Recent development in addressing challenges and implementing strategies for manganese dioxide cathodes in aqueous zinc ion batteries
Abstract
Safety issues of energy storage devices in daily life are receiving growing attention, together with resources and environmental concerns. Aqueous zinc ion batteries (AZIBs) have emerged as promising alternatives for extensive energy storage due to their ultra-high capacity, safety, and eco-friendliness. Manganese-based compounds are key to the functioning of AZIBs as the cathode materials thanks to their high operating voltage, substantial charge storage capacity, and eco-friendly characteristics. Despite these advantages, the development of high-performance Mn-based cathodes still faces the critical challenges of structural instability, manganese dissolution, and the relatively low conductivity. Primarily, the charge storage mechanism of manganese-based AZIBs is complex and subject to debate. In view of the above, this review focuses on the mostly investigated MnO2-based cathodes and comprehensively outlines the charge storage mechanisms of MnO2-based AZIBs. Current optimization strategies are systematically summarized and discussed. At last, the perspectives on elucidating advancing MnO2 cathodes are provided from the mechanistic, synthetic, and application-oriented aspects.
Keywords
INTRODUCTION
As the non-renewable energy resources become gradually exhausted with their induced ecological and environmental pollution[1], the goals of carbon neutrality have immediately reached a global consensus. Finding renewable alternative energy sources has been the focus of attention and the key to solving the energy dilemma. Obviously, given the fluctuating nature of most renewable resources for power generation (e.g., wind, tide, solar, etc.), electrochemical energy storage devices have received widespread attention to provide a stable energy supply[2]. Many types of charge carriers, including potassium (K+), sodium (Na+), and lithium (Li+), have been used in developing rechargeable battery technologies to date[3-8]. These developments have been made in systems such as organic electrolytes and aqueous electrolytes, providing a wider range of options for energy storage applications[9-15]. This diversity in charge carriers expands the potential for creating more efficient and tailored energy storage solutions.
Lithium-ion batteries are commonly employed in functioning electronic devices and electric automobiles in the present market[16]. However, their high cost, inadequate safety, and lack of resources are main drawbacks. These have long been recognized as the most common issues that lithium metal anodes encounter and have been considered obstacles to their commercialization[17]. In recent years, multivalent ion [e.g., magnesium (Mg2+), calcium (Ca2+), zinc (Zn2+), etc.] battery systems have emerged as powerful alternatives with their inherited abundance[18-22]. In parallel, aqueous batteries revive with appreciable rechargeability[23], offering affordability, superior security, large theoretical capacity, etc. As the interdisciplinary representative of multi-valence ion and aqueous batteries, aqueous zinc ion batteries (AZIBs) are currently a hotspot in battery research. Zn is a potential anode material due to its high volumetric capacity of 5,855 mAh cm-3 and a substantial specific capacity of 820 mAh g-1[24,25], with a relatively low redox potential of -0.76 V with reference to standard hydrogen electrode [Table 1][24-27]. Aqueous electrolytes are employed with higher safety, environmental friendliness, non-toxicity, and low volatility compared to non-aqueous batteries[28,29]. In addition, aqueous electrolytes possess superior ionic conductivity compared to organic electrolytes, enhancing the battery kinetics, including rate capability and charging/discharging capabilities[30]. A wide class of materials, e.g., Prussian blue analogs (PBAs), vanadium, nickel (Ni)-based, cobalt (Co)-based, and manganese (Mn)-based compounds, can be coupled with Zn anodes to construct AZIBs[31-39] [Figure 1A]. The electrochemical characteristics and reaction processes of the diverse AZIBs are outlined in Table 2 below. Among them, materials based on vanadium have drawn the most interest due to their strong electrochemical activity, which produces quick reaction kinetics, and their large theoretical capacity. Nevertheless, the energy storage capacity of AZIBs is constrained by the relatively modest voltage levels provided by vanadium-based materials[50]. As a typical metal-organic framework (MOF), analogs of Prussian blue can reach output voltages of approximately 1.6 volts, where the open pore structure provides a shorter and direct diffusion path for Zn2+ migration, promoting the rapid diffusion of Zn2+. Ni-based cathodes are known for their stable energy delivery and extended lifespan, but their low electrical conductivity and irreversible phase changes pose significant challenges to their advancement[51]. Conversely, Co-based cathodes exhibit stable cycling ability and high theoretical capacity, but their practical application is limited by their poor electrical conductivity and inefficient capacity utilization[51]. The performance of AZIBs is restricted by the characteristics of cathode materials. The evolved crystallographic structure of cathode materials can lead to the deteriorated cycling stability. Most importantly, the zinc storage mechanism can be altered during the electrochemical process. As a result, investigating innovative cathode materials is essential for AZIB performance[52].
Figure 1. (A) Scheme of an aqueous zinc ion battery; (B) the polymorphs of MnO2; (C) critical issues for the manganese-based cathode materials.
A comparison of various capability parameters for lithium, sodium, potassium, zinc, magnesium, and calcium
Element | Li | Na | K | Zn | Mg | Ca |
Relative atomic mass (g mol-1) | 6.941 | 22.990 | 39.098 | 65.38 | 24.305 | 40.078 |
Ionic radius (Å) | 0.76 | 1.02 | 1.38 | 0.74 | 0.72 | 1.00 |
Redox potential (V) vs. SHE | -3.04 | -2.71 | -2.92 | -0.76 | -2.37 | -2.87 |
Melting point (°C) | 180.5 | 97.8 | 63.5 | 419 | 650 | 842 |
Proportion | 0.0017 | 2.36 | 2.09 | 0.0075 | 2.3 | 3.1 |
A concise overview of the performance characteristics of various cathode materials in AZIBs
Cathode materials | Electrolyte component | Reaction mechanisms | Voltage (V) | Discharge capacity (mAh g-1) | References |
V2O5 | 3 M ZnSO4 | Insertion/desertion mechanism | 0.4-1.4 | 224 (100 mA g-1) | [40] |
VS2 | 1 M ZnSO4 | Insertion/desertion mechanism | 0.4-1.4 | 190 (50 mA g-1) | [41] |
CuHCF | 1 M ZnSO4 | Insertion/desertion mechanism | 0.2-1.2 | 56 (20 mA g-1) | [42] |
ZnHCF | 1 M ZnSO4 | Insertion/desertion mechanism | 0.8-1.9 | 65.4 (60 mA g-1) | [43] |
α-MnO2 | 1 M ZnSO4 | Insertion/desertion mechanism | 1.0-1.8 | 233 (83 mA g-1) | [44] |
β-MnO2 | 1 M ZnSO4 | Insertion/desertion mechanism | 1.0-1.8 | 270 (100 mA g-1) | [45] |
γ-MnO2 | 1 M ZnSO4 | Insertion/desertion mechanism | 1.0-1.8 | 285 (0.05 mA cm-1) | [46] |
δ-MnO2 | 1 M ZnSO4 | Insertion/desertion mechanism | 1.0-2.0 | 269 (100 mA g-1) | [47] |
Ni4Co LDH@Ag1.5NW | 1 M KOH + 0.1 M (CH3COO)2Zn | Insertion/desertion mechanism | 1.4-2.0 | 115.83 (100 mA g-1) | [48] |
ZnCo2O4-x | 2 M polyacrylamide (PAM)/ZnSO4 hydrogel electrolyte | 0.2-1.8 | 148.8 (50 mA g-1) | [49] |
Mn, as an abundant transition metal, has divalent, trivalent, and trivalent states[53]. Among its oxide forms, manganese dioxide (Mn(II)O2) has the most abundant crystallographic isomorphs. The fundamental structural unit of MnO2 is the [MnO6] octahedron. These units are linked together through angular or edge-sharing connections to form various MnO2 structures, such as one-dimensional (1D) tunnel structures (including α-MnO2, β-MnO2 and Todorokite manganite), two-dimensional (2D) layered structures, such as δ-MnO2, and three-dimensional (3D) reticular structures (including the spinel-type μ-MnO2)[54,55] [Figure 1B]. The unique properties of Mn-based materials make them a highly attractive choice for use as cathodes in AZIBs. However, there remain crucial problems that hinder their development: (1) during charging/discharging, Mn2+ undergoes an irreversible disproportionation reaction. The Jahn-Teller distortion of Mn2+ ions can cause the dissolution of manganese cathode materials over repeated charge-discharge cycles. Furthermore, the intercalation and deintercalation of protons can result in the by-product formation of layered double hydroxides on the MnO2 surface, affecting the battery performance[56,57]; (2) Irreversible phase transitions can occur, leading to a significant reduction in the battery capacity over time. Zinc ions, with their relatively large ionic radius (0.76 Å) and potent electrostatic forces, are prone to forming strong bonds with oxygen atoms. This tendency results in substantial internal stress within the material during the cycling of charge and discharge. Consequently, this can trigger a breakdown of the material structure or induce a phase transformation[58,59]; and (3) Mn-based cathode materials typically show relatively poor electrical conductivity, which constrains the reaction kinetics[60]. These problems seriously affect the cell capacity, cycle stability, and Coulombic efficiency of AZIBs. To tackle these challenges, various strategies have been developed, focusing on preventing manganese dissolution, strengthening the structural integrity, and enhancing the electrical conductivity of the materials [Figure 1C].
In light of the significance of Mn-based cathode materials, we have compiled this comprehensive review that concentrates on advancing and applying MnO2-based materials in cutting-edge AZIBs. This review starts by examining charge storage mechanisms of AZIBs that utilize Mn-based cathode materials. More than dissolution-deposition mechanism, we update current understanding on the time-evolving pH effects on charge storage process and point out the necessity of correlating cathode structure and electrolyte design. Consequently, we focus on a more systematic discussion on the current methods for improving MnO2-based cathode materials[61] from the perspectives of nanostructure design, interlayer adjustment, defect engineering, surface modification and electrolyte regulation. Finally, outlook and potential developments of Mn-based AZIBs are provided.
