Cardiac blood vessels and irreversible electroporation: findings from pulsed field ablation
Abstract
The clinical use of irreversible electroporation in invasive cardiac laboratories, termed pulsed field ablation (PFA), is gaining early enthusiasm among electrophysiologists for the management of both atrial and ventricular arrhythmogenic substrates. Though electroporation is regularly employed in other branches of science and medicine, concerns regarding the acute and permanent vascular effects of PFA remain. This comprehensive review aims to summarize the preclinical and adult clinical data published to date on PFA’s effects on pulmonary veins and coronary arteries. These data will be contrasted with the incidences of iatrogenic pulmonary vein stenosis and coronary artery injury secondary to thermal cardiac ablation modalities, namely radiofrequency energy, laser energy, and liquid nitrogen-based cryoablation.
Keywords
INTRODUCTION
Preface
This review article begins by introducing the history of irreversible electroporation before describing its mechanism of action and clinical application within cardiac electrophysiology. A brief summary of the vascular damage associated with thermal cardiac ablation is provided before atrial pulsed field ablation (highlighting preclinical and clinical pulmonary vein findings) and ventricular pulsed field ablation (highlighting preclinical and clinical coronary artery findings) are comprehensively reviewed and contrasted with the aforementioned thermal ablation outcomes.
Historical perspective
The investigation of therapeutic in vivo cardiac electroporation first appeared in the scientific literature in the year 2007[1]. In vitro cardiomyocyte electroporation was described as early as 1987, as the molecular complications of defibrillation and cardioversion were being elucidated[2]. This was many years after cell biology applications for electroporation were already regularly utilized in research laboratories. Clinically, oncology was the first medical discipline to utilize therapeutic electroporation, with literature dating back to the year 1994[3].
Mechanism of action and clinical application
Electroporation is defined as the application of brief supra-physiologic electric field pulses to a cell membrane such that the resulting voltage gradient overwhelms the phospholipid bilayer’s electrical capacitance[4], structurally destabilizing it via intramembrane current flow[5]. Microscopic zones of fluid vaporization lead to the spontaneous development of unstable pores of various sizes. This process can be performed without macroscopic heat generation, assuming a relatively low voltage per centimeter or low pulse duration/frequency, and subsequently minimal resistance from macroscopic current flow[6,7].
The ability to tune the pulse characteristics and field characteristics for irreversible electroporation allows for the targeting of specific tissue types. To this end, a “single shot” myocardium-targeting technique is being increasingly employed that minimizes heat generation and decreases case time, though multiple pulses are likely optimal for sufficient electrical isolation[8]. These pores increase the membrane permeability to a recoverable (reversible) or lethal (irreversible) degree, depending on their quantity, distribution, and diameter[9]. The necessity of a membrane elucidates irreversible electroporation’s ability to spare non-cell-based organic materials such as elastin and collagen, preserving extracellular matrices and subsequent tissue architecture while destroying cellular residents with a susceptible membrane dielectric constant contained within the electric field. Due to their epicardial location, it is thought that autonomic neuron ganglia/terminals may also be preserved with endocardial irreversible electroporation if not contained in the local electric field or oriented properly relative to plane(s) of the electric field[10-12].
The ability to apply sufficiently large electric field pulses using modern endovascular catheter techniques[13,14] facilitated the translation of irreversible electroporation to clinical cardiac electrophysiology for arrhythmogenic substrate management, termed pulsed field ablation (PFA). These electric field pulses can be applied in either a monophasic or biphasic waveform via either a unipolar or bipolar electrode configuration, with the biphasic-bipolar approach having the highest effect-specificity for irreversible plasma membrane pore creation, and allowing for precise lesion areas[13] with a wider margin of error regarding contact force[15] relative to the margin for thermal ablation techniques. The risk of unintentional arrhythmia induction by PFA during a vulnerable phase of the cardiac action potential is mitigated by gating the generator output to the surface electrocardiogram[16,17]. The presence of metal-containing biomaterials from an interventional cardiology case may amplify the pulsed electric field, though only computational data is currently available[18].
Though no clinical guidelines presently exist to govern the application of PFA by electrophysiologists in the United States or in Europe, the consensus of early adopters equates PFA with all prior forms of catheter ablation. Thus, in the European Union where a number of PFA systems have achieved CE certification, PFA may be pursued after failed rhythm control with pharmacologic therapy for patients suffering from atrial fibrillation (AFib)[19]. The advantage of PFA over thermal ablation modalities has become evident when anatomically challenging targets, due to thermal catheter technical limitations or neighboring temperature-sensitive structures, are successfully navigated without an increase in complications or a change in short-term outcomes.
Blood vessel injury from thermal cardiac ablation modalities
The utilization of cardiac catheters to rapidly facilitate local tissue temperature changes (heat for laser/radiofrequency energy and cold for liquid nitrogen-based cryoablation) and disrupt arrhythmogenic substrates carries the risk of unintended damage to the tissue neighboring the lesion [Figure 1]. Unlike PFA, these thermal ablation techniques destroy all cellular and extracellular tissue components via denaturing. Literature exists that proposes a porous media theory to describe and predict thermal ablation of myocardium[20-22]. Due to the compact mediastinal anatomy [Figure 2] and catheter movement due to cardiac activity or respiratory motion, tissues at risk for inadvertent or collateral damage include intracardiac tissues such as the conduction system and coronary arteries, and extracardiac tissues such as the phrenic nerve or esophagus.
Figure 1. Right coronary artery injury after radiofrequency ablation of the cavotricuspid isthmus. (A) Fluoroscopic radiograph (left anterior oblique view) depicts the ablation catheter (red arrow) placed at 6 o’clock position on the cavotricuspid isthmus. During the procedure, the patient developed chest pain with ST- segment elevation in the inferior surface electrocardiogram leads. (B) Emergent coronary angiography revealed near-total occlusion of the distal right coronary artery (white arrow). (C) Follow-up cardiac computed tomography post-revascularization revealed the location of the stenotic segment of the right coronary artery (white arrow) behind the pectinate muscles. This volume-rendered virtual dissection image is viewed from the right posterior oblique view and cranial direction. Multiplanar reconstruction image (C) top-right insertion viewed from the right anterior oblique direction reveals the distance between the right atrial vestibule (black arrow) and the affected right coronary artery (white arrow) is 1.3 millimeters at the pocket beneath the pectinate muscle. (D) Dissection image viewed from the right anterior oblique direction exhibits the proximity of the catheter tip to the distal right coronary artery (white arrow) during cavotricuspid isthmus ablation. L: left coronary aortic sinus; N: non-coronary aortic sinus; R: right coronary aortic sinus; mm: millimeters.
