risk factors. Metab Syndr Relat Disord 2009;7:305-14.
64. Nichols GA, Amitay EL, Chatterjee S, Steubl D. Health care costs associated with the development and combination of cardio-renal-metabolic diseases. Kidney360 2023;4:1382-8.
65. Eklof B, Perrin M, Delis KT, et al. Updated terminology of chronic venous disorders: the VEIN-TERM transatlantic interdisciplinary consensus document. J Vasc Surg 2009;49:498-501.
66. Lurie F, Passman M, Meisner M, et al. The 2020 update of the CEAP classification system and reporting standards. J Vasc Surg Venous Lymphat Disord 2020;8:342-52.
67. Salim S, Machin M, Patterson BO, Onida S, Davies AH. Global epidemiology of chronic venous disease: a systematic review with pooled prevalence analysis. Ann Surg 2021;274:971-6.
68. MacColl E, Khalil RA. Matrix metalloproteinases as regulators of vein structure and function: implications in chronic venous disease. J Pharmacol Exp Ther 2015;355:410-28.
69. De Maeseneer MG, Kakkos SK, Aherne T, et al. Editor’s choice - European Society for Vascular Surgery (ESVS) 2022 clinical practice guidelines on the management of chronic venous disease of the lower limbs. Eur J Vasc Endovasc Surg 2022;63:184-267.
70. Recek C. Conception of the venous hemodynamics in the lower extremity. Angiology 2006;57:556-63.
71. Townsley MI. Structure and composition of pulmonary arteries, capillaries, and veins. In: Comprehensive physiology. Wiley; 2011. pp. 675-709.
72. Urbanek T, Skop B, Wiaderkiewicz R, et al. Smooth muscle cell apoptosis in primary varicose veins. Eur J Vasc Endovasc Surg 2004;28:600-11.
74. Ortega MA, Fraile-Martínez O, García-Montero C, et al. Understanding chronic venous disease: a critical overview of its pathophysiology and medical management. J Clin Med 2021;10:3239.
75. Beebe-Dimmer JL, Pfeifer JR, Engle JS, Schottenfeld D. The epidemiology of chronic venous insufficiency and varicose veins. Ann Epidemiol 2005;15:175-84.
76. Jarošíková R, Roztočil K, Husáková J, et al. Chronic venous disease and its intersections with diabetes mellitus. Physiol Res 2023;72:280-6.
77. Gastaldi G, Pannier F, Roztočil K, et al. Chronic venous disease and diabetic microangiopathy: pathophysiology and commonalities. Int Angiol 2021;40:457-69.
78. Chung WS, Lin CL, Kao CH. Diabetes increases the risk of deep-vein thrombosis and pulmonary embolism. A population-based cohort study. Thromb Haemost 2015;114:812-8.
79. Fiebig A, Krusche P, Wolf A, et al. Heritability of chronic venous disease. Hum Genet 2010;127:669-74.
80. Yazıcı D, Sezer H. Insulin resistance, obesity and lipotoxicity. Adv Exp Med Biol 2017;960:277-304.
81. Li X, Yang Y, Shi X, Zhang Z, Ding S. Mitochondria-associated membranes as key regulators in cellular homeostasis and the potential impact of exercise on insulin resistance. Int J Mol Sci 2024;25:3196.
82. Dong Z, Yao X. Insight of the role of mitochondrial calcium homeostasis in hepatic insulin resistance. Mitochondrion 2022;62:128-38.
83. Xu C, Bailly-Maitre B, Reed JC. Endoplasmic reticulum stress: cell life and death decisions. J Clin Invest 2005;115:2656-64.
84. Onyango AN. Cellular stresses and stress responses in the pathogenesis of insulin resistance. Oxid Med Cell Longev 2018;2018:4321714.
85. Rzheshevsky AV. Decrease in ATP biosynthesis and dysfunction of biological membranes. Two possible key mechanisms of phenoptosis. Biochemistry 2014;79:1056-68.
86. Ayer A, Fazakerley DJ, James DE, Stocker R. The role of mitochondrial reactive oxygen species in insulin resistance. Free Radic Biol Med 2022;179:339-62.
87. Smetanina MA, Oscorbin IP, Shadrina AS, et al. Quantitative and structural characteristics of mitochondrial DNA in varicose veins. Vascul Pharmacol 2022;145:107021.
88. Smetanina MA, Kel AE, Sevost’ianova KS, et al. DNA methylation and gene expression profiling reveal MFAP5 as a regulatory driver of extracellular matrix remodeling in varicose vein disease. Epigenomics 2018;10:1103-19.