CHARGE STORAGE MECHANISMS FOR AZIBS
The diverse structural properties of cathode materials in AZIBs contribute to a more intricate and varied charge storage mechanism than the conventional rocking chair model observed in typical LIBs. This complexity arises from the interplay of ion insertion/extraction processes and structural transformations within the cathode materials[62]. The differences in reaction mechanisms significantly influence the key performance of the battery. Additionally, research into the reaction mechanism of MnO2-based cathodes is underway. Figure 2A gives a broad overview of the historical development of the reaction mechanism for MnO2-based AZIBs. They evolved from the early alkaline MnO2/Zn batteries. In 1986, Yamamoto pioneered the development of a prototype MnO2/Zn battery by substituting the alkaline electrolyte with a neutral zinc sulfate solution, although the reaction mechanism was unclear at that time [Figure 2B][63]. Until 2011, Xu et al. presented the idea of AZIBs and disclosed the insertion/desertion process of Zn2+ on the MnO2 cathode [Figure 2C][64]. With innovative material synthesis methods and characterization tools,
Figure 2. (A) Overview of the historical development of the reaction mechanism; (B) Zn2+ insertion/desertion mechanism; (C) Co-insertion/desertion mechanism; (D) Conversion reaction mechanism; (E) Dissolution-deposition reaction mechanism.
Insertion/desertion mechanism
The insertion of Zn2+ is the most basic reaction mechanism of MnO2 cathodes. Possessing an ionic radius of 0.74 Å, Zn2+ is easily deserted/inserted during the charging/discharging process, which exists in host materials with layer or tunnel structures. Since the inception of the MnO2/Zn cell, the primary proposed mechanism for energy storage involved the reversible insertion and extraction of Zn2+. Xu et al. contributed to this understanding by demonstrating that Zn2+ ions can indeed reversibly intercalate and deintercalate within the tunnel structure of α-MnO2 when using electrolytes such as ZnSO4 or Zn(NO3)2[64]. α-MnO2 is converted to ZnMn2O4 after Zn2+ insertion. The reaction equations are given as:
The crystal structure of α-MnO2 is characterized by its large and settled tunnels, which are well-suited for accommodating guest cations, including Zn2+, as depicted in Figure 3A. The tunnel structures are indeed pivotal for the reversible intercalation and deintercalation of Zn2+, which are essential processes in the operation of ZIBs. Figure 3B provides a visual representation of the electrochemical activity of α-MnO2 during the battery cycling. The presence of two distinct peaks near 1.3 and 1.7 V indicates the redox reactions occurring at the cathode during charging and discharging. The redox processes involving the insertion/extraction of Zn2+ ions from the α-MnO2 structure are indicated by these peaks. The cycling behavior depicted in Figure 3C, where the material is evaluated in its pristine state, after Zn2+ ions are extracted at a potential of 1.7 V, and after Zn2+ ions are inserted at a potential of 1.3 V, confirms the reversible nature of these processes. Khamsanga and Alfaruqi highlight the advantages of using nanoscale δ-MnO2, which has a shorter ion migration path than α-MnO2. The characteristic of faster insertion and extraction of Zn2+ in δ-MnO2 is indeed advantageous for enhancing the Zn2+ storage capacity. As shown in Figure 3D, the shorter ion migration path in δ-MnO2 leads to a more efficient charge storage process, which is beneficial for the overall performance of ZIBs[67,68]. Note that this mechanism exists not only in MnO2 but also in other cathodes in AZIBs, such as vanadium-based oxides[69], MnO[70], Mn2O3[66], organic compounds[71], and PBAs[42]. In 2012, Alfaruqi et al. showed that the MnOx cathode in AZIB involves only the Zn2+ (de)intercalation reaction through MnOx tunnels[46]. This mechanism implies that Mn disproportionation reactions result in metal ion dissolution, leading to accompanying pH variations during reactions. The observation of pH changes during electrode discharge cycling, ranging from approximately 4.2 to more than 5.2, indicates the presence of a secondary or parasitic process distinct from the primary reaction. This suggests that the electrochemical behavior of MnOx in ARZBs is more complex than initially anticipated.
Figure 3. (A) Working mechanism of AZIB; (B) The graph depicts the anodic and cathodic reactions of the AZIB separately; (C) Zn 2p core level spectra and XRD patterns of cathodic crystalline α-MnO2; This figure is reprinted (adapted) with permission from Xu et al. Copyright (2012) John/Wiley & Sons, Inc[64]. (D) XRD pattern of birnessite δ-MnO2. This figure is reprinted (adapted) with permission from Alfaruqi et al. Copyright (2015) Elsevier Inc[68].
Co-insertion/desertion mechanism
In cases where the cathode material features an open tunneling or layered structure, the electrochemical process can become more intricate than a simple Zn2+ ion insertion. In such instances, the reaction may also involve the participation of H+[72] [Figure 4A]. Aqueous electrolytes in AZIBs contain a higher concentration of H+ than non-aqueous electrolytes[55,73,74]. Using in situ X-ray diffraction (XRD) and ex situ transmission electron microscopy (TEM), Gao et al. confirmed the conjoined insertion and desertion of H+ and Zn2+ in α-MnO2[54]. H+ insertion brings to the MnOOH (Mn4+ to Mn3+). When H+ is inserted at the MnO2 cathode, the environment on the electrode surface becomes OH- rich as the H+ insertion, resulting in OH- reacting with Zn2+ and SO42- and forming zinc hydroxide sulfate ([Zn(OH)2]3ZnSO4∙5H2O) as a by-product in the electrolyte, as shown in the in-situ XRD spectra [Figure 4B]. During intercalation, where water-coordinated Zn2+ and Zn2+ ions are inserted into MnO2, the resulting products are identified as ZnMn3O7∙2H2O[75] and ZnMn2O4[76], as indicated by the XRD patterns in the corresponding charge/discharge states [Figure 4C]. This finding implies that the fluctuations in these peaks show a reversible Zn2+ insertion/desertion mechanism. The reaction equation for the -MnO2/Zn cell is expressed as:
Figure 4. (A) Phase evolution of MnO2; This figure is reprinted (adapted) with permission from Huang et al. Copyright (2019) Oahost[72]. (B) Phase evolution of cathode; (C) Contour graph of in situ XRD data. This figure is reprinted (adapted) with permission from Gao et al. Copyright (2020) Wiley VCH[54].
Furthermore, Bi et al. demonstrated that (Zn(OH)2)3(ZnSO4)(H2O)4 by-product exists on the cathode surface during H+ insertion[77]. After the by-products were dissolved in a mild acid, Zn 2p signals were found using X-ray photoelectron spectroscopy (XPS). The Zn2+ and H+ co-insertion/desertion is confirmed by the aforementioned results.
Conversion reaction mechanism
Other than the insertion/desertion type of charge storage mechanisms, Pan et al. proposed a chemical conversion reaction mechanism characterized by a chemical interaction between α-MnO2 and MnOOH[65]. This mechanism is distinct from the traditional intercalation/deintercalation process and is based on the chemical transformation of the cathode material during discharging[65]. Upon complete discharge, protons originating from the water molecules in the electrolyte can react with α-MnO2, producing MnOOH. During the discharge phase, hydroxide ions (OH-) can engage in the reaction with ZnSO4 and H2O to generate a complex compound, ZnSO4[Zn(OH)2]3·xH2O [Figure 5A], which renders the system electrically neutral. In addition to α-MnO2, β-MnO2 was also reported to exhibit this charge storage mechanism, and its cycling reaction route is shown in Figure 5B[78]. During the initial discharge phase, the transition from Mn3+ to Mn2+ occurs as protons react with β-MnO2 to form MnOOH. The reaction process in the cathode involves the conversion and deposition of MnOOH and Mn2+ as ε-MnO2 in the first charge. In later cycles, the MnOOH can be found, resulting from the reaction of the deposited MnO2 with protons. During discharge, a portion of the MnOOH partially dissolves into Mn2+, generating Zn4SO4(OH)6∙5H2O (ZHS). During the charging process, the Mn2+ present in MnO2 and ZHS is no longer detected. This cathode reaction process can be succinctly given as:
Figure 5. (A) The reaction path of β-MnO2; (B) The XRD patterns of α-MnO2; This figure is reprinted (adapted) with permission from Pan et al. Copyright (2016) Nature Research[65]. (C) In situ pH changes of β-MnO2/Zn. This figure is reprinted (adapted) with permission from Liu et al. Copyright (2021) Elsevier[78].
The pH value shown in Figure 5C increases during discharging and decreases during the charge phase. This supports the involvement of H+ in the electrode reaction process. In the chemical conversion reaction mechanism, new materials are formed, unlike the first two ion-inserted energy storage mechanisms. In this process, hydrogen ions are the only ions inserted/extracted into the cathode material, while Zn2+ is primarily converted to ZHS and does not participate directly in the charging/discharging reactions. The energy density and cycle life of batteries are influenced by the types and amounts of conversion-type cathodes and the category and structure of substrates[79]. Note that the crystal phase and electrolyte differences in manganese oxide necessitate a thorough and precise analysis of the reaction mechanism.
Dissolution-deposition mechanism
The dissolution-deposition process involves the electrical charge transfer between the electrodes, propelled by an electrical potential gradient. This process occurs under both kinetic and thermodynamic constraints, and it allows for the reversible movement of charges between the deposited electrode materials, facilitating the transformation of electrodeposition products back into soluble forms[80]. Liang et al. designed a MnO2/Zn battery based on the deposition-dissolution mechanism, with an electrolyte containing 0.3 mol/L ZnSO4 and 0.3 mol/L MnSO4[81]. The new full battery design operates at a voltage approximately 0.55 V higher than traditional MnO2-based aqueous batteries, attributed to the reaction of the deposition-dissolution mechanism.
In 2020, Guo et al. found that the insertion/desertion of Zn2+ and H+ occurred at the same time as the reaction, as illustrated in Figure 6A[82]. The discharge process of the first cycle with α-MnO2 or δ-MnO2 as the host material involves a series of reactions that produce Mn2+ and OH- ions. These ions then react with the surrounding ZnSO4 in the electrolyte to form ZHS, which consumes a significant amount of H2O. The depletion of H2O in the vicinity of MnO2 suppresses the subsequent dissolution of MnO2, increasing the Mn2+ content in the electrolyte and pH. On the other hand, the newly formed ZHS reacts with Mn2+ during the charge process of the first cycle to produce birnessite-MnO2, which substitutes for the original MnO2 as the host material. This dissolution-deposition mechanism is a key process that dominates the energy storage process and significantly contributes to the specific capacity of the battery. In subsequent cycles, this dissolution-deposition mechanism continues to recur, with birnessite-MnO2 acting as the host material. The intercalation/de-intercalation reaction of Zn2+ ions into and out of the MnO2 structure is considered less critical in the overall energy storage process. In their study, Kankanallu et al. investigated the chemical, morphological, and structural alterations that occur in β-MnO2 due to the dissolution-deposition process[83]. A multimodal approach was employed, integrating operando X-ray diffraction and absorption spectroscopy and X-ray microscopy. Figure 6B and C highlights that the main capacity during the first discharge cycle comes from the dissolution of MnO2 into Mn2+ ions. In parallel, the deposition of ZHS takes place in close proximity to the dissolution site, forming a layered structure on the MnO2 surface. Upon charging, two distinct Zn-Mn complex phases are deposited within the electrode. One is an amorphous phase that can be reversibly formed, while the other is a crystalline phase that is not reversible. Li et al. studied a redox chemical reaction involving MnO2/Mn2+ and interfacial regulation[84]. The study observed that the discharge initially caused the dissolution of MnO2 due to H+ conversion, consequently increasing the pH value and Mn2+ concentration in the electrolyte. Following this, the formation of ZHS occurred, with the process being largely governed by intricate dissolution and deposition reactions. As the charging phase progressed, the concurrent deposition of Zn2+ and Mn2+ created a Zn-rich birnessite phase, ZnxMn3O7, while simultaneously causing the dissolution of ZHS. Subsequently, this was followed by the conversion of ZnxMn3O7 to Mn7O13 through Zn2+ desertion and Mn2+ deposition. In addition, there is a reverse phase transition where the simultaneous insertion of Zn2+ and H+ ions induces the ZnxMn3O7 dissolution, generating ZHS. Continued insertion of H+ ions results in the further dissolution of ZnxMn3O7 until the final formation of ZHS is achieved[84].