Figure 2. Anatomy of the left and right sinuatrial nodal arteries. (A) High-resolution dissection-enhanced photograph of the left sinuatrial nodal artery (white arrow), which originates from the left circumflex artery and runs along the anterior wall of the left atrium within the Bachmann’s bundle. (B) Multiplanar reconstruction image from the cardiac computed tomography shows the left sinuatrial nodal artery (white arrow) running similar course, suggesting a potential risk of injury of this artery during radiofrequency catheter ablation of the anterior wall of the left atrium. (C) High-resolution dissection-enhanced photograph shows the right sinuatrial nodal artery (white arrow) running between the right atrial appendage and the aortic root. Note that epicardial fat filling this region was thoroughly removed to expose this artery. (D) Multiplanar reconstruction image from the cardiac computed tomography shows the right sinuatrial nodal artery (white arrow) running similar course, suggesting a potential risk of injury of this artery during radiofrequency catheter ablation of the medial wall of the right atrial appendage. L: left coronary aortic sinus; N: non-coronary aortic sinus; R: right coronary aortic sinus.
Thermal ablation mechanisms-of-action are non-selective with regard to tissue/organ toxicity. Furthermore, heterogeneity in lesion depth due to variations in cardiac tissue density or adjacent blood flow-facilitated convective cooling can have a proarrhythmic effect via producing intra-myocardial pathways for aberrant conduction. Thermometric tools that approximate surface temperatures during ablation are employed in the cardiac electrophysiology lab, in addition to chilled/heated saline irrigations to protect off-target damage to adjacent essential structures and contact-force catheters. Nonetheless, thermal complications still occur[23].
Acquired pulmonary vein stenosis secondary to cardiac ablation is defined as the reduction in vein lumen diameter near the location of pulmonary vein ablation [Figure 3]. Grading of this stenosis, accomplished by dedicated cardiac non-invasive imaging, ranges from mild (less than 50% reduction in lumen diameter) to moderate, and severe (greater than 70% reduction in lumen diameter)[24]. Morbidity from this iatrogenic diagnosis can vary from minimal to severe symptom burdens, and though corrective procedures exist, success rates vary and repeat surgeries/percutaneous interventions may be necessary due to the dynamic resolution process[25]. The mechanism of this stenosis is attributed to damage to the intimal layer, causing intimal proliferation and/or fibrosis via myofibroblast activation[26]. The incidence of all grades of pulmonary vein stenosis from catheter-based endocardial ablations is reported to range from 21%[27] to 42%[28]; however, multiple detection biases confound this statistic, particularly the number of and frequency of pulmonary vein imaging studies, given progression to persistent/stable pulmonary vein stenosis from the acute post-ablation stage is variable. Thankfully, the incidence of severe pulmonary vein stenosis with functionally limiting symptoms is negligible with increased operator awareness and modified protocols that minimize intra-vein energy delivery[29].
Figure 3. Pulmonary vein stenosis after balloon cryoablation. Cardiac computed tomography images (slab multiplanar reconstruction) before (A) and 8 months after (B) pulmonary vein isolation using a cryoablation balloon reveals moderate stenosis of the right superior, left superior, and left inferior pulmonary vein ostia. Middle panel images (left lateral view) and right panel images (right anterior oblique and caudal view) are volume-rendered virtual dissection images. LI: Left inferior pulmonary vein; LS: left superior pulmonary vein; RI: right inferior pulmonary vein; RS: right superior pulmonary vein.
Iatrogenic coronary artery vasospasm (time-limited vascular smooth muscle contraction to partial or complete lumen occlusion, usually less than one hour in duration) and post-ablation coronary artery stenosis (permanent restriction of lumen diameter) are markedly less common than acquired pulmonary vein stenosis, with an estimated incidence of < 1%[30]. This estimated incidence is likely an underestimate, given that post-ablation angiography is not routinely performed unless a patient’s symptoms warrant. Furthermore, aggregating cases that involve ablation near the coronary arteries would likely produce a greater incidence of coronary injury. The morbidity of coronary artery stenosis is similar to that of severe persistent pulmonary vein stenosis in that percutaneous and/or open interventions are necessary and may lead to subsequent repeat procedures. Implicated in both endocardial[31,32] and epicardial[33] ablation approaches, coronary artery stenosis can be graded using invasive angiographic techniques (Thrombolysis In Myocardial Infarction scale) or using non-invasive cardiac imaging techniques (example: contrast-enhanced computed tomography coronary angiogram).
ATRIAL PULSED FIELD ABLATION
Preface
It is necessary to acknowledge that multiple atrial ablation procedures implicate coronary arteries, including the right coronary artery during cavotricuspid isthmus ablation for right-sided flutter and the left coronary artery for perimitral ablation for left-sided flutter. Extremely limited data are available to discuss the coronary artery outcomes associated with pulsed field ablation for these particular arrhythmias. Subsequently, no discussion is warranted at this present time. However, we acknowledge the possibility of coronary artery effects during atrial pulsed field ablation, with the goal of increasing awareness for future investigation.
Efficacy and pulmonary vein stenosis
Due to their well-established risk profiles and broad market penetration, thermal cardiac ablation techniques are the tools of choice for invasive cardiologists and cardiac surgeons aiming to modify atrial arrhythmogenic substrate. PFA has slowly gained enthusiasm, though not approved by the Food and Drug Administration at this time, and is being evaluated for its efficacy in decreasing arrhythmogenic burden in supraventricular tachycardia[34], atrial tachycardia[35,36], atrial flutter[37], and atrial fibrillation[38]. In the non-pharmacologic management of atrial fibrillation, the plasticity of atrial substrate may necessitate multiple ablation procedures, including repeat pulmonary vein isolations or lesion extension to deal with post-ablation macroreentrant atrial tachycardia. The risk of pulmonary vein stenosis may correlate positively with the number of total thermal atrial fibrillation ablations[39]; however, parameter-optimized PFA has the theoretical advantage of being markedly toxic to cardiac myocytes, myofibroblasts, and other resident cardiac cell types due to their increased susceptibility. It is important to note the frequently encountered transient ST-segment elevation observed during PFA for pulmonary vein isolation secondary to ion movement disruption. No literature review to date has compiled both the preclinical and clinical outcomes regarding the prevalence of pulmonary vein stenosis with PFA versus the thermal alternatives.