89. Rötig A, Parfait B, Heidet L, Dujardin G, Rustin P, Munnich A. Sequence and structure of the human OXA1L gene and its upstream elements. Biochim Biophys Acta 1997;1361:6-10.
90. Poerschke S, Oeljeklaus S, Cruz-Zaragoza LD, et al. Identification of TMEM126A as OXA1L-interacting protein reveals cotranslational quality control in mitochondria. Mol Cell 2024;84:345-58.e5.
91. Xu Y, Bei Y, Li Y, Chu H. Phenotypic and functional transformation in smooth muscle cells derived from varicose veins. J Vasc Surg Venous Lymphat Disord 2017;5:723-33.
92. Ugarte-Uribe B, García-Sáez AJ. Apoptotic foci at mitochondria: in and around Bax pores. Philos Trans R Soc Lond B Biol Sci 2017;372:20160217.
93. Korsmeyer SJ, Yin XM, Oltvai ZN, Veis-Novack DJ, Linette GP. Reactive oxygen species and the regulation of cell death by the Bcl-2 gene family. Biochim Biophys Acta 1995;1271:63-6.
94. Gong Y, Luo S, Fan P, Zhu H, Li Y, Huang W. Growth hormone activates PI3K/Akt signaling and inhibits ROS accumulation and apoptosis in granulosa cells of patients with polycystic ovary syndrome. Reprod Biol Endocrinol 2020;18:121.
95. Lim CS, Kiriakidis S, Paleolog EM, Davies AH. Increased activation of the hypoxia-inducible factor pathway in varicose veins. J Vasc Surg 2012;55:1427-39.
96. Vande Velde C, Cizeau J, Dubik D, et al. BNIP3 and genetic control of necrosis-like cell death through the mitochondrial permeability transition pore. Mol Cell Biol 2000;20:5454-68.
97. Shadrina AS, Sharapov SZ, Shashkova TI, Tsepilov YA. Varicose veins of lower extremities: insights from the first large-scale genetic study. PLoS Genet 2019;15:e1008110.
98. Jiang M, Zhang YX, Bu WJ, et al. Piezo1 channel activation stimulates ATP production through enhancing mitochondrial respiration and glycolysis in vascular endothelial cells. Br J Pharmacol 2023;180:1862-77.
99. Zhao C, Sun Q, Tang L, et al. Mechanosensitive ion channel piezo1 regulates diet-induced adipose inflammation and systemic insulin resistance. Front Endocrinol 2019;10:373.
100. Pfluger PT, Kabra DG, Aichler M, et al. Calcineurin links mitochondrial elongation with energy metabolism. Cell Metab 2015;22:838-50.
101. Li M, Gong W, Chen J, Zhang Y, Ma Y, Tu X. PPP3R1 promotes MSCs senescence by inducing plasma membrane depolarization and increasing Ca2+ influx. Int J Mol Sci 2023;24:4421.
102. Zhou Z, Bai J, Zhong S, et al. Integrative genomic analysis of PPP3R1 in Alzheimer’s disease: a potential biomarker for predictive, preventive, and personalized medical approach. EPMA J 2021;12:647-58.
103. Brandman O, Liou J, Park WS, Meyer T. STIM2 is a feedback regulator that stabilizes basal cytosolic and endoplasmic reticulum Ca2+ levels. Cell 2007;131:1327-39.
104. O’Day DH. Calmodulin binding proteins and alzheimer's disease: biomarkers, regulatory enzymes and receptors that are regulated by calmodulin. Int J Mol Sci 2020;21:7344.
105. Glass CK, Olefsky JM. Inflammation and lipid signaling in the etiology of insulin resistance. Cell Metab 2012;15:635-45.
106. Asghar A, Sheikh N. Role of immune cells in obesity induced low grade inflammation and insulin resistance. Cell Immunol 2017;315:18-26.
107. Klimczak S, Śliwińska A. Epigenetic regulation of inflammation in insulin resistance. Semin Cell Dev Biol 2024;154:185-92.
108. Kanda H, Tateya S, Tamori Y, et al. MCP-1 contributes to macrophage infiltration into adipose tissue, insulin resistance, and hepatic steatosis in obesity. J Clin Invest 2006;116:1494-505.
110. Bastard JP, Maachi M, Van Nhieu JT, et al. Adipose tissue IL-6 content correlates with resistance to insulin activation of glucose uptake both in vivo and in vitro. J Clin Endocrinol Metab 2002;87:2084-9.
111. Curat CA, Miranville A, Sengenès C, et al. From blood monocytes to adipose tissue-resident macrophages: induction of diapedesis by human mature adipocytes. Diabetes 2004;53:1285-92.