Figure 6. (A) Energy storage mechanism in MnO2/Zn batteries. This figure is reprinted (adapted) with permission from Guo et al. Copyright (2020) Elsevier[82]. (B) Mn K-edge ex situ XAS; (C) Operando X-ray diffraction results. This figure is reprinted (adapted) with permission from Kankanallu et al Copyright (2023) RSC Publishing.[83].
The proposal of the deposition-dissolution mechanism has laid the theoretical foundation for the next-generation MnO2/Zn batteries. Unlike the ongoing debate over the dual- or single-electron transfer in conventional mildly acidic ARZBs, the Mn deposition-dissolution involves a dual electron transfer via the Mn2+/Mn4+ redox reaction. Consequently, the theoretical capacity of MnO2/Zn batteries has doubled, reaching a promising value of 616 mAh g-1.
The charge storage mechanism of MnO2-based AZIBs has been a subject of debate since its early development. While various reaction routes have been proposed, several critical factors continue to pose challenges. Understanding the charge storage mechanism of AZIBs is still ongoing, although it is established that the process is not merely a straightforward insertion/desertion mechanism. It is important to note that the exact function of Mn2+ additives in electrochemical processes, especially concerning intercalation and/or conversion reactions, is not yet fully understood. Researchers are still exploring how these additives influence the reaction mechanisms and the overall performance of the electrochemical systems. Further investigation is required to elucidate the specific effects of Mn2+ on the electrochemical behavior. Unlike the study conducted by Lee, which employed in situ pH analysis to investigate the correlation between electrolyte pH and the formation of ZBS [Figure 7A][85], there is limited comprehensive research on the structural and phase changes and the influence of pH during the charge and the discharge cycles of the MnO2 positive electrode in AZIBs[72]. Also, the impact of Mn2+ additives on the fluctuations of pH and the precise function of ZBS remains ambiguous, mainly due to the variations in the concentrations of additives and salts employed in the reported configurations. Excess additives can hinder ZBS formation, which, however, can be significantly influenced by factors such as the changing electrolyte pH (according to the Nernst equation:
Figure 7. (A) Schematic representation of the proposed electrochemical reaction in a typical MnO2/Zn aqueous battery. (B) The development of storage capacity and the mechanisms involved in energy storage during cycles that are influenced by variations in pH levels. This figure is reprinted (adapted) with permission from Yang et al. Copyright (2023) Wiley-VCH[88]. (C) Schematic galvanostatic discharge profiles. This figure is reprinted (adapted) with permission from Sambandam et al. Copyright (2022) Elsevier Inc[89].
The electrochemical performance of MnOx cathodes in AZIBs is influenced by the crystallographic structure of the MnOx polymorphs. Table 3 lists the various forms and crystal structures of MnO2, highlighting the different tunnel structures important for ion insertion and extraction. Among the tunnel-type cathodes, such as α-MnO2, β-MnO2, todorokite-type MnO2, and γ-MnO2, are particularly noteworthy. Additionally,
Type and crystal structure of MnO2
Type | Crystal structure or space group | Lattice constants/Å | Theoretical structures | References |
α-MnO2 | Monoclinic A2/m | a = b = 9.93 c = 2.86 | T2 × 2 | [90] |
β-MnO2 | Rutile structure P42/mnm | a = b = 4.41 c = 2.88 | T1 × 1 | [78] |
β-MnO2 | Pbnm | a = 4.66 b = 2.94 c = 9.34 | T1 × 2 | [53] |
γ-MnO2 | Ortho. | a = 9.61 b = 2.84 c = 4.42 | T1 × 1 & 1 × 2 | [91] |
δ-MnO2 | Birnessite | a = b = 2.83 c = 14.31 | L(m × ∞) | [92] |
λ-MnO2 | Defect spinel structure | a = b = c = 8.09 | N(m × n) | [93] |
ε-MnO2 | Defective NiAs P63/mmc | a = b = 2.83 c = 4.46 | T1 × 1 & 2 × 2 | [53] |
THE STRATEGIES OF PERFORMANCE OPTIMIZATION FOR MnO2-BASED CATHODE
Though being promising, MnO2-based cathodes face critical challenges in AZIBs, among which the three most critical ones are: (a) Manganese dissolution: during the cycling process, the active substance in the electrode dissolves in the electrolyte through disproportionation reaction and produces irreversible side reaction products, which directly leads to the rapid decay of battery capacity; (b) Structural instability: all polymorphs of MnO2 usually undergo structural transformation during repeated intercalation/decalcification of hydrated H+/Zn2+ ions, severe structural disruption, and volumetric changes, which leads to capacity degradation in AZIBs; and (c) Poor electrical conductivity: manganese oxides usually have poor electrical conductivity limiting the rate of electron transfer. Consequently, Mn-based cathode materials of AZIBs present drawbacks in terms of cycling and rate capability. Facing above challenges, several strategies have been employed on MnO2 cathodes, including nanostructure design, interlayer spacing, and defect engineering, surface modification, and electrolyte regulation.
Nanostructure design
The advanced capability of nanostructured electrode materials to improve the storage and discharge of foreign ions is achieved by reducing the diffusion distance for electron and ion transfer, thereby enhancing the diffusion dynamics. Such enhancement is ascribed to (a) the increased surface area-to-volume ratio, which affords more active sites to electrochemical reactions; and (b) the unique structural characteristics in the nanoscale for an electrode. Nanostructure can be classified into four categories: (a) zero-dimensional (0D); (b) 1D; (c) 2D; and (d) hierarchical structure.
Consequently, due to their distinct surface and structural properties, nanomaterials of varying diameters display a range of performances[96]. Nanomaterials (not only MnO2 nanomaterials) with 0D structures, such as those with particle sizes below 100 nm, offer a short pathway for ion diffusion and a broad interface with the electrolyte[97,98]. This enables them to offer distinct advantages; for instance, Wei et al. showed the creation of α-MnO2 with a surface area of 208 m2 g-1 and a substantial initial discharge capacity of
In addition to nanostructured MnO2, nanocomposite was prepared to boost the performance for MnO2 cathodes. Li et al. demonstrated the synthesis of a 3D core-shell structured layered MnO2 on N-doped carbon nanowires for use as a cathode material [Figure 8A and B][102]. Despite the similar shapes and two pairs of distinct redox peaks observed in the cyclic voltammetry (CV) curves of both samples [Figure 8C], the δ-MnO2 nanosheets on N-doped carbon nanowires (MnO2@NC) cathode demonstrated a superior peak current density, which is a sign of enhanced electrochemical storage capacity. This phenomenon was further confirmed by the higher capacity of the MnO2@NC cathode compared to the MnO2 nanosheets grown on bare carbon nanofibers (MnO2@CC) cathode, as shown in Figure 8D. The superior electrochemical performance of the MnO2@NC cathode is attributed to the enhanced conductivity of the N-doped carbon nanowires. MnO2@NC cathode material exhibits a higher capacitance than MnO2@CC cathode material (325 mAh g-1 at 100 mA g-1). Moreover, MnO2@NC demonstrates outstanding rate performance and stable cycling, maintaining around 95% of its original capacity after 2,500 cycles at a current density of 2A/g. Zhang et al. studied the integration of MnO2 nanosheets onto a skeleton of N-doped porous carbon nanosheets to enhance the reactivity and electrical conductivity of the cathode material[103]. Through a comprehensive investigation into the mechanisms responsible for the improved performance of the MnO2 composite electrode in batteries, they found that the N-doped carbon played a pivotal role in lowering the valence state of Mn4+ ions within the MnO2 structure. This reduction in valence state was instrumental in mitigating the electrostatic repulsion between Zn2+/H+ during the embedding process, thus facilitating a higher degree of cation insertion and accelerating ion migration within the electrode.
Interlayer adjustment
The impact of unstable electrode material structure on AZIBs during reaction can be improved by adjusting the interlayer spacing [Figure 9A][101]. Nam et al. explored the effect of structural water content on the capability of layered MnO2 (cw-MnO2). Their research indicated a direct correlation between the water content in cw-MnO2 and the battery capacity [Figure 9B][101]. Specifically, they found that cw-MnO2 with an optimal water molecule content of 0.94 exhibited superior capacity, reaching up to 350 mA h g-1. This finding was further supported by the data presented in Figure 9C-E, which clearly demonstrated the enhanced performance of the cw-MnO2 with the ideal amount of structural water. This finding indicates that suitable water content and interlamellar spacing can help achieve a satisfactory cycle performance by preventing manganese dissolution and preserving the integrity of the electrode structure during cycling. However, the optimal quantity of structural water remains uncertain. Determining how the layer spacing of Mn-based materials and their electrochemical performance relate to the quantity of structural water is crucial. The presence of structural water increases interlayer spacing, shielding electrostatic interactions and thereby facilitating foreign cation diffusion and stabilizing the host structure. However, an elevated content of structural water promotes rapid Zn2+ diffusion, which compromises structural stability and adversely affects the electrochemical performance.
Figure 9. (A) Zn2+-Intercalated structures and relative energies; (B) TGA profiles of pristine. Discharge-charge voltage profiles at various current densities for (C) pristine cw-MnO, (D) cw-MnO2-100, and (E) cw-MnO2-300; This figure is reprinted (adapted) with permission from Nam et al. Copyright (2019) RSC Publishing[101]. (F) Discharge-charge curves for the second cycle (G) differential discharge-charge capacity curves. This figure is reprinted (adapted) with permission from Liu et al. Copyright (2019) Royal Society of Chemistry[104].