Preclinical pulmonary vein findings with atrial PFA
Though in silico studies on PFA exist[40-42], the majority of preclinical PFA studies have been completed in in vivo animal models, both small[43] and large. Of these in vivo studies, only the large animal studies were designed to recapitulate clinically relevant methodologies regarding atrial tachyarrhythmia catheter ablation techniques in the appropriate anatomic structures. Nine studies were identified, seven conducted in various swine models[44-50] and two conducted in canine models[51,52]. Study designs and sample sizes varied widely [Table 1], and study durations ranged from 3 days[47,52] to 3 months; however, no pulmonary vein stenosis was reported in any of these preclinical studies. Of note, the resolution of pulmonary vein injury secondary to thermal exposure is a slow, time-dependent process, and thus, these acute studies may underestimate the true incidence. Assessment of pulmonary vein diameter/function was predominately assessed via post-mortem histopathology, though serial contrast- enhanced angiogram-based measurements were used as well[44].
Preclinical atrial pulsed field ablation studies that denied pulmonary vein stenosis
Research group [Ref.] | Year | Animal model (weight) | Sample size | Study design | Study duration | Pulsed field ablation | Method of stenosis evaluation |
van Driel et al.[44] | 2014 | Swine (60-75 kg) | 10 | PFA vs. RFA | 3 months | Endocardia l; bipolar; 200 J × 10 | Angiograms; histopathology |
Koruth et al.[45] | 2019 | Female Yorkshire swine (60-70 kg) | 17 | Monophasic PFA vs. biphasic PFA vs. RFA | 10 weeks | Endocardia l; Mono-800 V × 4 beats Bi-1,800 V × 10 beats | Histopathology |
Koruth et al.[46] | 2020 | Female Yorkshire swine (60-70 kg) | 12 | PFA: low dose vs. high dose | 4 weeks 2 weeks | Endocardia l; 21-24 A 24-28 A | ICE |
Ye et al.[47] | 2021 | Male Bama miniswine (80 ± 10 kg) | 3 | Bipolar PFA | 3 days | Endocardia l; 1,600 V/cm @ 8A | Histopathology |
Hsu et al.[48] | 2022 | Swine (no weights provided) | 8 | Safety: supra-therapeutic PFA energy | 1 month | Endocardia l; 1,800 V | ICE; flow velocity |
Zhao et al.[49] | 2022 | Male & female swine (55 kg) | 6 | Biphasic PFA | 1 month | Endocardial; 800-2,000 V | Histopathology |
Koruth et al.[50] | 2023 | Female Yorkshire swine (no weights provided) | 13 | Single-shot PFA: lose dose vs. high dose | 1 week 5 weeks | Endocardial | Histopathology |
Howard et al.[51] | 2020 | Male & female mongrel hound canine (28 kg) | 8 | PFA vs. RFA | 12 weeks | Endocardial; 1,500 V | Cardiac CT |
Koruth et al.[52] | 2023 | Canine (29-36 kg) | 29 | PFA vs. RFA | 0-30 days | Endocardial | Angiography |
Clinical pulmonary vein findings with atrial PFA
Similar to the preclinical reports, no pulmonary vein stenosis was reported in any of the ten clinical publications utilizing PFA for atrial tachyarrhythmia catheter ablation[53-62]. The majority of these studies were completed by the same research group [Table 2], and most of the studies involved pre-ablation pulmonary vein imaging followed by repeat imaging at 3 months post-ablation. This study design is not appropriate to detect acute pulmonary vein reactions, but is meaningful with respect to identifying clinically relevant vascular changes. Though the sample size for each study was small (< 400 patients), the majority of the trials were conducted internationally, increasing the external validity of the findings.
Adult clinical atrial pulsed field ablation studies that denied pulmonary vein stenosis
Research group [Ref.] | Year | Sample size | Study design | Follow up window | Pulsed field ablation | Method of stenosis evaluation |
Reddy et al.[53] | 2018 | 22 | 1st reported PFA use; catheter & surgical | 1 month | Endocardial & epicardial; 900-2,500 V | ICE; voltage mapping |
Reddy et al.[54] | 2019 | 81 | Safety | 120 days | Endocardial; monophasic (900-1,000V) & biphasic (1,800-2,000 V) | Electroanatomic mapping; cardiac CT |
Reddy et al.[55] | 2020 | 25 | PVI + LAPW ablation for persistent AF | 3 months | Endocardial; biphasic, bipolar; 1,600-2.000V | Cardiac CT |
Kuroki et al.[56] | 2020 | 80 | PFA vs. RFA for PV stenosis | 3 months | Endocardial; monophasic (900-1,000 V) & biphasic (1,800-2,000 V) | Cardiac CT |
Reddy et al.[57] | 2021 | 121 | Durability of PVI | 3 months | “Single shot” endocardial; 1,600-1,800 V | Cardiac CT |
Chen et al.[58] | 2022 | 20 | PFA with CIEDs | None | “Single shot” endocardial; biphasic; 1,900-2,000 V | Angiography |
Gunawardene et al.[59] | 2022 | 15 | Repeat AT ablation after prior AF/AT ablation | None | Endocardial; bipolar; 2,000 V | Angiography |
Turagam et al.[60] | 2023 | 21 | Safety and Durability of “Single Shot”; single vs. triple dose | 3 months | Endocardial; 1,700 V | Electroanatomic mapping |
Tohoku et al.[61] | 2023 | 360 | Repeat PVI with 31 mm vs. 35 mm catheter | 6 ± 4 months | Endocardial; 1,800-1,900 V | Angiography |
Duytschaever et al.[62] | 2023 | 226 | Safety and durability of PVI | 3 months | Endocardial; bipolar, biphasic; 1,800V | Cardiac CT |
PFA pulmonary vein stenosis complication rate compared to thermal ablation
Due to the fact that no reports have been published describing pulmonary vein stenosis from PFA, this complication remains specific to thermal ablation techniques. Though non-thermal in mechanism-of-action, PFA is capable of producing heat[6], specifically with high pulse cycle frequencies. Thus, pulmonary vein stenosis remains a theoretical complication of PFA as well. “Single shot” PFA applications reduce this theoretical risk to a negligible level.