112. Wang T, He C. Pro-inflammatory cytokines: the link between obesity and osteoarthritis. Cytokine Growth Factor Rev 2018;44:38-50.
113. Rotter V, Nagaev I, Smith U. Interleukin-6 (IL-6) induces insulin resistance in 3T3-L1 adipocytes and is, like IL-8 and tumor necrosis factor-alpha, overexpressed in human fat cells from insulin-resistant subjects. J Biol Chem 2003;278:45777-84.
114. Groeger M, Matsuo K, Heidary Arash E, et al. Modeling and therapeutic targeting of inflammation-induced hepatic insulin resistance using human iPSC-derived hepatocytes and macrophages. Nat Commun 2023;14:3902.
115. Akash MSH, Rehman K, Liaqat A. Tumor necrosis factor-alpha: role in development of insulin resistance and pathogenesis of type 2 diabetes mellitus. J Cell Biochem 2018;119:105-10.
116. Kim TH, Choi SE, Ha ES, et al. IL-6 induction of TLR-4 gene expression via STAT3 has an effect on insulin resistance in human skeletal muscle. Acta Diabetol 2013;50:189-200.
117. Kim JH, Kim JE, Liu HY, Cao W, Chen J. Regulation of interleukin-6-induced hepatic insulin resistance by mammalian target of rapamycin through the STAT3-SOCS3 pathway. J Biol Chem 2008;283:708-15.
118. Reardon CA, Lingaraju A, Schoenfelt KQ, et al. Obesity and insulin resistance promote atherosclerosis through an IFNγ-regulated macrophage protein network. Cell Rep 2018;23:3021-30.
119. Jacobs BN, Andraska EA, Obi AT, Wakefield TW. Pathophysiology of varicose veins. J Vasc Surg Venous Lymphat Disord 2017;5:460-7.
120. Tisato V, Zauli G, Voltan R, et al. Endothelial cells obtained from patients affected by chronic venous disease exhibit a pro-inflammatory phenotype. PLoS One 2012;7:e39543.
121. Abd El-Kader SM, Al-Jiffri OH. Impact of weight reduction on insulin resistance, adhesive molecules and adipokines dysregulation among obese type 2 diabetic patients. Afr Health Sci 2018;18:873-83.
122. Mikuła-Pietrasik J, Uruski P, Aniukiewicz K, et al. Serum from Varicose patients induces senescence-related dysfunction of vascular endothelium generating local and systemic proinflammatory conditions. Oxid Med Cell Longev 2016;2016:2069290.
123. Juhan-Vague I, Alessi MC, Morange PE. Hypofibrinolysis and increased PAI-1 are linked to atherothrombosis via insulin resistance and obesity. Ann Med 2000;32 Suppl 1:78-84.
124. Bui TM, Wiesolek HL, Sumagin R. ICAM-1: a master regulator of cellular responses in inflammation, injury resolution, and tumorigenesis. J Leukoc Biol 2020;108:787-99.
125. Tuleta I, Frangogiannis NG. Diabetic fibrosis. Biochim Biophys Acta Mol Basis Dis 2021;1867:166044.
126. Lattimer CR, Kalodiki E, Geroulakos G, Hoppensteadt D, Fareed J. Are inflammatory biomarkers increased in varicose vein blood? Clin Appl Thromb Hemost 2016;22:656-64.
127. Smetanina MA, Shevela AI, Gavrilov KA, Filipenko ML. The genetic constituent of varicose vein pathogenesis as a key for future treatment option development. Vessel Plus 2021;5:19.
128. Shah R, Hinkle CC, Ferguson JF, et al. Fractalkine is a novel human adipochemokine associated with type 2 diabetes. Diabetes 2011;60:1512-8.
129. Vettor R, Milan G, Rossato M, Federspil G. Review article: adipocytokines and insulin resistance. Aliment Pharmacol Ther 2005;22 Suppl 2:3-10.
130. Singh A, Babyak MA, Nolan DK, et al. Gene by stress genome-wide interaction analysis and path analysis identify EBF1 as a cardiovascular and metabolic risk gene. Eur J Hum Genet 2015;23:854-62.
131. Gustafson B, Smith U. Activation of canonical wingless-type MMTV integration site family (Wnt) signaling in mature adipocytes increases beta-catenin levels and leads to cell dedifferentiation and insulin resistance. J Biol Chem 2010;285:14031-41.
132. Lin HY, Weng SW, Shen FC, et al. Abrogation of toll-like receptor 4 mitigates obesity-induced oxidative stress, proinflammation, and insulin resistance through metabolic reprogramming of mitochondria in adipose tissue. Antioxid Redox Signal 2020;33:66-86.