Furthermore, as a pillar to support the structure, guest species can be pre-intercalated into the cathode material of a tunnel or layered construction. Liu et al. delved into the effects of pre-intercalated K+ ions on the electrochemical properties of tunnel-structured MnO2-graded nanotubes, denoted as α-K0.19MnO2[104]. For comparison, α-K0.07MnO2 nanotubes were prepared. The investigation demonstrated that the shape and crystal structure of α-K0.07MnO2 nanotubes did not change following the removal of K+. Although the discharging/charging plateaus of both materials were comparable in slope [Figure 9F], the α-K0.19MnO2 cathode showed a lower overpotential. Furthermore, as shown in Figure 9G, it exhibited a higher capacity, reaching 270 mAh g-1. Furthermore, the redox potential of the α-K0.19MnO2 cathode material is lower during insertion/extraction of Zn2+ and H+ ions. This implies that the reversible insertion/extraction of H+ and Zn2+ into the MnO2 is facilitated by the presence of K+ ions.
Besides, nucleating agents can be added in the preparation stage of MnO2 to improve the layer spacing. Hong et al. reduced the energy barrier of MnO2 crystal growth, nucleation and refinement by adding a polyethylene glycol (PEG) nucleating agent in the preparation stage of MnO2, which made PEG-regulated
Defect engineering
Nanostructure defect
MnO2-type crystalline forms are prone to substantial phase transitions and dissolution of Mn during cycling, forming structural collapses that lead to poor long-term cycling performance. Moreover, the advancement of Mn-based cathodes in energy storage is hindered by the challenge of sluggish reaction kinetics, which results from the intense electrostatic interactions between Zn2+ ions and the substrate material. Amorphous manganese dioxide has attracted a lot of attention because of its rich structural flaws and short-range atomic groupings as a solution to this problem. These defects are beneficial in secondary batteries as they can act as reversible ion storage sites, thereby improving ion diffusion kinetics and enhancing the overall capacity of the battery[106-109]. The incorporation of oxygen vacancies within the amorphous MnO2 structure is particularly noteworthy. These vacancies can significantly accelerate charge storage kinetics, allowing for more efficient lithium-ion insertion and deintercalation processes. Moreover, the oxygen-deficient structure of amorphous MnO2 has been shown to contribute to remarkable electrochemical stability, which is crucial for the longevity and reliability of energy storage devices[110]. On the flip side, the inherently isotropic nature of an amorphous structure can play a pivotal role in mitigating the strain that arises from volume changes during the cycling process. This characteristic is particularly beneficial as it can help minimize the degradation of the electrode material over time[111,112]. Cai et al. explored an amorphous manganese dioxide (A-MnO2-δ) electrode material based on abundant structural defects and intrinsic anisotropy, which possesses structural defects that can act as reversible ionic storage sites, enhance ion diffusion kinetics, and increase battery capacity[113]. This amorphous manganese oxide avoids structural collapse during cycling, which enables high-speed zinc ion diffusion kinetics as a cathode for high-speed and stable aqueous rechargeable ZIBs. Its structural flaws may improve battery capacity and ion diffusion kinetics by serving as reversible ion storage sites. Furthermore, the material amorphous structure exhibits an inherently isotropic behavior that may aid in relieving the pressures brought on by volume variations and lessen the electrode material deterioration. A high specific capacity of 301 mAh g-1 at 100 mA g-1 and 78% capacity retention rate after 1,000 cycles are provided by A-MnO2-δ.
Heteroatom doping
Defect introduction can involve using specific methods to create vacancies in the positive electrode material, thereby inducing structural defects. It can also be achieved by doping impurity atoms into the positive electrode material to create heterogeneous atomic defects or by replacing specific elements in Mn-based composite materials to enhance the electrochemical potential of the positive electrode material.
Liang et al. have investigated a novel approach to enhance the electrochemical performance of electrodes by altering their surface characteristics and the associated reaction kinetics[114]. They found that incorporating graphite nanosheets (GNS) with high hydrophobicity and minimal oxygen defects can effectively impede the rate-limiting step of Mn2+ adsorption. This manipulation of the surface properties creates free-standing MnO2 electrodes that exhibit remarkable stability, particularly during high depth of discharge (DOD) cycling and when subjected to multiple charge-discharge cycles. Post-cycling analysis of these electrodes revealed that the occurrence of undesired side reactions was significantly delayed and reduced. This finding challenges the conventional belief that extending the effective cycle life of a cell could be achieved solely by inhibiting the in situ electrodeposition of MnO2. The electrodes developed through this strategy demonstrate a significantly extended cycling life, surpassing 600 cycles, while preserving the high C-rate stability that is characteristic of α-MnO2. The outstanding performance of the electrodes validates the kinetic inhibition strategy proposed by the researchers. This strategy not only improves the cycling stability of MnO2/Zn batteries but also offers a new avenue for developing rechargeable batteries with long cycle lives. Hu et al. improved the electrochemical performance by depositing highly conductive Co9S8 on Ni foams (NF) to form a layered core-shell structure, as shown in Figure 10A, which provided a greater number of electrochemically active sites[115]. This method essentially involves first synthesizing MnO2 on NF and then heat treating it at 400 °C to form bean sprout-like MnO2, as shown in Figure 10B. Next, a simple hydrothermal method was used to uniformly grow MnO2@Co3O4 nanowires on MnO2/NF. Subsequently, an amino exchange reaction was carried out on Co3O4/MnO2/NF to form a MnO2@Co3O4 core-shell structure, and finally, MnO2@Co9S8 was synthesized through a vulcanization reaction. The number of electrochemically reactive active sites and conductivity of the electrode material were increased by coating the MnO2 arrays with highly conductive Co9S8. This enables the MnO2@Co9S8/NF electrode to have a high specific capacitance that is 3.4 and 10.1 times greater than that of the Co3O4@MnO2 and pure MnO2 electrodes, respectively. It achieves an impressive area capacitance of 1,540 mC cm-2 and an energy density of
Figure 10. (A) Schematic representation of preparation process for MnO2@Co9S8/NF; (B) Transmission mapping images of the core-shell structured MnO2@Co9S8/NF. This figure is reprinted (adapted) with permission from Hu et al. Copyright (2021) Pergamon[115].
Surface modification
Surface coatings offer a range of functions and properties that make them essential in various industrial applications. One primary function is to protect against corrosion, wear, oxidation, and pollution. This safeguards the underlying materials and substrates, prolonging their lifespan. In this regard, surface coating provides an effective method for optimizing manganese dioxide cathode materials for AZIBs. It can enhance the stability of manganese dioxide. By forming a protective film on the surface of MnO2, it can prevent unnecessary reactions with the external environment. This protective film can block the entry of oxygen, moisture, and other harmful substances. Also, surface coating methods can effectively inhibit the dissolution of the cathode material and shield the electrostatic interaction between guest ions[91,116].
Carbon materials are frequently used as coatings for MnO2 cathode materials in batteries. The cycle stability of the electrode is improved by this coating process, which is especially efficient in declining the dissolution of manganese ions from the cathode. A straightforward and economical technique has been devised by Islam et al. to generate α-MnO2@C or carbon-coated nanoparticles, which function very well as cathodes in AZIBs[117]. The synthesis process uses maleic acid (C4H4O4) as the carbon source to create a gel, which is then subjected to annealing at a relatively low temperature of 270 °C[117]. TEM analysis confirmed the presence of a uniform carbon network interspersed among the α-MnO2 nanoparticles. This carbon coating not only enhanced the structural integrity of the nanoparticles but also contributed to improved electrochemical performance. The α-MnO2@C composite exhibited a remarkable initial discharge capacity of 272 mAh g-1 at a current density of 66 mA g-1, which is a significant improvement over the pristine α-MnO2, which had a discharge capacity of 213 mAh g-1 under the same conditions. Moreover, the
Figure 11. (A) First cycle and (inset) second cycle. This figure is reprinted (adapted) with permission from Islam et al. Copyright (2017) Elsevier[117]. (B) Cycling performances of MGS; (C) the Ragone plot (based on the weight of cathode material) of the MGS cell; This figure is reprinted (adapted) with permission from Wu et al. Copyright (2018) Wiley-VCH[118]. (D) Cycling stability of MnO2 and PANI@MnO2 electrodes after 3000cycles; (E) Scheme of the synthetic procedure for PANI@MnO2 composite; (F) Schematic illustration of the preparation process of MnO2/rGO/PANI. This figure is reprinted (adapted) with permission from Mao et al. Copyright (2019) Springer[122].
Conductive polymers, for instance, polyaniline (PANI)[119], polypyrrole[120], and polythiophene[121], are excellent candidates for coating materials in battery technology due to their high conductivity and ability to prevent the dissolution of active substances in cathode materials. The application of this coating has the potential to significantly enhance battery performance by increasing the stability of the cathode material[122]. Bao et al. have synthesized PANI@MnO2 composite materials and compared the stability of α-MnO2 and PANI@MnO2 electrodes in a 1 mol/L Na2SO4 aqueous solution, as illustrated in Figure 11D and E[123]. After 3,000 cycles, the α-MnO2 electrode retained 75% of its specific capacitance, while the PANI@MnO2 composite exhibited a higher retention rate of 90%. The enhanced stability of the PANI@MnO2 composite is indeed due to the strong molecular interactions between the amine groups of PANI and the hydroxyl groups of MnO2. These interactions, along with the uniform coating of PANI on the surface of MnO2, are crucial for the electrochemical stability of the composite material. The uniform coating of PANI on MnO2, together with the robust molecular interactions between the amine groups of PANI and the hydroxyl groups of MnO2, contributes to the enhanced stability of the composite material[119]. This coating effectively inhibits the dissolution of MnO2 and the degradation of PANI, leading to superior cycling stability when compared to the individual components.