VENTRICULAR PULSED FIELD ABLATION
Preface
It is necessary to acknowledge that pulsed field ablation has the possibility of affecting cardiac veins during ventricular ablation procedures. To date, no data exist describing these effects. For this reason, no discussion is warranted at this present time. Nonetheless, we acknowledge the possibility of cardiac vein effects during ventricular pulsed field ablation, with the goal of increasing awareness for future investigation.
Efficacy and coronary artery spasm/stenosis
Applications for PFA include both atrial and ventricular arrhythmogenic substrate management. Though first described[1] and clinically approved in Europe[63] for atrial substrate modification, preclinical ventricular PFA data have suggested more favorable and homogeneous lesion characteristics in both normal and scarred ventricular myocardium[64-67]. However, additional preclinical and clinical evaluation is still necessary to clarify this potential advantage, relative to thermal catheter-based ablation techniques, namely radiofrequency energy. Theories attempting to explain this early data include a relatively high sensitivity of cardiomyocytes to PFA compared to the sensitivities of neighboring cardiac and non-cardiac (i.e., neurons, endothelial cells) cell types[68]. Though the details regarding the safety and durability of PFA continue to be elucidated in time, PFA may one day be a unique catheter-based electrophysiology tool that complements thermal energy in the electrophysiologist’s invasive armamentarium.
One of the safety questions that remains is how PFA affects ventricular cardiac vessels, specifically the epicardial coronary arteries. Irrespective of therapeutic efficacy, damage to the cells of any of the three muscular artery layers is to be avoided; otherwise, PFA would mitigate one arrhythmogenic risk but increase others. Any reactive changes in coronary arteries could lead to intimal hyperplasia and coronary lumen narrowing, smooth muscle hypertrophy and increased propensity to coronary vasospasm, or connective tissue disruptions leading to poor elasticity or compromised target vessels for surgical interventions such as coronary artery bypass grafting. Interestingly, early translational publications clearly documented irreversible electroporation’s ability to disrupt vascular smooth muscle cells and eradicate any cellular residents from arteries without altering extracellular support structures or overall vessel architecture[69-72]. This finding, though seemingly overlooked, could implicate the usage of PFA near arterial vessels, whose smooth muscle is critical to pressure regulation and subsequent flow.
Despite a decade of investigation, there is a lack of consensus regarding best practices to prevent inadvertent coronary injury. Many clinical practices now include the use of parenteral nitroglycerin during PFA in close proximity to coronary arteries, to reduce the risk of and sequelae from vasospasm[73,74]. This illustrates that short- and long-term reactive changes in cardiac vessels in response to PFA will continue to be a point of interest[75]. This portion of the review aims to succinctly summarize the preclinical and clinical ventricular PFA coronary data published to date.
Preclinical coronary artery findings with ventricular PFA
The preclinical safety and efficacy studies evaluating ventricular PFA have been completed in in vivo swine and canine models of normal cardiovascular physiology or induced heart failure with reduced ejection fraction[76-79,80-84] [Table 3]. The swine model remains the gold standard for evaluating clinical cardiac devices, given their similar cardiac dimensions, action potential physiology, and arrhythmogenic propensity compared to humans. Study durations ranged from 48 hours to 3 months. The most common method of coronary artery evaluation was direct visualization using contrast- enhanced fluoroscopy, while the most common method overall was histological analysis of coronary artery architecture. Of note, intimal hyperplasia secondary to coronary artery thermal injury is a slow, time-dependent process, and thus, these acute studies may underestimate the true incidence. Chronic studies to evaluate this phenomenon are needed.
Preclinical ventricular pulsed field ablation studies that denied coronary artery injury
Research group [Ref.] | Year | Animal model (weight) | Sample size | Study design | Study duration | Pulsed field ablation | Method of stenosis evaluation |
du Pré et al.[76] | 2013 | Swine (60-75 kg) | 9 | Lesion vs. non-lesion coronary dimensions | 3 weeks | Epicardial; monophasic; 30-360 J | Histopathology |
Neven et al.[77] | 2014 | Swine (60-75 kg) | 5 | Lesion vs. non-lesion coronary dimensions | 3 months | Epicardial; monophasic; 200 J | Angiography; histopathology |
Neven et al.[78] | 2014 | Swine (60-75 kg) | 5 | PFA dose- response | 3 months | Epicardial; monophasic; 50-200 J | Histopathology |
Neven et al.[79] | 2014 | Swine (60-75 kg) | 5 | PFA dose- response | 3 months | Epicardial; monophasic; 30-300 J | Angiography; histopathology |
Im et al.[80] | 2022 | Female swine (65.5 kg) | 10 | Lesion qualities: PFA vs. RFA | 2 h | Endocardial; biphasic, bipolar; 2,000 V | Histopathology |
Buist et al.[82] | 2023 | Female swine (60-75 kg) | 6 | PFA in coronary sinus | 3 weeks | Endocardial; monophasic; 100 J | Angiography; histopathology |
van Zyl et al.[84] | 2022 | Mongrel canine (30-45 kg) | 8 | PFA across IVS | 30 days | Endocardial; bipolar; 1,000-1,500 V | Angiography; histopathology |
Though the majority of studies were conducted with an epicardial approach by the same operators, the aggregate data[76-79,80,82,84] support the conclusion of ventricular coronary arteries being relatively inert to PFA’s cytotoxicity in the immediate time course, as well as the short-term (days) and medium-term (months) time courses. It is necessary to acknowledge the limitation of these datasets with regard to study timeline duration: no chronic (years-long) studies exist, likely related to the prohibitive costs of housing, feeding, and instrumenting continually growing swine. Studies noted variable intimal hyperplasia in response to PFA without stenosis[76,78] and preserved nerve, artery, and vein architecture via histopathology[80,84].