133. Rajendran P, Rengarajan T, Thangavel J, et al. The vascular endothelium and human diseases. Int J Biol Sci 2013;9:1057-69.
134. Barrett EJ, Eggleston EM, Inyard AC, et al. The vascular actions of insulin control its delivery to muscle and regulate the rate-limiting step in skeletal muscle insulin action. Diabetologia 2009;52:752-64.
135. Bradley EA, Richards SM, Keske MA, Rattigan S. Local NOS inhibition impairs vascular and metabolic actions of insulin in rat hindleg muscle in vivo. Am J Physiol Endocrinol Metab 2013;305:E745-50.
136. Dudzinski DM, Michel T. Life history of eNOS: partners and pathways. Cardiovasc Res 2007;75:247-60.
137. Muniyappa R, Montagnani M, Koh KK, Quon MJ. Cardiovascular actions of insulin. Endocr Rev 2007;28:463-91.
138. Liu Y, Petreaca M, Martins-Green M. Cell and molecular mechanisms of insulin-induced angiogenesis. J Cell Mol Med 2009;13:4492-504.
139. Hermann C, Assmus B, Urbich C, Zeiher AM, Dimmeler S. Insulin-mediated stimulation of protein kinase Akt: a potent survival signaling cascade for endothelial cells. Arterioscler Thromb Vasc Biol 2000;20:402-9.
140. Tiwari S, Sharma N, Gill PS, et al. Impaired sodium excretion and increased blood pressure in mice with targeted deletion of renal epithelial insulin receptor. Proc Natl Acad Sci USA 2008;105:6469-74.
141. Bindlish S, Ng J, Ghusn W, Fitch A, Bays HE. Obesity, thrombosis, venous disease, lymphatic disease, and lipedema: an obesity medicine association (OMA) clinical practice statement (CPS) 2023. Obes Pillars 2023;8:100092.
143. Segiet OA, Brzozowa-Zasada M, Piecuch A, Dudek D, Reichman-Warmusz E, Wojnicz R. Biomolecular mechanisms in varicose veins development. Ann Vasc Surg 2015;29:377-84.
144. Jung UJ, Choi MS. Obesity and its metabolic complications: the role of adipokines and the relationship between obesity, inflammation, insulin resistance, dyslipidemia and nonalcoholic fatty liver disease. Int J Mol Sci 2014;15:6184-223.
145. Costa D, Andreucci M, Ielapi N, et al. Molecular determinants of chronic venous disease: a comprehensive review. Int J Mol Sci 2023;24:1928.
146. Smetanina MA, Korolenya VA, Kel AE, et al. Epigenome-wide changes in the cell layers of the vein wall when exposing the venous endothelium to oscillatory shear stress. Epigenomes 2023;7:8.
147. Laakkonen JP, Lähteenvuo J, Jauhiainen S, Heikura T, Ylä-Herttuala S. Beyond endothelial cells: vascular endothelial growth factors in heart, vascular anomalies and placenta. Vascul Pharmacol 2019;112:91-101.
148. Birdina J, Pilmane M, Ligers A. The morphofunctional changes in the wall of varicose veins. Ann Vasc Surg 2017;42:274-84.
149. Sun X, Zhang H, Liu J, Wang G. Serum vascular endothelial growth factor level is elevated in patients with impaired glucose tolerance and type 2 diabetes mellitus. J Int Med Res 2019;47:5584-92.
150. Kowalewski R, Małkowski A, Sobolewski K, Gacko M. Vascular endothelial growth factor and its receptors in the varicose vein wall. Acta Angiol 2011;17:144-9. Available from: https://journals.viamedica.pl/acta_angiologica/article/view/18677 [Last accessed on 19 Oct 2024]
151. Bruczko-Goralewska M, Romanowicz L, Bączyk J, Wolańska M, Sobolewski K, Kowalewski R. Peptide growth factors and their receptors in the vein wall. J Investig Med 2019;67:1149-54.
152. van der Stoel MM, Kotini MP, Schoon RM, Affolter M, Belting HG, Huveneers S. Vinculin strengthens the endothelial barrier during vascular development. Vasc Biol 2023;5:e220012.
153. Fukaya E, Flores AM, Lindholm D, et al. Clinical and genetic determinants of varicose veins. Circulation 2018;138:2869-80.
154. Shao J, Zeng S, Zhou B, Xu H, Bian Y, Xu Y. Angiogenic factor with G patch and FHA domains 1 (Aggf1) promotes hepatic steatosis in mice. Biochem Biophys Res Commun 2017;482:134-40.