Surface engineering has proven to be a powerful tool in exploiting advanced composite materials for energy storage applications. By carefully designing the surface composition and structure, it is possible to achieve a synergistic effect that enhances the performance of the material. In the case of Mn-based electrodes, surface engineering can address the issue of manganese dissolution and improve electrical conductivity. Mao et al. have demonstrated this concept with the development of a composite aerogel electrode material (MnO2/rGO/PANI) [Figure 11F][122]. This material is prepared by coating PANI onto manganese dioxide (MnO2) and then combined with reduced graphene oxide (rGO). The resulting composite, when used in a (MnO2/rGO/PANI)/Zn battery, demonstrates a substantial capacity of 241.1 mAh g-1 and maintains 82.7% of its initial capacity after 600 cycles. The MnO2/rGO aerogel has a compact and dense structure, with MnO2 spheres encapsulated by rGO nanosheets and evenly dispersed throughout the graphene network. The N 1s spectrum, with its three distinct peaks at 399.5, 400.4 and 401.1 eV, indicates the presence of amine groups, polarons, and positively charged nitrogen species, respectively. These groups are essential for the conductivity of PANI, as they facilitate electron transfer within the polymer. The presence of these groups in the composite material suggests that the PANI coating significantly enhances the conductivity of the MnO2/rGO aerogel[124-126]. The method offers a practical approach to overcoming the limited conductivity of MnO2. Kamenskii et al. have engineered a composite material by integrating poly(3,4-ethylenedioxythiophene):poly(styrenesulfonate) (PEDOT:PSS) into the MnO2 framework[127]. This composite material, when used to coat MnO2, results in a denser and smoother surface, particularly for the MnO2/PEDOT:PSS electrode, compared to the bare MnO2 electrode. The modification of PEDOT:PSS not only expands the surface area but also boosts the conductivity of the electrode, which makes it easier for the charge to be transported on the electrode surface. This results in a notable improvement in the capacity of the electrode and its ability to maintain its performance over multiple cycles. The MnO2/PEDOT electrode demonstrates a remarkable specific capacity of 238 mAh g-1, with an impressive capacity retention rate of 99%. In contrast, the pristine δ-MnO2 cathode experiences a 10% decrease in capacity, whereas the modified electrodes show no capacity fading after stabilization. The structure stability is enhanced by the composite structure produced by combining organic compounds with manganese dioxide, making it more resistant to structural collapse and reducing adverse electrochemical performance caused by phase change[122]. This has been confirmed by the scanning electron microscopy (SEM) and ex-situ XPS analysis. Simultaneously, it improves the interaction between components. These advantages make such composite materials widely applicable in electrochemical energy storage devices such as ZIBs.
Electrolyte regulation
Electrolyte regulation is one of the most efficient methods to reduce the dissolution of cathode materials during cycling, and it plays an important role in solving the anode reversibility problem[128]. Using a ZnSO4 aqueous solution as the electrolyte in AZIBs is indeed common due to its favorable electrochemical properties[129]. However, it is important to recognize that cells utilizing ZnSO4 electrolytes often require an extended activation period to reach their maximum specific capacity. Furthermore, the cycling stability of AZIBs with ZnSO4 electrolytes can be suboptimal [Figure 12A][130]. Therefore, strategies from the angle of electrolytes are adopted, such as replacing ZnSO4 electrolytes with Zn(CF3SO3)2, synthesizing new zinc electrolytes, and employing additives. However, so far, little has been done on new zinc salts for MnO2 cathodes.
Figure 12. (A) MnO2/Zn battery chemistry. This figure is reprinted (adapted) with permission from Zhang et al. Copyright (2017) Springer Science and Business Media LLC[130]. (B) CV scanning performance of MnO2 electrodes with and without 0.1 M MnSO4 additive in 2 M ZnSO4 aqueous electrolyte. (C) Rate performance (using an electrolyte with a MnSO4 additive). This figure is reprinted (adapted) with permission from Pan et al. Copyright (2016) Nature Research[65].
Adding additives can effectively inhibit Mn2+ dissolution, regulate the ion distribution to obtain a dendrite-free Zn anode, stabilize the electrode/electrolyte interface, and improve the adaptability to extreme environmental conditions[131]. Regulating the electrolyte composition can effectively modulate the redox potential and mitigate side reactions, thereby controlling the formation of undesirable by-products. Treating the dissolution of the cathode material as a reversible process, one can leverage Le Chatelier's principle to optimize the salt concentration of the electrolyte. This strategy aims to increase the concentration of specific cations within the electrolyte, thus declining the dissolution of these cations from the cathode material[132]. It has been particularly effective in the context of Mn-based cathode materials.
Gel electrolytes have been greatly developed for flexible AZIBs[137], which enhances the excellent cycle durability of batteries[138-140]. Further, the incorporation of solid or gel-based electrolytes in battery technology has been demonstrated to mitigate the formation of Zn dendrites[141,142]. Solid or gel electrolytes, characterized by a reduced water content and a strong adsorption affinity, can effectively prevent the manganese dissolution, contributing to achieving long-term cyclability[138].
Based on the above, the mainstream approaches to boosting the performance of MnO2-based cathode materials have been systematically summarized [Table 4]. A primary method to achieve enhancement involves amalgamating MnO2 into nanocomposite materials, which capitalizes on the synergistic effects of the various components to yield outstanding electrochemical performance. Elaboration on the crystal structure of MnO2 itself, such as augmenting defect density and refining the nanostructure, can result in the stabilized crystal structure with enlarged interlayer spacing and the amplified surface area with more active sites. Also, surface modifications can effectuate alterations in the surface properties of MnO2 and further speed up the kinetics of the electrochemical reaction. Further, regulating the solvent in the electrolyte, salt ratio and concentration and incorporating additives can not only enhance electrochemical performance but alter the reaction routes.
Summary of the mainstream approaches to boosting the performance of MnO2-cathode materials
Cathode material | Approaches | Performance (mAh g-1) | References |
α-MnO2 (surface area 208 m2/g) | Nanostructure design | 234 (100 mA g-1) | [47] |
MnO2 (surface area 153 m2/g) | Nanostructure design | 323 (16 mA g-1) | [100] |
cw-MnO2 | Interlayer adjustment | 350 (100 mA g-1) | [101] |
A-MnO2-δ | Nanostructure defect | 301 (100 mA g-1) | [113] |
MnO2@Co9S8 | Heteroatom doping | 963 mF cm-2 (1.5 mA cm-2) | [115] |
α-MnO2@C | Surface modification | 272 (66 mA g-1) | [117] |
MnO2/rGO/PANI | Surface modification | 241 (100 mA g-1) | [122] |
β-MnO2 (aqueous Zn(CF3SO3)2 electrolyte) | Electrolyte Regulation | 275 (0.65 C) | [130] |
OUTLOOKS AND PERSPECTIVES
As next-generation energy storage devices, AZIBs have recently shown a lot of promise because of their low cost, excellent electrochemical performance, low toxicity, ease of production, and outstanding environmental protection. However, there are still important concerns that need to be resolved before AZIBs may be put into practice. The potential of MnO2 cathode materials is being investigated, and research on the charge storage mechanism of MnO2 cathodes is being actively discussed. Meanwhile, large-scale production of MnO2-based nanocomposite electrode materials remains immature. Founded on the above discussion on the charge storage mechanism and optimization strategies for MnO2 cathodes, we here provide an outlook on the future research directions of AZIBs [Figure 13].
(1) It is complex and debatable to the charge storage mechanism of the MnO2 cathode. Advanced in situ technologies, such as electrochemical quartz crystal microbalance (EQCM), are needed to provide a more comprehensive supplement to the microelectrochemical reaction mechanism. More than in situ and in operando characterization, similar diffraction features among the possible Mn-based products during charging/discharging may further disturb the analysis. Hence, the electrolyte system design may play a dispensable role in analyzing the charge storage mechanism of MnO2.
(2) The manganese dissolution-deposition in the process of charge/discharge deserve more intense attention, which is intimately related to the capacity decay of Mn-based electrodes. Importantly, it might become the key to improving the AZIB performance. To comprehensively understand its reaction paths, advanced analytical techniques, such as in situ spectroscopy and microscopy, can be employed to monitor its dissolution and deposition. Computational modeling and simulation can assist in predicting manganese behavior and identifying factors contributing to capacity decay. By integrating experimental and theoretical approaches, the fine details of dissolution-deposition mechanism can be explored, paving way on designing new-type Mn-based rechargeable batteries.
(3) Although numerous optimization strategies have been developed to modify the MnO2 cathode material, a single strategy cannot address multiple problems simultaneously. Surface coating to form nanocomposite structure may offer the most efficient solution by integrating multi-functions to depress manganese dissolution and improve the conductivity. On the other hand, optimization strategies need to be developed with the associated charge storage mechanism. Considering this, electrolytes, which provide large room to improve the battery performance, indeed call for the scrutinization on their related charge storage mechanism, especially for emerging water-in-salt electrolytes, deep eutectic solvents, and quasi-/solid state electrolytes. Moreover, few studies remain on the effects of fluid collectors and adhesives, which are crucial for enhancing the overall performance of AZIBs[116,143-145].
(4) The subject of flexible energy storage is continuing to expand as wearable flexible electronics, health monitors, and flexible display devices gradually evolve. High-toughness, low-cost expandable ZIBs have been produced quickly. The mechanical strength, water content of gel, and the tightness of the electrolyte-to-electrode contact interface, however, may cause the battery to distort and raise its risk factor during this process. Therefore, solving these problems has become the key to developing AZIBs.
(5) Exploring advanced cathode materials and understanding charge storage mechanisms are crucial for advancing zinc battery technology. Nevertheless, lacking comprehensive engineering insights can impede the practical application of scholarly breakthroughs within industrial settings. Consequently, it is imperative to focus on assessing novel research outcomes against critical benchmarks. This approach will expedite the shift from theoretical research to the widespread implementation of energy storage systems within real-world power networks and manufacturing processes. The critical benchmarks encompass energy storage capacity, safety, cost-effectiveness, the anode-to-cathode capacity ratio, etc.
Despite the significant achievements for the long-being explored MnO2 cathode materials, critical issues persist, necessitating further research to realize their application for AZIBs. In view of this, we comprehensively summarize the charge storage mechanisms for these materials in AZIBs, with current concern on the pH-dependent process and time-evolving trend. The main challenges and equivalent optimization strategies to MnO2 cathodes are then presented and analyzed thoroughly. With the above discussion, outlooks are provided from the perspectives of charge storage mechanism, advanced material/electrode design, and interdisciplinary functional design. We envision this review provide an insightful guide on the future development of advancing Mn-based AZIBs.
DECLARATIONS
Authors’ contributions
Substantial contributions to conception and design of the study, and project administration: Dai C, Yao M, Yuan D
Assistance in the design of the study, manuscript editing, and administrative support: Wang Q, Cai W, Zhang Y
Manuscript drafting and editing and technical support: Luo C, Lei H, Xiao Y, Nie X, Li Y
Availability of data and materials
Not applicable.