These findings are contrasted with more recent data from two distinct groups describing pathologic changes secondary to direct PFA[81,83], though some involved methodologies that are not commonly used in clinical practice. The authors’ reports included acute coronary artery spasm secondary to both intra-coronary and epicardial PFA, as well as chronic stenosis via neointimal hyperplasia secondary to intra-coronary artery PFA [Table 4]. These findings are disconcerting in the context of the clinical use of nitroglycerin to prevent coronary artery vasospasm during PFA[74,75], and duplication of these studies to corroborate the initial reports is paramount. A variable that may potentially account for these two divergent results is the location of PFA catheter placement. Though PFA is non-thermal in its cytotoxic mechanism of action, local heat generation is still theoretically possible, particularly with large voltages.
Preclinical Ventricular pulsed field ablation studies that confirmed coronary artery injury
Research group [Ref.] | Year | Animal model (weight) | Sample size | Study design | Study duration | Pulsed field ablation | Method of stenosis evaluation |
Ladejobi et al.[81] | 2022 | Swine (no weights provided) | 14 | IC and epicardial PFA | 1 month | Bipolar or unipolar; 10-143 J | Angiography; histopathology |
Higuchi et al.[83] | 2022 | Female Yorkshire swine (55-65 kg) | 4 | Direct coronary PFA | 8 weeks | Epicardial; 2,000 V | Angiography; histopathology |
Clinical coronary artery findings with ventricular PFA
Clinical data describing ventricular PFA are scarce, with only two case reports identified during an exhaustive search. The 2022 manuscript describes the use of PFA to treat myocardial infarction-related ventricular tachycardia in a patient who had undergone two prior attempts with radiofrequency ablation[84]. The authors report no acute complications from PFA, and the patient had no arrhythmia recurrence at 6 months post-procedure. The more recent case report[85] documents the use of PFA for management of monomorphic VT and reports no evidence of electrocardiogram changes nor coronary artery spasm post-PFA (prophylactic 3 milligrams of intra-coronary nitrate was administered) as evidenced by angiography.
Though multiple clinical trials evaluating the safety and utility of PFA for atrial fibrillation substrate management have been completed, effort has yet to be undertaken to pursue a ventricular tachyarrhythmia equivalent. Additional challenges inherent to ventricular ablation include: ventricular wall thickness, motion from cardiac contraction and subsequent chamber diminution, as well as complex intracavitary structures.
PFA coronary artery stenosis complication rate compared to thermal ablation
Given the limited datasets published on coronary artery injury secondary to PFA, its safety profile relative to thermal ablation remains unclear. Due to the non-thermal mechanism of action, it is reasonable to anticipate that the incidence of coronary artery stenosis will be lower for PFA compared to the thermal alternatives, but additional research is needed to support this hypothesis. Since PFA can damage vascular smooth muscle cells contained within muscular arteries, it must be presumed that both acute and chronic adverse structural changes may occur with this technology.
CONCLUSIONS
Irreversible electroporation is a well-established molecular technique that has more recently been adapted to catheter-based technologies for use in invasive cardiac laboratories. The use of irreversible electroporation to modify cardiac arrhythmia substrates, termed pulsed field ablation (PFA), is increasingly represented in both preclinical and clinical studies, but is still in an early stage and has yet to be approved by the Food and Drug Administration.
Regarding atrial PFA applications, both preclinical and clinical data report no hemodynamically significant stenosis of pulmonary veins. The incidence of pulmonary vein stenosis may be lower for PFA compared to the thermal ablation modalities. This possibility is confounded by the lack of chronic studies available.
Regarding ventricular PFA applications, mixed preclinical data exist regarding whether PFA may be deleterious to coronary arteries. PFA, when applied with clinically appropriate pulse and field characteristics, is capable of ablating vascular cell populations, particularly smooth muscle, without disrupting non-cellular vessel architecture. Nonetheless, prophylactic intra-coronary nitro-based vasodilator administration likely mitigates the hemodynamic sequelae of this smooth muscle effect. Clinical data is purely observational at this present stage of ventricular PFA technology validation, with only one case study identified in the literature.
Future PFA preclinical studies should seek to corroborate prior a priori findings with appropriately powered cohort studies with chronic study durations, and future PFA clinical studies should incorporate high-resolution non-invasive cardiac imaging and functional testing to chronologically describe any coronary perfusion effects.
DECLARATIONS
Acknowledgments
We thank the individuals who have donated their bodies and tissues for the advancement of medical education and research. We thank Koji Fukuzawa, Mitsuru Takami, and Yu Izawa (Kobe University School of Medicine) for their clinical images.
Authors’ contributions
Manuscript drafting: Chinyere IR
Figure drafting, manuscript review: Mori S
Clinical context, manuscript review and revision: Hutchinson MD
Availability of data and materials
Not applicable.
Financial support and sponsorship
Chinyere IR is supported by the National Institutes of Health NIGMS 5-T32GM141830-02.
Conflicts of interest
All authors declare no conflicts of interest.
Ethical approval and consent to participate
Not applicable.
Consent for publication
Not applicable.
Copyright
© The Author(s) 2024.
REFERENCES
1. Lavee J, Onik G, Mikus P, Rubinsky B. A novel nonthermal energy source for surgical epicardial atrial ablation: irreversible electroporation. Heart Surg Forum 2007;10:E162-7.
2. Jones JL, Jones RE, Balasky G. Microlesion formation in myocardial cells by high-intensity electric field stimulation. Am J Physiol 1987;253:H480-6.
3. Dev SB, Hofmann GA. Electrochemotherapy - a novel method of cancer treatment. Cancer Treat Rev 1994;20:105-15.
4. Bakhtiari S, Manshadi MKD, Candas M, Beskok A. Changes in electrical capacitance of cell membrane reflect drug partitioning-induced alterations in lipid bilayer. Micromachines 2023;14:316.
5. Napotnik T, Polajžer T, Miklavčič D. Cell death due to electroporation - a review. Bioelectrochemistry 2021;141:107871.