155. D'Oria R, Laviola L, Giorgino F, Unfer V, Bettocchi S, Scioscia M. PKB/Akt and MAPK/ERK phosphorylation is highly induced by inositols: novel potential insights in endothelial dysfunction in preeclampsia. Pregnancy Hypertens 2017;10:107-12.
156. Paternostro G, Pagano D, Gnecchi-Ruscone T, Bonser RS, Camici PG. Insulin resistance in patients with cardiac hypertrophy. Cardiovasc Res 1999;42:246-53.
157. Kim JI, Huh JY, Sohn JH, et al. Lipid-overloaded enlarged adipocytes provoke insulin resistance independent of inflammation. Mol Cell Biol 2015;35:1686-99.
158. Badier-Commander C, Couvelard A, Henin D, Verbeuren T, Michel JB, Jacob MP. Smooth muscle cell modulation and cytokine overproduction in varicose veins. An in situ study. J Pathol 2001;193:398-407.
159. Surendran S, Ramegowda KS, Suresh A, et al. Arterialization and anomalous vein wall remodeling in varicose veins is associated with upregulated FoxC2-Dll4 pathway. Lab Invest 2016;96:399-408.
160. Zhang X, Wang Y, Zuo F, et al. Effect of insulin-regulated FOXC2 expression in adipocyte differentiation and insulin resistance. Diabetes Metab Syndr Obes 2020;13:2801-9.
161. Meissburger B, Stachorski L, Röder E, Rudofsky G, Wolfrum C. Tissue inhibitor of matrix metalloproteinase 1 (TIMP1) controls adipogenesis in obesity in mice and in humans. Diabetologia 2011;54:1468-79.
162. Serralheiro P, Soares A, Costa Almeida CM, Verde I. TGF-β1 in vascular wall pathology: unraveling chronic venous insufficiency pathophysiology. Int J Mol Sci 2017;18:2534.
163. Asthana P, Wong HLX. Preventing obesity, insulin resistance and type 2 diabetes by targeting MT1-MMP. Biochim Biophys Acta Mol Basis Dis 2024;1870:167081.
164. Smetanina MA, Korolenya VA, Sipin FA, et al. Loci cg06256735 and cg15815843 in the MFAP5 gene regulatory regions are hypomethylated in varicose veins apparently due to active demethylation. Biosci Rep 2024;44:BSR20231938.
165. Combs MD, Knutsen RH, Broekelmann TJ, et al. Microfibril-associated glycoprotein 2 (MAGP2) loss of function has pleiotropic effects in vivo. J Biol Chem 2013;288:28869-80.
166. Chen Z, Zhao H, Meng L, Yu S, Liu Z, Xue J. Microfibril-associated glycoprotein-2 promoted fracture healing via integrin αvβ3/PTK2/AKT signaling. Lab Invest 2023;103:100121.
167. Lemaire R, Korn JH, Shipley JM, Lafyatis R. Increased expression of type I collagen induced by microfibril-associated glycoprotein 2: novel mechanistic insights into the molecular basis of dermal fibrosis in scleroderma. Arthritis Rheum 2005;52:1812-23.
168. Vaittinen M, Kolehmainen M, Schwab U, Uusitupa M, Pulkkinen L. Microfibrillar-associated protein 5 is linked with markers of obesity-related extracellular matrix remodeling and inflammation. Nutr Diabetes 2011;1:e15.
169. Özen G, Doğan BSU, Teskin Ö, Norel X, Topal G. The role of perivascular adipose tissue on human saphenous vein vascular tone. J Fac Pharm Istanbul 2016;46:1-13. Available from: https://dergipark.org.tr/tr/download/article-file/266733 [Last accessed on 19 Oct 2024]
Cite This Article
How to Cite
Download Citation
Export Citation File:
Type of Import
Tips on Downloading Citation
Citation Manager File Format
Type of Import
Direct Import: When the Direct Import option is selected (the default state), a dialogue box will give you the option to Save or Open the downloaded citation data. Choosing Open will either launch your citation manager or give you a choice of applications with which to use the metadata. The Save option saves the file locally for later use.
Indirect Import: When the Indirect Import option is selected, the metadata is displayed and may be copied and pasted as needed.
About This Article
Copyright
Data & Comments
Data
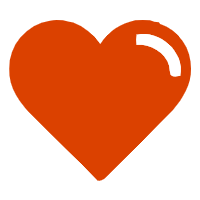
Comments
Comments must be written in English. Spam, offensive content, impersonation, and private information will not be permitted. If any comment is reported and identified as inappropriate content by OAE staff, the comment will be removed without notice. If you have any queries or need any help, please contact us at [email protected].