Financial support and sponsorship
This work was financially supported by the National Key Research and Development Program (2021YFB2400202), the Fundamental Research Funds for the Central Universities (No. YJ202280), the 100 Talented Team of Hunan Province (XiangZu [2016] 91), and the Natural Science Foundation of Hunan Province (2022JJ30613).
Conflicts of interest
All authors declared that there are no conflicts of interest.
Ethical approval and consent to participate
Not applicable.
Consent for publication
Not applicable.
Copyright
© The Author(s) 2024.
REFERENCES
1. Jing J, Liu M, Colvin VL, Li W, Yu WW. Photocatalytic degradation of nitrogen-containing organic compounds over TiO2. J Mol Catal A Chem 2011;351:17-28.
2. Guan P, Zhou L, Yu Z, et al. Recent progress of surface coating on cathode materials for high-performance lithium-ion batteries. J Energy Chem 2020;43:220-35.
3. Ponrouch A, Frontera C, Bardé F, Palacín MR. Towards a calcium-based rechargeable battery. Nat Mater 2016;15:169-72.
4. Nam KW, Kim S, Lee S, et al. The high performance of crystal water containing manganese birnessite cathodes for magnesium batteries. Nano Lett 2015;15:4071-9.
5. Saha P, Datta MK, Velikokhatnyi OI, Manivannan A, Alman D, Kumta PN. Rechargeable magnesium battery: current status and key challenges for the future. Prog Mater Sci 2014;66:1-86.
6. Muldoon J, Bucur CB, Oliver AG, et al. Electrolyte roadblocks to a magnesium rechargeable battery. Energy Environ Sci 2012;5:5941.
7. Pramudita JC, Sehrawat D, Goonetilleke D, Sharma N. An initial review of the status of electrode materials for potassium-ion batteries. Adv Energy Mater 2017;7:1602911.
8. Han J, Niu Y, Bao SJ, Yu YN, Lu SY, Xu M. Nanocubic KTi2(PO4)3 electrodes for potassium-ion batteries. Chem Commun 2016;52:11661-4.
10. Hueso KB, Armand M, Rojo T. High temperature sodium batteries: status, challenges and future trends. Energy Environ Sci 2013;6:734.
11. Luo C, Xu Y, Zhu Y, et al. Selenium@mesoporous carbon composite with superior lithium and sodium storage capacity. ACS Nano 2013;7:8003-10.
12. Yang X, Xu J, Chang Z, et al. Blood-capillary-inspired, free-standing, flexible, and low-cost super-hydrophobic N-CNTs@SS cathodes for high-capacity, high-rate, and stable Li-air batteries. Adv Energy Mater 2018;8:1702242.
13. Abouimrane A, Dambournet D, Chapman KW, Chupas PJ, Weng W, Amine K. A new class of lithium and sodium rechargeable batteries based on selenium and selenium-sulfur as a positive electrode. J Am Chem Soc 2012;134:4505-8.
14. Liu J, Xu C, Chen Z, Ni S, Shen ZX. Progress in aqueous rechargeable batteries. Green Energy Environ 2018;3:20-41.
15. Wang Q, Sarkar A, Wang D, et al. Multi-anionic and -cationic compounds: new high entropy materials for advanced Li-ion batteries. Energy Environ Sci 2019;12:2433-42.
16. Wang Q, Yao M, Zhu A, Wang Q, Wu H, Zhang Y. Semi-metallic superionic layers suppressing voltage fading of Li-rich layered oxide towards superior-stable Li-ion batteries. Angew Chem Int Ed 2023;62:e202309049.
17. Kang S, Cheng J, Gao W, Cui L. Toward safer lithium metal batteries: a review. Energy Mater 2023;3:300043.
18. Pan Z, Liu X, Yang J, et al. Aqueous rechargeable multivalent metal-ion batteries: advances and challenges. Adv Energy Mater 2021;11:2100608.
19. Qiu S, Xu Y, Wu X, Ji X. Prussian blue analogues as electrodes for aqueous monovalent ion batteries. Electrochem Energy Rev 2022;5:242-62.
20. Dong N, Zhang F, Pan H. Towards the practical application of Zn metal anodes for mild aqueous rechargeable Zn batteries. Chem Sci 2022;13:8243-52.
21. Wan F, Zhang Y, Zhang L, et al. Reversible Oxygen redox chemistry in aqueous zinc-ion batteries. Angew Chem Int Ed 2019;58:7062-7.
22. Zhao K, Wang C, Yu Y, et al. Ultrathin surface coating enables stabilized zinc metal anode. Adv Mater Inter 2018;5:1800848.
23. Yang F, Yuwono JA, Hao J, et al. Understanding H2 evolution electrochemistry to minimize solvated water impact on zinc-anode performance. Adv Mater 2022;34:e2206754.
24. Zhao G, Rui K, Dou SX, Sun W. Heterostructures for electrochemical hydrogen evolution reaction: a review. Adv Funct Mater 2018;28:1803291.
25. Wang F, Borodin O, Gao T, et al. Highly reversible zinc metal anode for aqueous batteries. Nat Mater 2018;17:543-9.
26. Kim JY, Liu G, Shim GY, Kim H, Lee JK. Functionalized Zn@ZnO hexagonal pyramid array for dendrite-free and ultrastable zinc metal anodes. Adv Funct Mater 2020;30:2004210.
27. He H, Tong H, Song X, Song X, Liu J. Highly stable Zn metal anodes enabled by atomic layer deposited Al2O3 coating for aqueous zinc-ion batteries. J Mater Chem A 2020;8:7836-46.
28. Tarascon JM, Armand M. Issues and challenges facing rechargeable lithium batteries. Nature 2001;414:359-67.
29. Olivetti EA, Ceder G, Gaustad GG, Fu X. Lithium-ion battery supply chain considerations: analysis of potential bottlenecks in critical metals. Joule 2017;1:229-43.
30. Wang L, Wang P, Wang T, Yin Y, Guo Y, Wang C. Prussian blue nanocubes as cathode materials for aqueous Na-Zn hybrid batteries. J Power Sources 2017;355:18-22.
31. Liu X, Ma L, Du Y, Lu Q, Yang A, Wang X. Vanadium pentoxide nanofibers/carbon nanotubes hybrid film for high-performance aqueous zinc-ion batteries. Nanomaterials 2021;11:1054.
32. Li Y, Yu D, Lin S, Sun D, Lei Z. Preparation of α-MnO2 nanorods/porous carbon cathode for aqueous zinc-ion batteries. Acta Chimica Sinica 2021;79:200.
33. Li Z, Liu T, Meng R, et al. Insights into the structure stability of prussian blue for aqueous zinc ion batteries. Energy Environ Mater 2021;4:111-6.
34. Liu Q, Ma Z, Chen Z, et al. A polyaniline surface-modified Prussian blue analogue cathode for flexible aqueous Zn-ion batteries. Chem Commun 2022;58:8226-9.
35. Sun W, Wang F, Hou S, et al. Zn/MnO2 battery chemistry with H+ and Zn2+ coinsertion. J Am Chem Soc 2017;139:9775-8.
36. Wu P, Kong X, Feng Y, et al. Phase engineering on amorphous/crystalline γ-Fe2O3 nanosheets for boosting dielectric loss and high-performance microwave absorption. Adv Funct Mater 2024;34:2311983.
37. Ding J, Du Z, Li B, et al. Unlocking the potential of disordered rocksalts for aqueous zinc-ion batteries. Adv Mater 2019;31:e1904369.
38. Caldeira V, Rouget R, Fourgeot F, et al. Controlling the shape change and dendritic growth in Zn negative electrodes for application in Zn/Ni batteries. J Power Sources 2017;350:109-16.
39. Wang X, Wang F, Wang L, et al. An aqueous rechargeable Zn//Co3O4 Battery with high energy density and good cycling behavior. Adv Mater 2016;28:4904-11.
40. Zhou J, Shan L, Wu Z, Guo X, Fang G, Liang S. Investigation of V2O5 as a low-cost rechargeable aqueous zinc ion battery cathode. Chem Commun 2018;54:4457-60.
41. He P, Yan M, Zhang G, et al. Layered VS2 nanosheet-based aqueous Zn ion battery cathode. Adv Energy Mater 2017;7:1601920.
42. Jia Z, Wang B, Wang Y. Copper hexacyanoferrate with a well-defined open framework as a positive electrode for aqueous zinc ion batteries. Mater Chem Phys 2015;149-50:601-6.
43. Zhang L, Chen L, Zhou X, Liu Z. Towards high-voltage aqueous metal-ion batteries beyond 1.5 V: the zinc/zinc hexacyanoferrate system. Adv Energy Mater 2015;5:1400930.
44. Alfaruqi MH, Gim J, Kim S, et al. Enhanced reversible divalent zinc storage in a structurally stable α-MnO2 nanorod electrode. J Power Sources 2015;288:320-7.
45. Islam S, Alfaruqi MH, Mathew V, et al. Facile synthesis and the exploration of the zinc storage mechanism of β-MnO2 nanorods with exposed (101) planes as a novel cathode material for high performance eco-friendly zinc-ion batteries. J Mater Chem A 2017;5:23299-309.
46. Alfaruqi MH, Mathew V, Gim J, et al. Electrochemically induced structural transformation in a γ-MnO2 cathode of a high capacity zinc-ion battery system. Chem Mater 2015;27:3609-20.
47. Wei C, Xu C, Li B, Du H, Kang F. Preparation and characterization of manganese dioxides with nano-sized tunnel structures for zinc ion storage. J Phys Chem Solids 2012;73:1487-91.
48. Xuan X, Qian M, Pan L, et al. A hollow tubular NiCo layacknered double hydroxide@Ag nanowire structure for high-power-density flexible aqueous Ni//Zn battery. J Energy Chem 2022;70:593-603.
49. Huang J, Li Y, Xie R, et al. Structural engineering of cathodes for improved Zn-ion batteries. J Energy Chem 2021;58:147-55.
50. Cui Y, Ding Y, Guo L, et al. Ultra-long Zn3V2O7(OH)2·2H2O nanowires grown on carbon cloth as cathode material for aqueous zinc-ion batteries. Energy Mater 2023;3:300023.
51. Zhu Y, Guan P, Zhu R, et al. Recent advances in flexible alkaline zinc-based batteries: materials, structures, and perspectives. J Energy Chem 2023;87:61-88.
52. Lu C, Yang Z, Wang Y, et al. Ethylene glycol-regulated ammonium vanadate with stable layered structure and favorable interplanar spacing as high-performance cathode for aqueous zinc ion batteries. Chin Chem Lett 2023;34:108572.