6. Aycock KN, Campelo SN, Davalos RV. A comparative modeling study of thermal mitigation strategies in irreversible electroporation treatments. J Heat Transfer 2022;144:031206.
7. Dunki-Jacobs EM, Philips P, Martin RC 2nd. Evaluation of thermal injury to liver, pancreas and kidney during irreversible electroporation in an in vivo experimental model. Br J Surg 2014;101:1113-21.
8. Ruwald MH, Johannessen A, Hansen ML, Haugdal M, Worck R, Hansen J. Utility of high-density 3D-bipolar voltage map after pulmonary vein isolation by pulsed-field ablation. JACC Clin Electrophysiol 2023;9:588-90.
9. Lafarge EJ, Muller P, Schroder AP, Zaitseva E, Behrends JC, Marques CM. Activation energy for pore opening in lipid membranes under an electric field. Proc Natl Acad Sci USA 2023;120:e2213112120.
10. Guo F, Wang J, Deng Q, et al. Effects of pulsed field ablation on autonomic nervous system in paroxysmal atrial fibrillation: a pilot study. Heart Rhythm 2023;20:329-38.
11. Musikantow DR, Neuzil P, Petru J, et al. Pulsed field ablation to treat atrial fibrillation: autonomic nervous system effects. JACC Clin Electrophysiol 2023;9:481-93.
12. Stojadinović P, Wichterle D, Peichl P, et al. Autonomic changes are more durable after radiofrequency than pulsed electric field pulmonary vein ablation. JACC Clin Electrophysiol 2022;8:895-904.
13. Howard B, Verma A, Tzou WS, et al. Effects of electrode-tissue proximity on cardiac lesion formation using pulsed field ablation. Circ Arrhythm Electrophysiol 2022;15:e011110.
14. Belalcazar A. Safety and efficacy aspects of pulsed field ablation catheters as a function of electrode proximity to blood and energy delivery method. 2021;2:560-9.
15. Mattison L, Verma A, Tarakji KG, et al. Effect of contact force on pulsed field ablation lesions in porcine cardiac tissue. J Cardiovasc Electrophysiol 2023;34:693-9.
16. Kawamura I, Reddy VY, Santos-Gallego CG, et al. Electrophysiology, pathology, and imaging of pulsed field ablation of scarred and healthy ventricles in swine. Circ Arrhythm Electrophysiol 2023;16:e011369.
17. Verma A, Boersma L, Haines DE, et al. First-in-human experience and acute procedural outcomes using a novel pulsed field ablation system: the PULSED AF pilot trial. Circ Arrhythm Electrophysiol 2022;15:e010168.
18. González-Suárez A, Pérez JJ, O'Brien B, Elahi A. In silico modelling to assess the electrical and thermal disturbance provoked by a metal intracoronary stent during epicardial pulsed electric field ablation. J Cardiovasc Dev Dis 2022;9:458.
19. Reddy VY, Gerstenfeld EP, Natale A, et al. ADVENT Investigators. Pulsed field or conventional thermal ablation for paroxysmal atrial fibrillation. N Engl J Med 2023;389:1660-71.
20. Iasiello M, Andreozzi A, Bianco N, Vafai K. The porous media theory applied to radiofrequency catheter ablation. HFF 2020;30:2669-81.
21. Iasiello M, Andreozzi A, Bianco N, Vafai K. Effects of pulsed radiofrequency source on cardiac ablation. Bioengineering 2023;10:227.
22. Pérez JJ, Nadal E, Berjano E, González-Suárez A. Computer modeling of radiofrequency cardiac ablation including heartbeat-induced electrode displacement. Comput Biol Med 2022;144:105346.
23. Stöckigt F, Schrickel JW, Andrié R, Lickfett L. Atrioesophageal fistula after cryoballoon pulmonary vein isolation. J Cardiovasc Electrophysiol 2012;23:1254-7.
24. Restrepo AJ, Dickfeld TM. 62 - Computed Tomography for Electrophysiology. In: Douglas P. Zipes, José Jalife, William G. Stevenson, Editors. Cardiac electrophysiology: from cell to bedside. Amsterdam: Elsevier; 2018. pp. 587-600.
25. Ahero A, Frauenfelder T, Breitenstein A, Ammann P, Kucher N, Barco S. Partially reversible lung consolidation after revascularization of a total occlusion of both left pulmonary veins following ablation of atrial fibrillation: a case report. Eur Heart J Case Rep 2023;7:ytad057.
26. Vanderlaan RD, Caldarone CA. Pulmonary vein stenosis: incremental knowledge gains to improve outcomes. Children 2021;8:481.
27. Samuel M, Khairy P, Mongeon FP, et al. Pulmonary vein stenosis after atrial fibrillation ablation: insights from the ADVICE trial. Can J Cardiol 2020;36:1965-74.
28. Teunissen C, Velthuis BK, Hassink RJ, et al. Incidence of pulmonary vein stenosis after radiofrequency catheter ablation of atrial fibrillation. JACC Clin Electrophysiol 2017;3:589-98.
29. Kawamura I, Reddy VY, Wang BJ, et al. Pulsed field ablation of the porcine ventricle using a focal lattice-tip catheter. Circ Arrhythm Electrophysiol 2022;15:e011120.
30. Roberts-Thomson KC, Steven D, Seiler J, et al. Coronary artery injury due to catheter ablation in adults: presentations and outcomes. Circulation 2009;120:1465-73.
31. Yue-Chun L, Jia-Feng L, Xue-Qiang G, Peng C. Chronic left coronary artery stenosis after radiofrequency ablation of idiopathic premature ventricular contraction originating from left coronary sinus cusp. Circ Arrhythm Electrophysiol 2016;9:e004353.
32. Caldwell JC, Fath-Odoubadi F, Garratt CJ. Right coronary artery damage during cavotricuspid isthmus ablation. Pacing Clin Electrophysiol 2010;33:e110-3.
33. Stavrakis S, Jackman WM, Nakagawa H, et al. Risk of coronary artery injury with radiofrequency ablation and cryoablation of epicardial posteroseptal accessory pathways within the coronary venous system. Circ Arrhythm Electrophysiol 2014;7:113-9.
34. Li FH, Zeng R, Zhang Q, Ma CS. The efficacy and safety of pulsed-field ablation in para-Hisian paroxysmal supraventricular tachycardia: first-in-human pilot trial. Eur Heart J 2023;44:ehac779.026.
35. Adeliño R, Combes S, El Bouazzaoui R, Albenque JP, Combes N, Boveda S. Pulsed-field ablation of recurrent right atrial tachycardia: expanding the use of electroporation beyond atrial fibrillation. Europace 2023;25:1512.
36. Phlips T, Verhaeghe L, Antole N, Koopman P, Vijgen J. Pulsed field ablation using a focal contact force catheter allowed successful ablation of a focal right atrial tachycardia in the proximity of the phrenic nerve. HeartRhythm Case Rep 2023;9:434-6.
37. Winkelmann S, Lemoine M, Wuerger T, et al. Safety of pulsed-field ablation in patients with cardiac implantable electronic devices. A single-center pilot study. EP Europace 2022;24:euac053.234.
38. Reddy VY, Lehmann JW, Gerstenfeld EP, et al. A randomized controlled trial of pulsed field ablation versus standard-of-care ablation for paroxysmal atrial fibrillation: the ADVENT trial rationale and design. Heart Rhythm O2 2023;4:317-28.
39. Fender EA, Widmer RJ, Hodge DO, et al. Severe pulmonary vein stenosis resulting from ablation for atrial fibrillation: presentation, management, and clinical outcomes. Circulation 2016;134:1812-21.
40. Meckes D, Emami M, Fong I, Lau DH, Sanders P. Pulsed-field ablation: computational modeling of electric fields for lesion depth analysis. Heart Rhythm O2 2022;3:433-40.
41. Gómez-Barea M, García-Sánchez T, Ivorra A. A computational comparison of radiofrequency and pulsed field ablation in terms of lesion morphology in the cardiac chamber. Sci Rep 2022;12:16144.
42. Zang L, Gu K, Ji X, Zhang H, Yan S, Wu X. Comparative analysis of temperature rise between convective heat transfer method and computational fluid dynamics method in an anatomy-based left atrium model during pulsed field ablation: a computational study. J Cardiovasc Dev Dis 2023;10:56.
43. García-Sánchez T, Amorós-Figueras G, Jorge E, et al. Parametric study of pulsed field ablation with biphasic waveforms in an in vivo heart model: the role of frequency. Circ Arrhythm Electrophysiol 2022;15:e010992.
44. van Driel VJ, Neven KG, van Wessel H, et al. Pulmonary vein stenosis after catheter ablation: electroporation versus radiofrequency. Circ Arrhythm Electrophysiol 2014;7:734-8.
45. Koruth J, Kuroki K, Iwasawa J, et al. Preclinical evaluation of pulsed field ablation: electrophysiological and histological assessment of thoracic vein isolation. Circ Arrhythm Electrophysiol 2019;12:e007781.
46. Koruth JS, Kuroki K, Kawamura I, et al. Focal pulsed field ablation for pulmonary vein isolation and linear atrial lesions: a preclinical assessment of safety and durability. Circ Arrhythm Electrophysiol 2020;13:e008716.
47. Ye X, Liu S, Yin H, et al. Study on optimal parameter and target for pulsed-field ablation of atrial fibrillation. Front Cardiovasc Med 2021;8:690092.
48. Hsu JC, Gibson D, Banker R, et al. In vivo porcine characterization of atrial lesion safety and efficacy utilizing a circular pulsed-field ablation catheter including assessment of collateral damage to adjacent tissue in supratherapeutic ablation applications. J Cardiovasc Electrophysiol 2022;33:1480-8.
49. Zhao Z, Chen Y, Wu B, et al. Pulsed-field ablation using a novel ablation-mapping integrated system for pulmonary vein isolation-a preliminary animal study. J Cardiovasc Dev Dis 2022;9:425.
50. Koruth J, Kawamura I, Dukkipati SR, Neuzil P, Reddy VY. Preclinical assessment of the feasibility, safety and lesion durability of a novel 'single-shot' pulsed field ablation catheter for pulmonary vein isolation. Europace 2023;25:1369-78.
51. Howard B, Haines DE, Verma A, et al. Reduction in pulmonary vein stenosis and collateral damage with pulsed field ablation compared with radiofrequency ablation in a canine model. Circ Arrhythm Electrophysiol 2020;13:e008337.
52. Koruth J, Verma A, Kawamura I, et al. PV isolation using a spherical array pfa catheter: preclinical assessment and comparison to radiofrequency ablation. JACC Clin Electrophysiol 2023;9:652-66.
53. Reddy VY, Koruth J, Jais P, et al. Ablation of atrial fibrillation with pulsed electric fields: an ultra-rapid, tissue-selective modality for cardiac ablation. JACC Clin Electrophysiol 2018;4:987-95.
54. Reddy VY, Neuzil P, Koruth JS, et al. Pulsed field ablation for pulmonary vein isolation in atrial fibrillation. J Am Coll Cardiol 2019;74:315-26.
55. Reddy VY, Anic A, Koruth J, et al. Pulsed field ablation in patients with persistent atrial fibrillation. J Am Coll Cardiol 2020;76:1068-80.
56. Kuroki K, Whang W, Eggert C, et al. Ostial dimensional changes after pulmonary vein isolation: Pulsed field ablation vs radiofrequency ablation. Heart Rhythm 2020;17:1528-35.
57. Reddy VY, Dukkipati SR, Neuzil P, et al. Pulsed field ablation of paroxysmal atrial fibrillation: 1-year outcomes of IMPULSE, PEFCAT, and PEFCAT II. JACC Clin Electrophysiol 2021;7:614-27.
58. Chen S, Chun JKR, Bordignon S, et al. Pulsed field ablation-based pulmonary vein isolation in atrial fibrillation patients with cardiac implantable electronic devices: practical approach and device interrogation (PFA in CIEDs). J Interv Card Electrophysiol 2023;66:1929-38.
59. Gunawardene MA, Schaeffer BN, Jularic M, et al. Pulsed field ablation in patients with complex consecutive atrial tachycardia in conjunction with ultra-high density mapping: Proof of concept. J Cardiovasc Electrophysiol 2022;33:2431-43.