53. Wei W, Cui X, Chen W, Ivey DG. Manganese oxide-based materials as electrochemical supercapacitor electrodes. Chem Soc Rev 2011;40:1697-721.
54. Gao X, Wu H, Li W, et al. H+-insertion boosted α-MnO2 for an aqueous Zn-ion battery. Small 2020;16:e1905842.
55. Wan F, Zhang L, Dai X, Wang X, Niu Z, Chen J. Aqueous rechargeable zinc/sodium vanadate batteries with enhanced performance from simultaneous insertion of dual carriers. Nat Commun 2018;9:1656.
56. Álvarez-serrano I, Almodóvar P, Giraldo DA, Llopis F, Solsona B, López ML. Stable manganese-oxide composites as cathodes for Zn-ion batteries: interface activation from in situ layer electrochemical deposition under 2 V. Adv Mater Inter 2022;9:2101924.
57. Shen Z, Tang Z, Li C, et al. Precise proton redistribution for two-electron redox in aqueous zinc/manganese dioxide batteries. Adv Energy Mater 2021;11:2102055.
58. Jing F, Liu Y, Shang Y, et al. Dual ions intercalation drives high-performance aqueous Zn-ion storage on birnessite-type manganese oxides cathode. Energy Stor Mater 2022;49:164-71.
59. Zhao Y, Zhang P, Liang J, et al. Unlocking layered double hydroxide as a high-performance cathode material for aqueous zinc-ion batteries. Adv Mater 2022;34:e2204320.
60. Guo D, Zhao W, Pan F, Liu G. Block copolymer-derived porous carbon fibers enable high MnO2 loading and fast charging in aqueous zinc-ion battery. Batteries Supercaps 2022;5:e202100380.
61. Dai H, Zhou R, Zhang Z, Zhou J, Sun G. Design of manganese dioxide for supercapacitors and zinc-ion batteries: similarities and differences. Energy Mater 2022;2:200040.
62. Chen H, Dai C, Xiao F, et al. Reunderstanding the reaction mechanism of aqueous Zn-Mn batteries with sulfate electrolytes: role of the zinc sulfate hydroxide. Adv Mater 2022;34:e2109092.
63. Shoji T, Hishinuma M, Yamamoto T. Zinc-manganese dioxide galvanic cell using zinc sulphate as electrolyte. Rechargeability of the cell. J Appl Electrochem 1988;18:521-6.
64. Xu C, Li B, Du H, Kang F. Energetic zinc ion chemistry: the rechargeable zinc ion battery. Angew Chem Int Ed 2012;51:933-5.
65. Pan H, Shao Y, Yan P, et al. Reversible aqueous zinc/manganese oxide energy storage from conversion reactions. Nat Energy 2016;1:16039.
66. Zhu C, Fang G, Liang S, et al. Electrochemically induced cationic defect in MnO intercalation cathode for aqueous zinc-ion battery. Energy Stor Mater 2020;24:394-401.
67. Khamsanga S, Pornprasertsuk R, Yonezawa T, Mohamad AA, Kheawhom S. δ-MnO2 nanoflower/graphite cathode for rechargeable aqueous zinc ion batteries. Sci Rep 2019;9:8441.
68. Alfaruqi MH, Gim J, Kim S, et al. A layered δ-MnO2 nanoflake cathode with high zinc-storage capacities for eco-friendly battery applications. Electrochem Commun 2015;60:121-5.
69. Mathew V, Sambandam B, Kim S, et al. Manganese and vanadium oxide cathodes for aqueous rechargeable zinc-ion batteries: a focused view on performance, mechanism, and developments. ACS Energy Lett 2020;5:2376-400.
70. Fu Y, Wei Q, Zhang G, et al. High-performance reversible aqueous Zn-ion battery based on porous MnOx nanorods coated by MOF-derived N-doped carbon. Adv Energy Mater 2018;8:1801445.
71. Wang Q, Liu Y, Chen P. Phenazine-based organic cathode for aqueous zinc secondary batteries. J Power Sources 2020;468:228401.
72. Huang Y, Mou J, Liu W, et al. Novel insights into energy storage mechanism of aqueous rechargeable Zn/MnO2 batteries with participation of Mn2. Nanomicro Lett 2019;11:49.
73. Zhao Q, Chen X, Wang Z, et al. Unravelling H+/Zn2+ synergistic intercalation in a novel phase of manganese oxide for high-performance aqueous rechargeable battery. Small 2019;15:e1904545.
74. Wang L, Zheng J. Recent advances in cathode materials of rechargeable aqueous zinc-ion batteries. Mater Today Adv 2020;7:100078.
75. Qiu N, Chen H, Yang Z, Sun S, Wang Y. Synthesis of manganese-based complex as cathode material for aqueous rechargeable batteries. RSC Adv 2018;8:15703-8.
76. Siamionau U, Aniskevich Y, Mazanik A, et al. Rechargeable zinc-ion batteries with manganese dioxide cathode: How critical is choice of manganese dioxide polymorphs in aqueous solutions? J Power Sources 2022;523:231023.
77. Bi S, Wu Y, Cao A, Tian J, Zhang S, Niu Z. Free-standing three-dimensional carbon nanotubes/amorphous MnO2 cathodes for aqueous zinc-ion batteries with superior rate performance. Mater Today Energy 2020;18:100548.
78. Liu W, Zhang X, Huang Y, et al. β-MnO2 with proton conversion mechanism in rechargeable zinc ion battery. J Energy Chem 2021;56:365-73.
79. Kang J, Zhao Z, Li H, Meng Y, Hu B, Lu H. An overview of aqueous zinc-ion batteries based on conversion-type cathodes. Energy Mater 2022;2:200009.
80. Xu Y, Huang W, Liu J, et al. Promoting the reversibility of electrolytic MnO2-Zn battery with high areal capacity by VOSO4 mediator. Energy Mater 2024;4:400005.
81. Liang G, Mo F, Li H, et al. A universal principle to design reversible aqueous batteries based on deposition-dissolution mechanism. Adv Energy Mater 2019;9:1901838.
82. Guo X, Zhou J, Bai C, Li X, Fang G, Liang S. Zn/MnO2 battery chemistry with dissolution-deposition mechanism. Mater Today Energy 2020;16:100396.
83. Kankanallu VR, Zheng X, Leschev D, et al. Elucidating a dissolution-deposition reaction mechanism by multimodal synchrotron X-ray characterization in aqueous Zn/MnO2 batteries. Energy Environ Sci 2023;16:2464-82.
84. Li H, Yao H, Sun X, et al. Interface regulated MnO2/Mn2+ redox chemistry in aqueous Zn ion batteries. Chem Eng J 2022;446:137205.
85. Lee B, Seo HR, Lee HR, et al. Critical role of pH evolution of electrolyte in the reaction mechanism for rechargeable zinc batteries. ChemSusChem 2016;9:2948-56.
86. Wang L, Cao X, Xu L, Chen J, Zheng J. Transformed akhtenskite MnO2 from Mn3O4 as cathode for a rechargeable aqueous zinc ion battery. ACS Sustain Chem Eng 2018;6:16055-63.
87. Kim SH, Oh SM. Degradation mechanism of layered MnO2 cathodes in Zn/ZnSO4/MnO2 rechargeable cells. J Power Sources 1998;72:150-8.
88. Yang H, Zhang T, Chen D, et al. Protocol in evaluating capacity of Zn-Mn aqueous batteries: a clue of pH. Adv Mater 2023;35:e2300053.
89. Sambandam B, Mathew V, Kim S, et al. An analysis of the electrochemical mechanism of manganese oxides in aqueous zinc batteries. Chem 2022;8:924-46.
90. Ma Y, Xu M, Liu R, et al. Molecular tailoring of MnO2 by bismuth doping to achieve aqueous zinc-ion battery with capacitor-level durability. Energy Stor Mater 2022;48:212-22.
91. Wang C, Zeng Y, Xiao X, et al. γ-MnO2 nanorods/graphene composite as efficient cathode for advanced rechargeable aqueous zinc-ion battery. J Energy Chem 2020;43:182-7.
92. Zhao M, Luo Y, Zhu L, et al. Ultrathin δ-MnO2 nanosheets branched onto N-doped carbon nanotubes as binder-free cathode electrodes for aqueous zinc-ion batteries with a high areal capacity. J Alloys Compd 2022;913:165124.
93. Li L, Yang Q, Wang D, et al. Facile synthesis λ-MnO2 spinel for highly effective catalytic oxidation of benzene. Chem Eng J 2021;421:127828.
94. Zhang N, Cheng F, Liu Y, et al. Cation-deficient spinel ZnMn2O4 cathode in Zn(CF3SO3)2 electrolyte for rechargeable aqueous zn-ion battery. J Am Chem Soc 2016;138:12894-901.
95. Pam ME, Yan D, Yu J, et al. Microstructural engineering of cathode materials for advanced zinc-ion aqueous batteries. Adv Sci 2020;8:2002722.
96. Yu Z, Tetard L, Zhai L, Thomas J. Supercapacitor electrode materials: nanostructures from 0 to 3 dimensions. Energy Environ Sci 2015;8:702-30.
97. Hao J, Mou J, Zhang J, et al. Electrochemically induced spinel-layered phase transition of Mn3O4 in high performance neutral aqueous rechargeable zinc battery. Electrochim Acta 2018;259:170-8.
98. Wang Y, Li H, He P, Hosono E, Zhou H. Nano active materials for lithium-ion batteries. Nanoscale 2010;2:1294-305.
99. Song H, Liu Y, Zhang C, Liu C, Cao G. Mo-doped LiV3O8 nanorod-assembled nanosheets as a high performance cathode material for lithium ion batteries. J Mater Chem A 2015;3:3547-58.
100. Alfaruqi MH, Islam S, Gim J, et al. A high surface area tunnel-type α-MnO2 nanorod cathode by a simple solvent-free synthesis for rechargeable aqueous zinc-ion batteries. Chem Phys Lett 2016;650:64-8.
101. Nam KW, Kim H, Choi JH, Choi JW. Crystal water for high performance layered manganese oxide cathodes in aqueous rechargeable zinc batteries. Energy Environ Sci 2019;12:1999-2009.
102. Li X, Zhou Q, Yang Z, et al. Unraveling the role of nitrogen-doped carbon nanowires incorporated with MnO2 nanosheets as high performance cathode for zinc-ion batteries. Energy Environ Mater 2023;6:e12378.