60. Turagam MK, Neuzil P, Petru J, et al. PV isolation using a spherical array PFA catheter: application repetition and lesion durability (PULSE-EU Study). JACC Clin Electrophysiol 2023;9:638-48.
61. Tohoku S, Chun KRJ, Bordignon S, et al. Findings from repeat ablation using high-density mapping after pulmonary vein isolation with pulsed field ablation. Europace 2023;25:433-40.
62. Duytschaever M, De Potter T, Grimaldi M, et al. inspIRE Trial Investigators. Paroxysmal atrial fibrillation ablation using a novel variable-loop biphasic pulsed field ablation catheter integrated with a 3-dimensional mapping system: 1-year outcomes of the multicenter inspIRE study. Circ Arrhythm Electrophysiol 2023;16:e011780.
63. Ekanem E, Reddy VY, Schmidt B, et al. MANIFEST-PF Cooperative. Multi-national survey on the methods, efficacy, and safety on the post-approval clinical use of pulsed field ablation (MANIFEST-PF). Europace 2022;24:1256-66.
64. Repp ML, Chinyere IR. Opportunities and challenges in catheter-based irreversible electroporation for ventricular tachycardia. Pathophysiology 2024;31:32-43.
65. Yavin HD, Higuchi K, Sroubek J, Younis A, Zilberman I, Anter E. Pulsed-field ablation in ventricular myocardium using a focal catheter: the impact of application repetition on lesion dimensions. Circ Arrhythm Electrophysiol 2021;14:e010375.
66. Koruth JS, Kuroki K, Iwasawa J, et al. Endocardial ventricular pulsed field ablation: a proof-of-concept preclinical evaluation. Europace 2020;22:434-9.
67. Sandhu U, Alkukhun L, Kheiri B, et al. In vivo pulsed-field ablation in healthy vs. chronically infarcted ventricular myocardium: biophysical and histologic characterization. Europace 2023;25:1503-9.
68. Hunter DW, Kostecki G, Fish JM, Jensen JA, Tandri H. In vitro cell selectivity of reversible and irreversible: electroporation in cardiac tissue. Circ Arrhythm Electrophysiol 2021;14:e008817.
69. Maor E, Ivorra A, Leor J, Rubinsky B. The effect of irreversible electroporation on blood vessels. Technol Cancer Res Treat 2007;6:307-12.
70. Maor E, Ivorra A, Rubinsky B. Non thermal irreversible electroporation: novel technology for vascular smooth muscle cells ablation. PLoS One 2009;4:e4757.
71. Maor E, Ivorra A, Mitchell JJ, Rubinsky B. Vascular smooth muscle cells ablation with endovascular nonthermal irreversible electroporation. J Vasc Interv Radiol 2010;21:1708-15.
72. Phillips M, Maor E, Rubinsky B. Nonthermal irreversible electroporation for tissue decellularization. J Biomech Eng 2010;132:091003.
73. Reddy VY, Petru J, Funasako M, et al. Coronary arterial spasm during pulsed field ablation to treat atrial fibrillation. Circulation 2022;146:1808-19.
74. Gunawardene MA, Schaeffer BN, Jularic M, et al. Coronary spasm during pulsed field ablation of the mitral isthmus line. JACC Clin Electrophysiol 2021;7:1618-20.
75. Wilber DJ. Pulsed-field ablation and the coronary arteries: not so simple. JACC Clin Electrophysiol 2022;8:1497-9.
76. du Pré BC, van Driel VJ, van Wessel H, et al. Minimal coronary artery damage by myocardial electroporation ablation. Europace 2013;15:144-9.
77. Neven K, van Driel V, van Wessel H, et al. Safety and feasibility of closed chest epicardial catheter ablation using electroporation. Circ Arrhythm Electrophysiol ;2014 7:913-9.
78. Neven K, van Driel V, van Wessel H, van Es R, Doevendans PA, Wittkampf F. Myocardial lesion size after epicardial electroporation catheter ablation after subxiphoid puncture. Circ Arrhythm Electrophysiol 2014;7:728-33.
79. Neven K, van Driel V, van Wessel H, van Es R, Doevendans PA, Wittkampf F. Epicardial linear electroporation ablation and lesion size. Heart Rhythm 2014;11:1465-70.
80. Im SI, Higuchi S, Lee A, et al. Pulsed field ablation of left ventricular myocardium in a swine infarct model. JACC Clin Electrophysiol 2022;8:722-31.
81. Ladejobi A, Christopoulos G, Tan N, et al. Effects of Pulsed Electric Fields on the Coronary Arteries in Swine. Circ Arrhythm Electrophysiol 2022;15:e010668.
82. Buist TJ, Groen MHA, Wittkampf FHM, et al. Feasibility of linear irreversible electroporation ablation in the coronary sinus. Cardiovasc Eng Technol 2023;14:60-6.
83. Higuchi S, Im SI, Stillson C, et al. Effect of epicardial pulsed field ablation directly on coronary arteries. JACC Clin Electrophysiol 2022;8:1486-96.
84. van Zyl M, Ladas TP, Tri JA, et al. Bipolar electroporation across the interventricular septum: electrophysiological, imaging, and histopathological characteristics. JACC Clin Electrophysiol 2022;8:1106-18.
Cite This Article
How to Cite
Download Citation
Export Citation File:
Type of Import
Tips on Downloading Citation
Citation Manager File Format
Type of Import
Direct Import: When the Direct Import option is selected (the default state), a dialogue box will give you the option to Save or Open the downloaded citation data. Choosing Open will either launch your citation manager or give you a choice of applications with which to use the metadata. The Save option saves the file locally for later use.
Indirect Import: When the Indirect Import option is selected, the metadata is displayed and may be copied and pasted as needed.
About This Article
Copyright
Data & Comments
Data
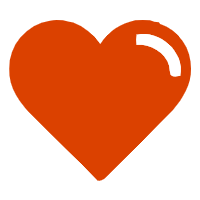
Comments
Comments must be written in English. Spam, offensive content, impersonation, and private information will not be permitted. If any comment is reported and identified as inappropriate content by OAE staff, the comment will be removed without notice. If you have any queries or need any help, please contact us at [email protected].