103. Zhang Y, Deng S, Li Y, et al. Anchoring MnO2 on nitrogen-doped porous carbon nanosheets as flexible arrays cathodes for advanced rechargeable Zn-MnO2 batteries. Energy Stor Mater 2020;29:52-9.
104. Liu G, Huang H, Bi R, Xiao X, Ma T, Zhang L. K+ pre-intercalated manganese dioxide with enhanced Zn2+ diffusion for high rate and durable aqueous zinc-ion batteries. J Mater Chem A 2019;7:20806-12.
105. Hong S, Jin S, Deng Y, et al. Efficient scalable hydrothermal synthesis of MnO2 with controlled polymorphs and morphologies for enhanced battery cathodes. ACS Energy Lett 2023;8:1744-51.
106. Wang H, Yin K, Qin N, et al. Oxygen-deficient titanium dioxide as a functional host for lithium-sulfur batteries. J Mater Chem A 2019;7:10346-53.
107. Ren Q, Qin N, Liu B, et al. An oxygen-deficient vanadium oxide@N-doped carbon heterostructure for sodium-ion batteries: insights into the charge storage mechanism and enhanced reaction kinetics. J Mater Chem A 2020;8:3450-8.
108. Uchaker E, Zheng YZ, Li S, Candelaria SL, Hu S, Cao GZ. Better than crystalline: amorphous vanadium oxide for sodium-ion batteries. J Mater Chem A 2014;2:18208-14.
109. Ku JH, Ryu JH, Kim SH, Han OH, Oh SM. Reversible lithium storage with high mobility at structural defects in amorphous molybdenum dioxide electrode. Adv Funct Mater 2012;22:3658-64.
110. Xiao D, Lv X, Fan J, Li Q, Chen Z. Zn-based batteries for energy storage. Energy Mater 2023;3:300007.
111. Huang S, Liu L, Zheng Y, et al. Efficient sodium storage in rolled-up amorphous Si nanomembranes. Adv Mater 2018;30:e1706637.
112. Fan L, Li X, Yan B, et al. Controlled SnO2 crystallinity effectively dominating sodium storage performance. Adv Energy Mater 2016;6:1502057.
113. Cai Y, Chua R, Huang S, Ren H, Srinivasan M. Amorphous manganese dioxide with the enhanced pseudocapacitive performance for aqueous rechargeable zinc-ion battery. Chem Eng J 2020;396:125221.
114. Liang R, Fu J, Deng Y, et al. Parasitic electrodeposition in Zn-MnO2 batteries and its suppression for prolonged cyclability. Energy Stor Mater 2021;36:478-84.
115. Hu Q, Jiang X, He M, Zheng Q, Lam KH, Lin D. Core-shell nanostructured MnO2@Co9S8 arrays for high-performance supercapacitors. Electrochim Acta 2020;338:135896.
116. Li Q, Wang Y, Mo F, et al. Calendar life of Zn batteries based on Zn anode with Zn powder/current collector structure. Adv Energy Mater 2021;11:2003931.
117. Islam S, Alfaruqi MH, Song J, et al. Carbon-coated manganese dioxide nanoparticles and their enhanced electrochemical properties for zinc-ion battery applications. J Energy Chem 2017;26:815-9.
118. Wu B, Zhang G, Yan M, et al. Graphene scroll-coated α-MnO2 nanowires as high-performance cathode materials for aqueous Zn-ion battery. Small 2018;14:e1703850.
119. Gök A, Sarı B, Talu M. Synthesis and characterization of conducting substituted polyanilines. Synth Met 2004;142:41-8.
120. Benhaddad L, Gamby J, Makhloufi L, Pailleret A, Pillier F, Takenouti H. Improvement of capacitive performances of symmetric carbon/carbon supercapacitors by addition of nanostructured polypyrrole powder. J Power Sources 2016;307:297-307.
121. Lu Q, Zhou Y. Synthesis of mesoporous polythiophene/MnO2 nanocomposite and its enhanced pseudocapacitive properties. J Power Sources 2011;196:4088-94.
122. Mao J, Wu F, Shi W, et al. Preparation of polyaniline-coated composite aerogel of MnO2 and reduced graphene oxide for high-performance zinc-ion battery. Chin J Polym Sci 2020;38:514-21.
123. Bao X, Zhang Z, Zhou D. Pseudo-capacitive performance enhancement of α-MnO2 via in situ coating with polyaniline. Synth Met 2020;260:116271.
124. Zang X, Li X, Zhu M, et al. Graphene/polyaniline woven fabric composite films as flexible supercapacitor electrodes. Nanoscale 2015;7:7318-22.
125. Tantawy HR, Kengne BF, Mcilroy DN, et al. X-ray photoelectron spectroscopy analysis for the chemical impact of solvent addition rate on electromagnetic shielding effectiveness of HCl-doped polyaniline nanopowders. J Appl Phys 2015;118:175501.
126. Han J, Wang K, Liu W, et al. Rational design of nano-architecture composite hydrogel electrode towards high performance Zn-ion hybrid cell. Nanoscale 2018;10:13083-91.
127. Kamenskii MA, Volkov FS, Eliseeva SN, Holze R, Kondratiev VV. Comparative Study of PEDOT- and PEDOT:PSS Modified δ-MnO2 cathodes for aqueous zinc batteries with enhanced properties. J Electrochem Soc 2023;170:010505.
128. Li Y, Yao H, Liu X, Yang X, Yuan D. Roles of electrolyte additive in Zn chemistry. Nano Res 2023;16:9179-94.
129. Qiu N, Chen H, Yang Z, Sun S, Wang Y. Low-cost birnessite as a promising cathode for high-performance aqueous rechargeable batteries. Electrochim Acta 2018;272:154-60.
130. Zhang N, Cheng F, Liu J, et al. Rechargeable aqueous zinc-manganese dioxide batteries with high energy and power densities. Nat Commun 2017;8:405.
131. Fan W, Xiong X, Xu Y, et al. Constructing stable Zn anodes for aqueous rechargeable zinc batteries. Next Energy 2023;1:100049.
132. Bhattachar SN, Deschenes LA, Wesley JA. Solubility: it’s not just for physical chemists. Drug Discov Today 2006;11:1012-8.
133. Tie Z, Niu Z. Design strategies for high-performance aqueous Zn/organic batteries. Angew Chem Int Ed 2020;59:21293-303.
134. Tie Z, Liu L, Deng S, Zhao D, Niu Z. Proton insertion chemistry of a zinc-organic battery. Angew Chem Int Ed 2020;59:4920-4.
135. Liu S, He J, Liu D, et al. Suppressing vanadium dissolution by modulating aqueous electrolyte structure for ultralong lifespan zinc ion batteries at low current density. Energy Stor Mater 2022;49:93-101.
136. Liu N, Wu X, Yin Y, et al. Constructing the efficient ion diffusion pathway by introducing oxygen defects in Mn2O3 for high-performance aqueous zinc-ion batteries. ACS Appl Mater Interfaces 2020;12:28199-205.
137. Jin X, Song L, Dai C, et al. A flexible aqueous zinc-iodine microbattery with unprecedented energy density. Adv Mater 2022;34:e2109450.
138. Geng Y, Pan L, Peng Z, et al. Electrolyte additive engineering for aqueous Zn ion batteries. Energy Stor Mater 2022;51:733-55.
139. Chen M, Zhou W, Wang A, et al. Anti-freezing flexible aqueous Zn-MnO2 batteries working at -35 °C enabled by a borax-crosslinked polyvinyl alcohol/glycerol gel electrolyte. J Mater Chem A 2020;8:6828-41.
140. Liu X, Li X, Yang X, et al. Influence of water on gel electrolytes for zinc-ion batteries. Chem Asian J 2023;18:e202201280.
141. Yuan D, Li X, Yao H, et al. A liquid crystal ionomer-type electrolyte toward ordering-induced regulation for highly reversible zinc ion battery. Adv Sci 2023;10:e2206469.
142. Yuan D, Zhao J, Ren H, et al. Anion texturing towards dendrite-free Zn anode for aqueous rechargeable batteries. Angew Chem Int Ed 2021;60:7213-9.
143. Liu B, Yuan X, Li Y. Colossal capacity loss during calendar aging of Zn battery chemistries. ACS Energy Lett 2023;8:3820-8.
144. Zhu R, Xiong Z, Yang H, et al. Anode/cathode dual-purpose aluminum current collectors for aqueous zinc-ion batteries. Adv Funct Mater 2023;33:2211274.
Cite This Article
Export citation file: BibTeX | RIS
OAE Style
Luo C, Lei H, Xiao Y, Nie X, Li Y, Wang Q, Cai W, Dai C, Yao M, Zhang Y, Yuan D. Recent development in addressing challenges and implementing strategies for manganese dioxide cathodes in aqueous zinc ion batteries. Energy Mater 2024;4:400036. http://dx.doi.org/10.20517/energymater.2023.119
AMA Style
Luo C, Lei H, Xiao Y, Nie X, Li Y, Wang Q, Cai W, Dai C, Yao M, Zhang Y, Yuan D. Recent development in addressing challenges and implementing strategies for manganese dioxide cathodes in aqueous zinc ion batteries. Energy Materials. 2024; 4(3): 400036. http://dx.doi.org/10.20517/energymater.2023.119
Chicago/Turabian Style
Luo, Chi, Haoyun Lei, Yiyang Xiao, Xiaoxin Nie, Yuhang Li, Qian Wang, Wenlong Cai, Chunlong Dai, Meng Yao, Yun Zhang, Du Yuan. 2024. "Recent development in addressing challenges and implementing strategies for manganese dioxide cathodes in aqueous zinc ion batteries" Energy Materials. 4, no.3: 400036. http://dx.doi.org/10.20517/energymater.2023.119
ACS Style
Luo, C.; Lei H.; Xiao Y.; Nie X.; Li Y.; Wang Q.; Cai W.; Dai C.; Yao M.; Zhang Y.; Yuan D. Recent development in addressing challenges and implementing strategies for manganese dioxide cathodes in aqueous zinc ion batteries. Energy Mater. 2024, 4, 400036. http://dx.doi.org/10.20517/energymater.2023.119
About This Article
Special Issue
Copyright
Data & Comments
Data
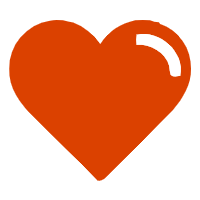

Comments
Comments must be written in English. Spam, offensive content, impersonation, and private information will not be permitted. If any comment is reported and identified as inappropriate content by OAE staff, the comment will be removed without notice. If you have any queries or need any help, please contact us at support@oaepublish.com.